Abstract
Glucosyltransferases (Gtrs) and O-acetyltransferase (Oac) are integral membrane proteins embedded within the cytoplasmic membrane of Shigella flexneri. Gtrs and Oac are responsible for unidirectional host serotype conversion by altering the epitopic properties of the bacterial surface lipopolysaccharide (LPS) O-antigen. In this study, we present the membrane topology of a recently recognized Gtr, GtrIc, which is known to mediate S. flenxeri serotype switching from 1a to 1c. The GtrIc topology is shown to deviate from those typically seen in S. flexneri Gtrs. GtrIc has 11 hydrophilic loops, 10 transmembrane helices, a double intramembrane dipping loop 5, and a cytoplasmic N- and C-terminus. Along with a unique membrane topology, the identification of non-critical Gtr-conserved peptide motifs within large periplasmic loops (N-terminal D/ExD/E and C-terminal KK), which have previously been proven essential for the activity of other Gtrs, challenge current opinions of a similar mechanism for enzyme function between members of the S. flexneri Gtr family.
Introduction
Shigella flexneri is the primary causative agent of endemic bacillary dysentery or shigellosis worldwide, especially in developing countries where occurrences of lethal infections are most prominent [Citation1,Citation2]. There are 15 known serotypes of S. flexneri. These are categorized according to the epitopic properties of their bacterial surface LPS and can be detected biochemically or genetically [Citation3]. This intraspecies serodiversity hinders the development of a world-first protective vaccine against S. flexneri-mediated bowel infections [Citation4]. Thirteen of the serotypes (excluding 6 and 6a) express a common serotype Y LPS backbone [Citation5]. The basic S. flexneri LPS can undergo significant immunogenic changes by modifying the structure of its constituent O-antigen molecules. Gtr and Oac enzymes are principally responsible for this phenomenon, which in turn allows for serotype conversion within the species. Gtrs and Oac respectively mediate the attachment of glucosyl and O-acetyl groups to nascent O-antigen polysaccharide. Gtrs often operate in tandem sets of three: GtrA, GtrB and Gtr[type], which are together genetically encoded by a single operon, in contrast to Oac, which is encoded by an independent open-reading frame (ORF). For S. flexneri serotypes harbouring glucosylated O-antigens, GtrA and GtrB are found highly conserved and are interchangeable among strains. Gtr[type] however, is unique to each serotype and directs serotype-specific modification of the O-antigen. The Gtr-mediated mechanisms for O-antigen glucosylation remain largely unclear though it is believed to take place in the bacterial periplasm where bactoprenol glucose substrate is accessible [Citation6–8]. Stagg et al. [Citation9] recently identified the gtrIc gene encoding for the Gtr[type] that drives serotype conversion from 1a to 1c. It is thought that the GtrIc protein product (59.9 kDa; 526 residues) adds a second glucosyl group to the 1a O-antigen glucosyl side chain resulting in a unique GlcNac disaccharide attachment which is only seen in serotype 1c and not in other serotypes. Consequently, the GtrIc capacity for O-antigen modification is unlike those of other Gtr[type] members as it is strictly dependent on GtrI activity. The distinct 1c serotype, prevalent in East Asian/Pacific regions, was first identified in the 1980s but only now are we beginning to unravel the biomechanisms behind its serological emergence and appreciate it as a genuine, rather than a provisional S. flexneri serotype [Citation10–13].
In this study, we analyze the membrane topology of the novel GtrIc. Previous reports on S. flexneri GtrII and GtrV topologies have suggested a structural similarity between the Gtrs [Citation14,Citation15]. Members of this protein family commonly have nine transmembrane helices, 10 hydrophilic loops, a re-entrant loop 5, a cytoplasmic N-terminus and a periplasmic C-terminus. Using predictive software and the widely-used pho-lac dual reporter, originally developed by Alexeyev and Winkler [Citation16], we show that GtrIc possesses a unique Gtr secondary structure, non-conforming with the previously seen topologies of GtrII and GtrV. GtrIc topology studies also revealed three large periplasmic domains which have been implicated in Gtr[type] activity because of their considerable size to accommodate for substrate binding as well as an associated periplasmic localization with donor substrate. Two conserved Gtr sequence moieties were identified within these large periplasmic loops, including an N-terminal D/ExD/E motif (where x annotates any residue) and C-terminal KK (dilysine) sites. Earlier reports have established the functional significance of such moieties in other Gtrs, hinting at a common enzymatic mode of action for members of the Gtr[type] family [Citation15,Citation17]. By point-mutational analysis, our studies show the conserved D/ExD/E and KK motifs to be non-essential in GtrIc function. These are first signs of an alternative Gtr mode of catalysis for S. flexneri O-antigen glucosylation.
