Abstract
Transcranial near-infrared laser therapy (NILT) has been investigated as a novel neuroprotective treatment for acute ischemic stroke (AIS), for approximately 10 years. Two clinical trials, NeuroThera Effectiveness and Safety Trial (NEST)-1 and NEST-2, have evaluated the use of NILT to promote clinical recovery in patients with AIS. This review covers preclinical, translational, and clinical studies documented during the period 1997–2010. The primary aim of this article is to detail the development profile of NILT to treat AIS. Secondly, insight into possible mechanisms involved in light therapy will be presented. Lastly, possible new directions that should be considered to improve the efficacy profile of NILT in AIS patients will be discussed. The use of NILT was advanced to clinical trials based upon extensive translational research using multiple species. NILT, which may promote functional and behavioral recovery via a mitochondrial mechanism and by enhancing cerebral blood flow, may eventually be established as an Food and Drug Administration (FDA)-approved treatment for stroke. The NEST-3 trial, which is the pivotal trial for FDA approval, should incorporate hypotheses derived from translational studies to ensure efficacy in patients. Future NILT studies should consider administration of a thrombolytic to enhance cerebral reperfusion alongside NILT neuroprotection.
Key messages
Translational 1: Near-infrared laser therapy (NILT) has been shown to be neuroprotective with a long therapeutic window in preclinical studies in two species.
Translational 2: In embolized rabbits, NILT is safe to administer concurrently with the thrombolytic, tPA (Activase®, Alteplase®).
Translational 3: NILT improves behavioral function, enhances mitochondrial function, and increases cerebral blood flow.
Clinical 1: NILT may improve clinical function in stroke patients with National Institutes of Health Stroke Scale scores <15.
Background
The potential impact of near-infrared laser therapy (NILT) for the treatment of acute ischemic stroke (AIS) and other diseases, such as traumatic brain injury, where neuroprotection may be useful, is enormous. According to the 2009 USA stroke statistics, each year approximately 0.8 million people suffer a stroke (3 every 2 minutes, with 1 death every 3 minutes), 75% of which are first strokes and the remainder recurrent attacks (Citation1,Citation2). Annually, 18% of stroke victims die from the brain attack. AIS is the third leading cause of death and the leading cause of adult disability in the USA with an estimated cost of $68.9 billion annually. The World Health Organization (WHO) estimates that of the 15 million victims who suffer strokes annually, more than 5 million die from the brain insult, and approximately 5.5 million are permanently disabled (Citation3).
The only FDA-approved therapeutic agent that can be used effectively following an ischemic stroke is the thrombolytic, tissue plasminogen activator (tPA) (Activase®, Alteplase®), a plasminogen activator that promotes thrombolysis by activating the endogenous fibrinolytic system (Citation4–7). Even though Alteplase is not an optimal treatment, it has been shown to be effective in patients when given i.v. up to 4.5 hours after a stroke (Citation8,Citation9). Currently, tPA is FDA-approved for use within a 3-hour time-to-treatment (TT) window (Citation4,Citation8,Citation10–17). Moreover, with tPA treatment, there is significant risk of hemorrhagic transformation (HT) or intracerebral hemorrhage (ICH) in approximately 5%–8% of patients treated within 3–4.5 hours of a stroke (Citation18), and the odds ratio for mortality rate increases after 4 hours (Citation18). Since over 60% of patients do not regain full function after tPA treatment, there is considerable need to develop new neuroprotective therapies to prevent nerve cell death associated with ischemic insults.
Since NILT has not been shown to be effective in all stroke patients (Citation19,Citation20), the challenge of further developing NILT for stroke lies within the paucity of important information available on the mechanisms of action of NILT in stroke and how to most effectively deliver NILT to cortical and subcortical brain structures. The basic idea underlying the use of NILT to improve clinical function is that the treatment may realign homeostatic mechanisms to promote neuronal survival within the penumbral ‘at-risk’ area, increase the function of neuronal circuits, and then improve clinical presentation. This focused perspective article should shed some light on the utility of NILT to treat AIS, including its benefits and shortcomings.
Focus on light penetration and mechanism
It has been demonstrated that irradiation with specific wavelengths (nm) of infrared light (i.e. 808 nm), allows for light penetration of the scalp, skull, and brain tissues (Citation21–25). This type of irradiation results in a variety of photobiostimulation effects, which have been documented for both in-vitro and in-vivo conditions (Citation19,Citation22,Citation23,Citation26–31). The biological effects of NILT are wavelength-specific and are not due to thermal effects of the laser beam (Citation25,Citation32,Citation33). The original rabbit embolic stroke study by Lapchak et al. (Citation24) showed that a 10-minute irradiation of the rabbit brain at midline using high continuous wave (CW) energy (25 mW/cm2, 15 J/cm2), through the overlying shaved skin, which resulted in significant behavioral improvement, increased surface skin temperature below the probe by up to 3°C; however, the focal brain temperature directly under the laser probe was increased by 0.8–1.8°C during the NILT treatment. This was considered to be minimal heating and of no possible deleterious consequence.
Photobiostimulation increases adenosine triphosphate (ATP) formation after energy absorption inside mitochondria (Citation34) in a wavelength-specific manner (Citation25). A compound that absorbs energy in the spectral region of interest (808 nm) is known as a chromophore. There is evidence that suggests that a primary mitochondrial chromophore for photobiostimulation is cytochrome c oxidase (COX) (Citation26,Citation34–36). This enzyme complex contains two copper centers, CuA and CuB. The CuA center has a broad absorption peak around 830 nm in its oxidized form. The laser used in preclinical and clinical studies delivers energy at 808 nm, which is within this absorption peak. COX is a terminal enzyme in the cellular respiratory chain and is located in the inner mitochondrial membrane. It plays a central role in the cellular bioenergetics by delivering protons across the inner membrane and thereby driving the formation of ATP by oxidative phosphorylation. In addition to leading to increased mitochondrial ATP formation, photobiostimulation may also initiate secondary cell-signaling pathways (Citation37). The overall result is improved energy metabolism and, potentially, enhanced cell viability. This may ultimately be an be an important aspect of NILT pharmacology, because the normalization of ATP levels in cells within the ‘penumbra’ may maintain homeostatic functions of cells and prevent spreading of ischemia-induced death within the penumbra.
The proposed mechanism in ischemic tissue involving mitochondrial stimulation is based upon a recent study by Lapchak and de Taboada (Citation37) using a rabbit embolic stroke model. In the study, the authors tested the effect of varying cortical power density on ATP content in brain tissues following embolization. Moreover, the authors used CW laser delivery for low cortical power densities, and, to achieve high cortical power densities without excessively heating the scalp, skull, or brain tissues, high peak power densities were delivered to the cortical surface using pulse wave (PW) NILT. As shown in the study (Citation37), and , CW NILT (2 minutes, cortical irradiance of 7.5 mW/cm2; cortical fluence of 0.9 J/cm2) increased cortical ATP content by 41% (P > 0.05 compared to sham-treated embolized control or naive control). However, when NILT was delivered using a PW mode, PW1 (3.50 mJ per pulse @ 100 Hz; average cortical irradiance of 37.5 mW/cm2, average cortical fluence of 4.5 J/cm2) increased cortical ATP content by 157% (compared to sham-treated embolized rabbits; P < 0.01), and PW2 mode (24.5 mJ at a frequency of 100 Hz; average cortical irradiance of 262.5 mW/cm2, average cortical fluence of 31.5 J/cm2) increased cortical ATP content by 221% (compared to sham-treated embolized rabbits; P = 0.0001). Based upon the initial portion of the graph, and assuming linearity, a value of 2.86 J/cm2 was calculated for theoretical ‘normalization’ of ATP content to 100% or control levels. Currently, one of the putative mechanisms for NILT-induced behavioral improvement following stroke involves the stimulation of mitochondria to produce ATP, which then leads to preservation of tissue in the ischemic penumbra and enhanced neurorecovery. Since this may occur via glycolysis (anaerobic) or oxidative phosphorylation (aerobic) mechanisms, additional biochemical studies are required to differentiate between the two pathways.
Figure 1. Correlation between power density, behavioral improvement, and cortical ATP. Panel A provides data for a correlation between energy density and cortical ATP content measured following embolic strokes. The curve is biphasic showing a steep slope up to 4.5 J/cm2 and a shallow slope thereafter. An extrapolated value of 2.86 J/cm2 was calculated for ‘normalization’ of ATP content to 100% of control (Citation37). Panel B shows the correlation between energy density and behavioral improvement. The data show that a threshold energy density equal to or above 0.9 J/cm2 is required to produce a significant (*P < 0.05) increase in P50 values (clot dose or burden in brain measured in mg that produces abnormality in 50% of the population) following an embolic stroke (Citation24,Citation46).
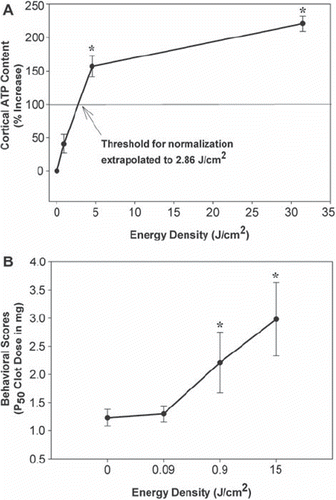
In addition to the energy hypothesis proposed above, there are also studies that show that NILT decreases apoptosis (Citation38) and probably enhances recovery of function (Citation39) via neurogenesis and the production of endogenous neurotrophic factors (Citation40). An interesting study from Uozumi and colleagues also suggests that there is a correlation between laser-induced changes in nitric oxide synthase, nitric oxide (NO), and cerebral blood flow (CBF) (Citation41). The authors studied the effect of NILT using three different power densities (0.8, 1.6, and 3.2 W/cm2) on NO production in brain tissue. Without additional laser specifications, the actual cortical fluence cannot be calculated. The study showed that near-infrared laser irradiation (1.6 W/cm2 for 15–45 minutes) significantly increased CBF, which was correlated with increased cortical NO concentration. Importantly, the study showed that the physiological effects were independent of increased temperature. While there was a small increase in temperature, similar to that previously described by Lapchak and colleagues (Citation24) for CW treatment, the increase only amounted to 0.8°C when a power density of 1.6 W/cm2 was used. Thus, the minimal heating effect is not a critical mechanism involved in CBF regulation by NILT. Therefore, currently multiple mechanisms are hypothesized to be involved in NILT photobiostimulation, including increased mitochondrial function and enhanced CBF, both of which may contribute to neuroprotection and restoration of function.
One important observation regarding the potential of PW NILT should be made at this point. The translational rabbit studies described above comparing CW to PW NILT suggest that the CW energy used in the NeuroThera Effectiveness and Safety Trial (NEST)-1 and NEST-2 clinical trials may have been insufficient to produce maximal photobiostimulation of mitochondria, thus tissue survival and overall function were not stimulated maximally. It is hypothesized that this may be achievable using a PW NILT treatment regimen.
Translational NILT studies
In this section, a comprehensive review of NILT effects in preclinical stroke models will be discussed as a foundation for further clinical trial development. documents the relationship between laser therapy use and behavioral or biochemical outcomes.