Materials and methods
In silico topology studies
Seven web-based topology predicting applets were used to predict the membrane topology of GtrIc. As input for all programs, the GtrIc protein sequence was analyzed on the basis of residue hydrophobicity indices and charge distribution. The programs utilized in this study include DAS [http://www.sbc.su.se/∼miklos/DAS/] [Citation18], HMMTOP [http://www.enzim.hu/hmmtop/] [Citation19], PHDhtm [http://npsa-pbil.ibcp.fr/] [Citation20], SOSUI [http://bp.nuap.nagoya-u.ac.jp/sosui/] [Citation21], TMHMM [http://www.cbs.dtu.dk/services/TMHMM-2.0/] [Citation22], TMpred [http://www.ch.embnet.org/software/TMPRED_form.html] [Citation23], as well as TopPred [http://bioweb.pasteur.fr/seqanal/interfaces/toppred.html] [Citation24].
Molecular cloning and DNA techniques
Standard procedures for bacterial cultivation, recombinant DNA cloning and bacterial transformations by electroporation followed those set by Sambrook and Russell [Citation25]. Plasmid constructs created in this study were derived from the high copy-number cloning vector pBCSK+ from Stratagene (see Table SI in Supplementary material, online version only). PCR was performed using oligonucleotides supplied by Sigma-Aldrich (see Table SII in Supplementary material, online version only) and Pfu Ultra II (Strategene). Restriction endonucleases were supplied by Fermentas and New England Biolabs. Ligations were performed using T4 DNA ligase (Promega). DNA sequencing was performed at the Biomolecular Resource Facility, John Curtin School of Medical Research, Australian National University. The QuikChange Site-Directed Mutagenesis Kit (Stratagene) was used to introduce point mutations within gtrIc before mutant DNA was introduced into XL1-Blue MRF' Escherichia coli for cloning and repair of nicked DNA.
Topology determination via a genetic approach
The pho-lac topology dual reporter was fused in-frame at various sites of the gtrIc ORF by three distinct fusion approaches: (i) The high-throughput nested deletion system [Citation26], (ii) PCR, and (iii) the sandwich method [Citation27]. These methods have been used in the past for topology determination of other S. flexneri O-antigen modifying proteins [Citation7,Citation14,Citation15,Citation28]. The Erase-a-base Kit (Promega) was used to obtain a series of nested deletion gtrIc/pho-lac fusions. A template plasmid pNV1734 was first constructed to allow ExoIII 3′ to 5′ degradation of gtrIc from its downstream terminus (see Figure S1 in Supplementary material, online version only). pNV1734 was cloned by PCR amplification of gtrIc from pNV1650 [Citation9] using primers GtrIcF2- NheI (5′-CAGTGCTAGCAGGGATTCAACTGATTGG-3′) and GtrIcR2-BamHI (5′-TGAGGATCCGACAGGATCAATCACCGC-3′) and then subsequent replacement of the oac gene in pNV1492 [Citation28] with the gtrIc amplicon flanked by NheI and BamHI cloning sites. pNV1734 was also used as template to construct PCR-mediated gtrIc/pho-lac fusions. Sandwich gtrIc/pho-lac/gtrIc constructs were achieved by inserting a pho-lac/EcoICRI/NarI double digest from pMA632 [Citation16] into a gtrIc HpaI site that was introduced through site-directed mutagenesis using pNV1650 as template.