Table I. Correlation between cortical fluence and outcome following an embolic stroke.
Correlation between NILT energy density and behavioral function
As previously described (Citation24,Citation42), the rabbit small clot embolic stroke model (RSCEM) was used to determine the effects of cortical surface energy density (J/cm2) on behavioral performance. In this set of data, the authors show that there is a correlation between energy density and behavioral outcome following embolic stroke. In the studies, behavioral outcome was measured using a statistical quantal analysis method previously described (Citation43,Citation44). The method, which used a dichotomous rating scale, allows for a measure of how a large population of embolized rabbits will respond to a specific treatment. The study showed that an energy density of 0.09 J/cm2 was insufficient to produce behavioral improvement after a stroke and thus is below the threshold required for a behavioral response (). It should also be noted that an energy density of 0.09 J/cm2 is approximately 10% of the energy used in the CW NILT group in the ATP analysis studies described above, where only a small statistically insignificant increase (41%) in ATP content was measured. However, as energy density is increased to 0.9 J/cm2 and 15 J/cm2, significant behavioral improvements were measured () as well as cortical ATP levels ( and ).
Therapeutic window analysis
Since enrollment of patients in clinical trials can be somewhat difficult if the therapy has to be initiated in the hyperacute phase after a stroke (Citation45), development of treatment with relatively long therapeutic windows will ultimately be beneficial to greater numbers of patients. To determine the therapeutic window for NILT, we used the RSCEM and altered the time of NILT treatment following embolization. Initial studies showed that NILT was effective when applied 5 minutes following embolization (Citation24), but since that is clinically unrealistic, we expanded the study to include treatment initiated 1, 3, 6, 12, or 24 hours following embolization. provides information on the therapeutic window in embolized rabbits. The beneficial effect of NILT is statistically better (P < 0.05) than the control group up to 6 hours following an embolic stroke (). When measuring the effects of NILT initiated 12 hours following embolization, there was a shift in quantal analysis curves toward a positive effect (P > 0.05), but there was large variability in the group, suggesting that not all embolized rabbits responded to the treatment. This could be due to differential infarct localization in various rabbits because of the nature of the small clot embolic stroke model (Citation43), which causes heterogeneous infarct cores and large surface areas of ‘penumbra’. Unfortunately, unlike clinical trials where patients can be stratified into stroke severity, the RSCEM does not allow for that type of post-hoc analysis due to the inclusion of low numbers of ‘patients’ in each study. However, when a beneficial effect of NILT was measured, it was durable and could be measured up to 21 days after a single treatment (Citation24,Citation46).
Figure 2. NILT therapeutic window analysis in rabbits following embolic strokes. Therapeutic window for continuous wave (CW) NILT induced clinical improvement in small clot-embolized rabbits. Data are shown as clinical improvement (% Increase) as a function of NILT treatment time (initiation post embolization). The beneficial effect of NILT is statistically significant (*P < 0.05) when applied up to 6 hours following an embolic stroke (Citation24,Citation42,Citation46), but not thereafter.
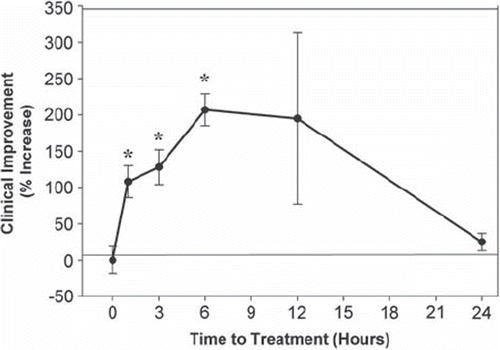
Additional pharmacology studies with CW NILT (7.5 mW/cm2) have been done by Oron and colleagues using a rodent stroke model (Citation39) produced by insertion of an intraluminal filament to occlude the middle cerebral artery. Interestingly, the authors reported that NILT was ineffective when applied 4 hours after filament placement, but was extremely effective when applied 24 hours after filament placement. In the study, the rats were permitted to survive for 28 days post stroke, and then several behavioral tests were conducted. The studies with the 24-hour treatment delay showed that there was a 32% improvement in performance on all behavioral tests by the NILT group relative to controls, and these differences were highly statistically significant. Similar findings using CW NILT (7.5 mW/cm2) and the same filament model were published by de Taboada et al. (Citation22). The reason for the obvious difference in therapeutic window between rabbits and rats is not so obvious and remains unknown, but may be related to the method of stroke or ischemia induction and model design. Clearly from the two rat studies (Citation22,Citation39), the model is designed to study long-term recovery of function as it may be related to repopulation of neuronal tracts by new cells after NILT induction. The authors (Citation39) suggest that neurogenesis may be involved in the NILT effects in rats, but with small changes in bromodeoxyuridine staining it is uncertain whether NILT induces neurogenesis to an extent sufficient to reinnervate the vast area of infarcted cortex and striatum following filament-induced cell death. Additional studies are required to study the effects of NILT on neurogenesis and synaptogenesis.
To pulse or not to pulse?
As described previously in the mechanisms section (see above), PW mode NILT was shown to be more efficacious than CW NILT at increasing ATP content in the cortex of embolized rabbits (). We also used the RSCEM to determine if PW1 NILT (300 microsecond pulses at 1 kHz, 30% Duty cycle, cortical fluence 0.9 J/cm2) or PW2 NILT (2 millisecond pulses at 100 Hz, 20% Duty cycle, cortical fluence 0.9 J/cm2) would be more effective than CW NILT (7.5 mW/cm2, cortical fluence 0.9 J/cm2, 100% Duty cycle) when a 6-hour delay of treatment was used post embolization (Citation46). With these specific settings, using a common power density of 7.5 mW/cm2, 2 minutes’ treatment time to produce irradiation times of 120, 36, and 24 seconds, respectively (Citation46), the study found that PW NILT modes produced statistically significant increases (87%–90%) in performance (P < 0.05). Even though CW NILT also increased performance, there was much variability in the response with the lower power density, and the trend did not reach statistical significance (P > 0.05). The original RSCEM studies used 10-minute NILT with a power density of 25 mW/cm2 (cortical fluence 15 J/cm2) to provide therapeutic efficacy data with a 6-hour delay (Citation24). Thus, the studies suggested that PW NILT treatment may be more beneficial to treat stroke than CW NILT.
Safety profile of NILT—preparing for a tPA combination study in patients
The goal of preclinical translational research is to accrue an extensive data portfolio so that the steering committee of the clinical trial could be advised of beneficial or possibly detrimental interactions before testing the therapies in stroke patients. Stroke patients and their families who consent to experimental therapies should be assured of the safety of the experimental treatments, as much as humanly possible. This can only be accomplished if the research community conducts appropriate experiments, in valid models, to support a clinical trial.
In this section, a rabbit large clot embolic stroke model (RLCEM), previously described by Lyden (Citation47), Lapchak et al. (Citation48), and more recently by Lapchak in a refined version of the original model (Citation49), was used to determine the safety profile of NILT and NILT in combination with tPA (Citation48). The studies were designed in order to initiate a four-arm randomized double-blind clinical trial to include NILT and the combination of NILT plus the standard of care, tPA (Citation50). For these studies, we used CW NILT (10 mW/cm2, 1.2 J/cm2), which was the NILT treatment in NEST-1 and NEST-2, and a standard maximally effective rabbit dose of tPA (Citation48,Citation51). In this translational study trial design, NILT was initiated 30 minutes following the start of tPA administration, a time when thrombolysis was near complete or complete. The study showed that CW NILT-treated embolized rabbits had a hemorrhage rate of 21.7%, whereas sham-treated embolized control rabbits had a hemorrhage rate of 17.4% (P > 0.05). Thus, NILT did not significantly affect hemorrhage rate in embolized rabbits (). Moreover, we found that NILT decreased tPA-induced hemorrhage rate by 30% compared to the tPA-treated group, which increased hemorrhage by 160% over base-line. The increase in hemorrhage rate following tPA administration is consistent with previous studies (Citation52). We also found that there was no effect of CW NILT on hemorrhage volume measured in tPA-treated rabbits, and 24-hour survival rate was unaffected (Citation48). This preclinical safety study should eventually lead the way to initiate a four-arm clinical trial with enrollment of patients to receive tPA within the current FDA-approved therapeutic window of 3 hours or possibly within the expanded 4.5-hour window that should be approved by the FDA in due course.
Additional safety studies in rodents have been documented by Ilic et al. (Citation23). The basic study design was to test the effects of increasing power density between 7.5 mW/cm2, 75 mW/cm2, and 750 mW/cm2 on histochemical and behavioral measures of neuronal damage using CW treatment. The authors found that no significant tissue damage could be detected using either light or electron microscopic damage at the two lower doses. However, at the highest dose, there was significant behavioral and histological damage. Thus, CW NILT below 75 mW/cm2 appeared to be safe and well tolerated.
NILT in AIS clinical trials
As alluded to previously, two randomized double-blind clinical trials with NILT (10 mW/cm2, delivering an estimated cortical fluence of 1.2 J/cm2) have already been completed (Citation19,Citation20), and, because of a reproducible and measurable clinical improvement in AIS patients with National Institutes of Health Stroke Scale (NIHSS) scores < 15, a third trial is currently being developed. This section will document a side-by-side comparison of the NEST clinical trial design and outcome (). In addition, presents details of the time-course of NILT development from innovation and patents between 1997 and 2002, publication of the first translational studies in 2004, and initiation of NEST-3 in 2010.
Table II. Comparison of NEST-1, NEST-2, and NEST-3 clinical trials.
Figure 3. NILT development profile to treat stroke. Complete development profile for NILT to treat AIS from concept to multiple phase III clinical trials supported by translational research studies in multiple preclinical stroke models. RSCEM = rabbit small clot embolic stroke model; RLCEM = rabbit large clot embolic stroke model. Citations are in brackets.
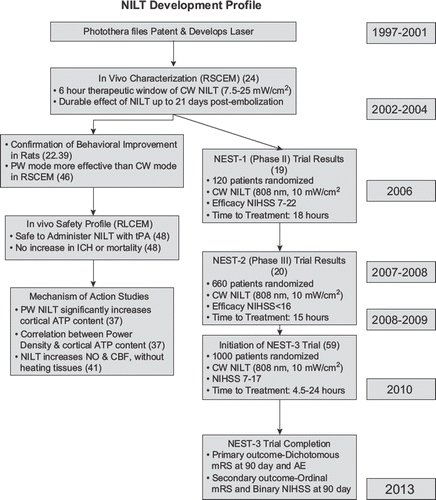
NEST-1
The phase II NEST-1 clinical trial (Citation19), which studied NILT in 120 patients, was a prospective, double-blind, randomized (2:1) study of patients 40–85 years of age treated within 24 hours of ischemic stroke onset with base-line NIHSS scores of 7–22. The primary end-points were the NIHSS and the modified Rankin Scale (mRS) commonly used in clinical trials (Citation8,Citation53–56). The specific end-point criteria used in NEST-1 were the following:
NIHSS: Three categories of values of the NIHSS score were used. The three NIHSS strata were 7 to 10, 11 to 15, and 16 to 22. Since the NIHSS scale is not an interval scale, the categories were used to reduce potential heterogeneity. NIHSS outcome was collapsed into a binary NIH outcome, where successful treatment could be measured in two ways: as a 90-day NIHSS score 0 to 1 or as a decrease in score (change) of 9 or more points from base-line to 90 days.
The mRS 90-day outcome was also measured in two ways: The first used the standard seven-category ordinal form, analyzed across the whole distribution of scores on the 0 to 6 mRS scale (full mRS), whereas the alternate used a binary mRS with scores of 0,2 considered as positive or successful and scores of 3,6 as negative or treatment failure.
Unlike the rabbit embolic stroke studies where a single application was sufficient, for human, because of the size differences, the probe was applied to 20 points on the skull for a duration of 2 min at each spot, for a power density of 10 mW/cm2 and energy density of 1.2 J/cm2. This ensured that NILT could encompass the complete cortex and allow for limited penetration of the laser light. The results of the NEST-1 and NEST-2 studies were quite promising and showed that more patients in the active treatment group had successful outcomes than did controls, as measured prospectively on the NIHSS and mRS. In an innovative landmark neuroprotection clinical trial, Lampl and colleagues (Citation19) found that NILT-treated patients showed greater improvement from base-line to 90 days (P = 0.021) than did the sham-treated group (). Logistic regression controlling for age, sex, TT, and base-line score showed that 38% of NILT patients achieved a final NIHSS score of 0–1 and improved by 9 points or greater. For the binary mRS outcome, 0,2 versus 2,6, 60% of NILT-treated patients had a positive outcome (P = 0.034–0.043). Mortality rates and serious adverse events did not differ significantly. This clinical trial, which was originally designed to be a safety study, provided positive results which were the incentive to quickly expand to the NEST-2 trials to study efficacy.
NEST-2
Thus, based upon the NEST-1 results, a larger phase III trial, NEST-2, was conducted in 660 stroke victims (Citation20). The protocol of the trial was nearly identical to NEST-1 except the primary end-point was the mRS, although NIHSS was used for additional analysis (i.e. stratification of results according to enrollment base-line). The specific mRS end-point criteria used in NEST-2 were as follows: the primary efficacy outcome measure was the dichotomous mRS scores measured 90 days after NILT with success measured (as mRS 0,2) and failure (mRS 3,6). Overall, the trial results did not reach statistical significance (P = 0.094), and this was not considered to be positive, when all patients were included. This finding was unexpected in light of NEST-1 trial results. However, post-hoc analysis indicated that only moderately affected AIS patients with NIHSS 7–15 upon enrollment achieved better performance at 90 days. This did reach statistical significance for the particular patient group (P = 0.044). Thus, using pre-specified subgroup analyses, the study found that a subgroup with NIHSS 7–15 benefited from the NILT, but patients with high base-line NIHSS scores were not improved. This may be a technical limitation of the low power density CW used in the NEST-1 and NEST-2 clinical trials and currently in use in the NEST-3 trial.
NILT depth penetration analysis
Based upon experience with the rabbit embolic stroke model and design studies for that particular model, optimal NILT penetration of the rabbit skull and brain may be achieved to a depth of 25–30 mm, essentially its complete thickness, and the NILT beam would encompass the majority of the brain if placed on the skin surface posterior to bregma (21 mm) on the midline (Citation24). However, using rats, de Taboada et al. (Citation22) have postulated there would be an estimated power density drop from 10 mW/cm2 (1.2 J/cm2) at the cortex of the rat brain to approximately 7.5 μW/cm2 (0.9 mJ/cm2) at 18 mm depth from the cortical surface. Because of the size of the rabbit brain, a similar decrease is expected. In the NEST-2 trial publication, the investigators also indicate that NILT will only penetrate the brain to approximately 20 mm depth (Citation20) using the CW design (10 mW/cm2, 1.2 J/cm2). Using a basic mathematical approach and knowing that the average skull thickness in humans is 7–10 mm (Citation57) and the distance from the skull to the brain is an additional 1–2 mm, then maximal NILT penetration would be limited to approximately 8 mm. The range of thickness of the human cortex is 1–4.5 mm (Citation58). Because of the complex pattern of sulci in the human brain, the surface area is extensive, and there will be differential NILT penetration when the probe is placed on the skin surface overlying the skull. With the manual placement of the probe at 20 pre-specified positions, there may be insufficient coverage of all structures underlying the cortex with CW NILT. Thus, in effect, NILT will not effectively reach all cortical layers and subcortical structures damaged by ischemia. This may be viewed as a limitation in the current treatment regimen design and the way in which NILT is being delivered to patients.
Because the rabbit embolic stroke model is one of great utility in the design and conduct of clinical trials to evaluate new modes of neuroprotection, information from the model showing that PW NILT may be more beneficial than CW NILT, at both improving behavior and also increasing energy production (cortical ATP content), should be considered in the design of NEST-3 clinical trial (see below). The greater effectiveness of PW NILT to increase ATP content may be due to increased penetration of photons through the brain using the PW modes, since, at the pulsed peaks, the PW modes will deposit more photons deeper into the brain. This study suggests that PW NILT may be useful to study in AIS patients in future clinical trials.
NEST-3
Currently, the NEST-3 clinical trial design reported on the National Institutes of Health web site (Citation59) is similar to the NEST-2 clinical trial (). The trial is designed to enroll 1,000 patients within 24 hours of a stroke with an NIHSS base-line of 7–17, the range where beneficial effects of NILT were observed in the NEST-1 and NEST-2 (Citation19,Citation20) clinical trials, but more specifically in the NEST-2 trial where NILT was ineffective in patients enrolled with an NIHSS base-line above 16 points. The current trial design does not include patients treated with the standard-of-care therapy, tPA, within 3–4.5 hours of a stroke, primarily because the time window chosen by the investigators (4.5–24 hours) precludes administration of tPA. Future trials should consider evaluating NILT with a thrombolytic to improve reperfusion and provide neuroprotection in a design similar to that used to test Cerovive and Erythropoietin trials (Citation60,Citation61).
Conclusions
The principal aim of this review was to directly evaluate the literature regarding the development profile of NILT in translational stroke models. Using a rabbit embolic stroke model that uses clinical rating scores as the primary end-point, which is based upon motor function components of the NIHSS for stroke in humans (Citation43), studies showed that NILT has durable efficacy when applied up to 6 hours following a stroke. Studies indicated that a threshold power density was required for behavioral improvement and for increasing mitochondrial function measured using cortical ATP content. A comparison of CW and PW NILT showed that both can effectively improve behavior in embolized rabbits (). PW NILT is superior at improving behavior following embolic strokes in rabbits, and PW NILT also produced greater increases in mitochondrial function, based upon cortical ATP content measurements. Overall, because of the translational nature of preclinical studies done to date, it is hypothesized that PW NILT may be more effective than CW NILT in the treatment of embolic stroke in patients. The hypothesis should be tested in future NILT clinical trials.
Based upon the scientific justification presented in this review and the efficacy profile of NILT in multiple preclinical translational models and in two randomized clinical trials in AIS patients, NILT should be pursued as a possible important neuroprotective treatment for stroke patients. However, given the observation that CW NILT was not effective in all patients in the NEST-2 trial, valuable information may be gleaned from translational studies to further direct NILT clinical trials so that the treatment may be beneficial to a large population of stroke patients with diverse NIHSS scores upon enrollment in the trial. Translational studies using behavior and biochemical end-points suggest that development of a pulse treatment mode may be beneficial via enhancement of mitochondrial function and possibly by allowing for deeper penetration of light into the brain. Thus, neuroprotection or recovery of function in both subcortical and cortical structures may translate into more significant improvement using the NIHSS and mRS scales.
Moreover, since tPA is currently the FDA- approved standard of care for use within a 3-hour therapeutic window (Citation50), there may be substantial value in treating stroke patients with tPA to allow for reperfusion of previously occluded or blocked cerebral vessels prior to administration of NILT. According to the NILT NEST-3 trial design (Citation59), NILT initiation is proposed to be between 4.5 and 24 hours following an ischemic stroke. The earliest treatment time, 4.5 hours, is in agreement with the newly expanded 4.5 hour therapeutic window data for tPA (Citation8,Citation9). Since a translational rabbit study has shown that NILT could be applied with tPA (Citation48) without detrimental effects, combination therapy should be considered as appropriate for a future NEST clinical trial.
Acknowledgements
Mr Luis de Taboada of Photothera Inc. is thanked for his assistance with laser specifications.
Declaration of interest: This work was supported by a U01 Translational research grant NS060685 to Paul Lapchak. Paul Lapchak is on the Scientific Advisory Boards of Photothera Inc. He has no financial interest in Photothera Inc. Photothera Inc. did not pay the author to contribute this article to the scientific literature and had no editorial influence on the scientific content of this article.
References
- Ingall T. Stroke—incidence, mortality, morbidity and risk. J Insur Med. 2004;36:143–52.
- Lloyd-Jones D, Adams R, Carnethon M, De Simone G, Ferguson TB, Flegal K, . Heart disease and stroke statistics—2009 update: a report from the American Heart Association Statistics Committee and Stroke Statistics Subcommittee. Circulation. 2009;119:480–6.
- WHO. World Health Organization Stroke Statistics. Available at: www.strokecenter.org/patients/stats.htm.(Accessed 12 October 2010).
- Lapchak PA. Development of thrombolytic therapy for stroke: a perspective. Expert Opin Investig Drugs. 2002;11: 1623–32.
- Schellinger PD, Fiebach JB, Mohr A, Ringleb PA, Jansen O, Hacke W. Thrombolytic therapy for ischemic stroke—a review. Part II—Intra-arterial thrombolysis, vertebrobasilar stroke, phase IV trials, and stroke imaging. Crit Care Med. 2001;29:1819–25.
- Schellinger PD, Fiebach JB, Mohr A, Ringleb PA, Jansen O, Hacke W. Thrombolytic therapy for ischemic stroke—a review. Part I—Intravenous thrombolysis. Crit Care Med. 2001;29:1812–8.
- Verstraete M. Newer thrombolytic agents. Ann Acad Med Singapore. 1999;28:424–33.
- Hacke W, Kaste M, Bluhmki E, Brozman M, Davalos A, Guidetti D, . Thrombolysis with alteplase 3 to 4.5 hours after acute ischemic stroke. N Engl J Med. 2008;359:1317–29.
- Lansberg MG, Bluhmki E, Thijs VN. Efficacy and safety of tissue plasminogen activator 3 to 4.5 hours after acute ischemic stroke: a metaanalysis. Stroke. 2009;40:2438–41.
- Albers GW, Bates VE, Clark WM, Bell R, Verro P, Hamilton SA. Intravenous tissue-type plasminogen activator for treatment of acute stroke: the Standard Treatment with Alteplase to Reverse Stroke (STARS) study. JAMA. 2000;283: 1145–50.
- Alberts MJ. tPA in acute ischemic stroke: United States experience and issues for the future. Neurology. 1998; 51(3 Suppl 3):S53–>5.
- Christou I, Alexandrov AV, Burgin WS, Wojner AW, Felberg RA, Malkoff M, . Timing of recanalization after tissue plasminogen activator therapy determined by transcranial doppler correlates with clinical recovery from ischemic stroke. Stroke. 2000;31:1812–6.
- Clark WM, Albers GW, Madden KP, Hamilton S. The rtPA (alteplase) 0- to 6-hour acute stroke trial, part A (A0276g): results of a double-blind, placebo-controlled, multicenter study. Thromblytic therapy in acute ischemic stroke study investigators. Stroke. 2000;31:811–6.
- Grotta JC, Alexandrov AV. tPA-associated reperfusion after acute stroke demonstrated by SPECT. Stroke. 1998;29:429–32.
- Grotta JC, Burgin WS, El-Mitwalli A, Long M, Campbell M, Morgenstern LB, . Intravenous tissue-type plasminogen activator therapy for ischemic stroke: Houston experience 1996 to 2000. Arch Neurol. 2001;58:2009–13.
- Hacke W, Brott T, Caplan L, Meier D, Fieschi C, von Kummer R, . Thrombolysis in acute ischemic stroke: controlled trials and clinical experience. Neurology. 1999;53:S3–14.
- Hacke W, Kaste M, Fieschi C, Toni D, Lesaffre E, von Kummer R, . Intravenous thrombolysis with recombinant tissue plasminogen activator for acute hemispheric stroke. The European Cooperative Acute Stroke Study (ECASS). JAMA. 1995;274:1017–25.
- Lees KR, Bluhmki E, von Kummer R, Brott TG, Toni D, Grotta JC, . Time to treatment with intravenous alteplase and outcome in stroke: an updated pooled analysis of ECASS, ATLANTIS, NINDS, and EPITHET trials. Lancet. 2010; 375:1695–703.
- Lampl Y, Zivin JA, Fisher M, Lew R, Welin L, Dahlof B, . Infrared laser therapy for ischemic stroke: a new treatment strategy: results of the NeuroThera Effectiveness and Safety Trial-1 (NEST-1). Stroke. 2007;38:1843–9.
- Zivin JA, Albers GW, Bornstein N, Chippendale T, Dahlof B, Devlin T, . Effectiveness and safety of transcranial laser therapy for acute ischemic stroke. Stroke. 2009;40:1359–64.
- Zhang Q, Ma H, Nioka S, Chance B. Study of near infrared technology for intracranial hematoma detection. J Biomed Opt. 2000;5:206–13.
- Detaboada L, Ilic S, Leichliter-Martha S, Oron U, Oron A, Streeter J. Transcranial application of low-energy laser irradiation improves neurological deficits in rats following acute stroke. Lasers Surg Med. 2006;38:70–3.
- Ilic S, Leichliter S, Streeter J, Oron A, DeTaboada L, Oron U. Effects of power densities, continuous and pulse frequencies, and number of sessions of low-level laser therapy on intact rat brain. Photomed Laser Surg. 2006;24:458–66.
- Lapchak PA, Wei J, Zivin JA. Transcranial infrared laser therapy improves clinical rating scores after embolic strokes in rabbits. Stroke. 2004;35:1985–8.
- Mochizuki-Oda N, Kataoka Y, Cui Y, Yamada H, Heya M, Awazu K. Effects of near-infra-red laser irradiation on adenosine triphosphate and adenosine diphosphate contents of rat brain tissue. Neurosci Lett. 2002;323:207–10.
- Desmet KD, Paz DA, Corry JJ, Eells JT, Wong-Riley MT, Henry MM, . Clinical and experimental applications of NIR-LED photobiomodulation. Photomed Laser Surg. 2006; 24:121–8.
- Nissan M, Rochkind S, Razon N, Bartal A. HeNe laser irradiation delivered transcutaneously: its effect on the sciatic nerve of rats. Lasers Surg Med. 1986;6:435–8.
- Byrnes KR, Waynant RW, Ilev IK, Wu X, Barna L, Smith K, . Light promotes regeneration and functional recovery and alters the immune response after spinal cord injury. Lasers Surg Med. 2005;36:171–85.
- Oron U, Yaakobi T, Oron A, Mordechovitz D, Shofti R, Hayam G, . Low-energy laser irradiation reduces formation of scar tissue after myocardial infarction in rats and dogs. Circulation. 2001;103:296–301.
- Ad N, Oron U. Impact of low level laser irradiation on infarct size in the rat following myocardial infarction. Int J Cardiol. 2001;80:109–16.
- Lapchak PA, Araujo DM. Advances in ischemic stroke treatment: neuroprotective and combination therapies. Expert Opin Emerg Drugs. 2007;12:97–112.
- Anders JJ, Borke RC, Woolery SK, Van de Merwe WP. Low power laser irradiation alters the rate of regeneration of the rat facial nerve. Lasers Surg Med. 1993;13:72–82.
- Castro-e-Silva O Jr, Zucoloto S, Marcassa LG, Marcassa J, Kurachi C, Melo CA, . Spectral response for laser enhancement in hepatic regeneration for hepatectomized rats. Lasers Surg Med. 2003;32:50–3.
- Eells JT. Therapeutic photobiomodulation for methanol- induced retinal toxicity. Proc Natl Acad Sci U S A. 2003; 100:3439–44.
- Karu T. Mitochondrial mechanisms of photobiomodulation in context of new data about multiple roles of ATP. Photomed Laser Surg. 2010;28:159–60.
- Drochioiu G. Laser-induced ATP formation: mechanism and consequences. Photomed Laser Surg. 2010;28:573–4.
- Lapchak PA, De Taboada L. Transcranial near infrared laser treatment (NILT) increases cortical adenosine-5’-triphosphate (ATP) content following embolic strokes in rabbits. Brain Res. 2010;1306:100–5.
- Liang HL, Whelan HT, Eells JT, Wong-Riley MT. Near- infrared light via light-emitting diode treatment is therapeutic against rotenone- and 1-methyl-4-phenylpyridinium ion-induced neurotoxicity. Neuroscience. 2008;153:963–74.
- Oron A, Oron U, Chen J, Eilam A, Zhang C, Sadeh M, . Low-level laser therapy applied transcranially to rats after induction of stroke significantly reduces long-term neurological deficits. Stroke. 2006;37:2620–4.
- Leung MC, Lo SC, Siu FK, So KF. Treatment of experimentally induced transient cerebral ischemia with low energy laser inhibits nitric oxide synthase activity and up-regulates the expression of transforming growth factor-beta 1. Lasers Surg Med. 2002;31:283–8.
- Uozumi Y, Nawashiro H, Sato S, Kawauchi S, Shima K, Kikuchi M. Targeted increase in cerebral blood flow by transcranial near-infrared laser irradiation. Lasers Surg Med. 2010;42:566–76.
- Lapchak PA, Streeter J, De Taboada L. Transcranial near infrared laser therapy (NILT) to treat acute ischemic stroke: a review of efficacy, safety and possible mechanism of action derived from rabbit embolic stroke studies. Proceedings SPIE [Review]. 2010;75520R:7552–31.
- Lapchak PA. Translational stroke research using a rabbit embolic stroke model: a correlative analysis hypothesis for novel therapy development. Transl Stroke Res. 2010;1:96–107.
- Waud DR. On biological assays involving quantal responses. J Pharmacol Exp Ther. 1972;183:577–607.
- Ferguson KN, Kidwell CS, Starkman S, Saver JL. Hyperacute treatment initiation in neuroprotective agent stroke trials. J Stroke Cerebrovasc Dis. 2004;13:109–12.
- Lapchak PA, Salgado KF, Chao CH, Zivin JA. Transcranial near-infrared light therapy improves motor function following embolic strokes in rabbits: An extended therapeutic window study using continuous and pulse frequency delivery modes. Neuroscience. 2007;148:907–14.
- Lyden PD, Zivin JA, Soll M, Sitzer M, Rothrock JF, Alksne J. Intracerebral hemorrhage after experimental embolic infarction. Anticoagulation. Arch Neurol. 1987;44:848–50.
- Lapchak PA, Han MK, Salgado KF, Streeter J, Zivin JA. Safety profile of transcranial near-infrared laser therapy administered in combination with thrombolytic therapy to embolized rabbits. Stroke. 2008;39:3073–8.
- Lapchak PA. A new embolus injection method to evaluate intracerebral hemorrhage in New Zealand white rabbits. Brain Res. 2010;1349C:129–36.
- Tissue plasminogen activator for acute ischemic stroke. The National Institute of Neurological Disorders and Stroke rt-PA Stroke Study Group. N Engl J Med. 1995;333:1581–7.
- Lapchak PA, Araujo DM, Zivin JA. Comparison of tenecteplase with alteplase on clinical rating scores following small clot embolic strokes in rabbits. Exp Neurol. 2004; 185:154–9.
- Lapchak PA, Chapman DF, Zivin JA. Metalloproteinase inhibition reduces thrombolytic (tissue plasminogen activator)-induced hemorrhage after thromboembolic stroke. Stroke. 2000;31:3034–40.
- Lyden P, Lu M, Jackson C, Marler J, Kothari R, Brott T, . Underlying structure of the National Institutes of Health Stroke Scale: results of a factor analysis. NINDS tPA Stroke Trial Investigators. Stroke. 1999;30:2347–54.
- Bluhmki E, Chamorro A, Davalos A, Machnig T, Sauce C, Wahlgren N, . Stroke treatment with alteplase given 3.0–4.5 h after onset of acute ischaemic stroke (ECASS III): additional outcomes and subgroup analysis of a randomised controlled trial. Lancet Neurol. 2009;8:1095–102.
- Saver JL, Gornbein J, Grotta J, Liebeskind D, Lutsep H, Schwamm L, . Number needed to treat to benefit and to harm for intravenous tissue plasminogen activator therapy in the 3- to 4.5-hour window. Joint outcome table analysis of the ECASS 3 Trial. Stroke. 2009;40:2433–7.
- Saver JL, Gornbein J. Treatment effects for which shift or binary analyses are advantageous in acute stroke trials. Neurology. 2009;72:1310–5.
- Lynnerup N, Astrup JG, Sejrsen B. Thickness of the human cranial diploe in relation to age, sex and general body build. Head Face Med. 2005;1:13.
- Fischl B, Dale AM. Measuring the thickness of the human cerebral cortex from magnetic resonance images. Proc Natl Acad Sci U S A. 2000;97:11050–5.
- NEST-3. Available at: http://clinicaltrials.gov/ct2/show/NCT01120301?term=NEST&rank=2 (accessed 12 October 2010)
- Ehrenreich H, Weissenborn K, Prange H, Schneider D, Weimar C, Wartenberg K, . Recombinant human erythropoietin in the treatment of acute ischemic stroke. Stroke. 2009;40:e647–56.
- Shuaib A, Lees KR, Lyden P, Grotta J, Davalos A, Davis SM, . NXY-059 for the treatment of acute ischemic stroke. N Engl J Med. 2007;357:562–71.