Plasmid constructs were introduced into JM109 E. coli for pho-lac expression. E. coli strains containing the different fusion products are listed in . The pho-lac dual reporter encodes an alkaline phosphatase (PhoA)/β-galactosidase α-subunit (LacZα) chimeral protein. Use of this system for tagging various gtrIc codons enabled the simultaneous analysis of PhoA and LacZ activities, consequently avoiding the need to construct independent enzyme fusions. Provided PhoA functions only in the periplasm and LacZ activity (via. α-complementation) is restricted to the cytoplasm, the subcellular localization and displacement of tagged GtrIc residues was determined relative to the Gram-negative cytoplasmic membrane.
Table I. Dual reporter activity for fusions within GtrIc.
PhoA and LacZ activities were initially detected in bacterial hosts by colouration on dual indicator (DI) plates containing their respective chromogenic substrates 5-bromo-4-chloro-3-inolyl phosphate (X-phos) and 6-chloro-3-inolyl-β-D-galactoside (Red-gal) as outlined by Alexeyev and Winkler [Citation16]. Colourless colony growth indicated absent or non-informative pho-lac fusions, for instance, out-of-frame fusions. Blue chromogenic release within bacterial clones grown in DI plates indicated PhoA activity and its hydrolysis of X-phos within the periplasm of cells. Alternatively, red coloured colonies on DI plates suggested LacZ-mediated hydrolysis of Red-gal in the bacterial cytoplasm and subsequent release of red chromogen. To correct for variable gene expression or protein synthesis of fusion products, we calculated normalized activity ratios (NARs) for the comparative analysis of PhoA and LacZ reporter activities as described by Manoil [Citation29] and Miller [Citation30], respectively. NARs represent a quantitative comparison of accessibility of reporter enzymes to bind and mediate hydrolysis of their respective substrates. NARs were obtained after calculating the relative maximum and minimum PhoA and LacZ activities seen in each distinct fusion group (C-terminal truncation and sandwich):
Functional analysis of GtrIc
Serotype conversion assays are a common practice for the detection of S. flexneri O-antigen modifying Gtr activity [Citation15,Citation17,Citation28]. In this study, a S. flexneri serotype 1a strain, SFL1416 (NCTC #3, National Collection of Type Cultures), was used for GtrIc functional testing by host serotype conversion from 1a to 1c. All S. flexneri strains used in functional assays are listed in Supplementary Table SI (in Supplementary material, online version only). Serotype 1c-specific MASF1c monoclonal antibody [Citation31] diluted 1:500 was used to profile bacterial LPS. For colony immunoblots, bacterial cultures were grown to log phase and standardized to A600 0.8–1.0 before transfer onto nitrocellulose membranes (Micron Separations) over LB agar and grown at 37°C for 2 h. Colonies were then fixed, washed and immunoblotted as previously described by Stagg et al. [Citation9]. Anti-mouse IgM peroxidase conjugate (Sigma Aldrich) was used as secondary antibody diluted 1:800. Functional studies were also carried out by slide agglutination as outlined by Stagg et al. [Citation9]. Bacteria were grown to log phase at 37°C until an A600 0.8–1.0 was reached and were gently mixed with monoclonal antibody (MASF1c) on a glass slide.
Results and discussion
GtrIc topology
A total of seven topology predicting programs were used to model the secondary structure of GtrIc. A consensus of five readouts (DAS, HMMTOP, PHDhtm, TMHMM, and TMpred) indicated that GtrIc was composed of at least 12 transmembrane α-helices and with a doublet signal at peak 6, suggested a possible re-entrant loop midway along the protein sequence (). A cytoplasmic N-terminus was detected in four programs (HMMTOP, PHDhtm, TMHMM, and TMpred) on the basis of von Heijne's “Positive-inside Rule” which states that for prokaryotic polytopic membrane proteins like GtrIc, positively charged amino acid residues preferentially localise within the cytoplasm as opposed to the periplasm [Citation32]. In silico predictions formulated the hypothetical GtrIc topology model that was verified experimentally using the pho-lac system (see Figure S2 in Supplementary material, online version only).
Figure 1. DAS predictions for potential presence of membrane spanning segments within GtrIc. Twelve transmembrane α-helices were detected at the loose cut-off (dotted red line). Peak signals are annotated according to these measurements. At the strict cut-off (solid blue line), 13 transmembrane α-helices were identified. DAS is used to represent the consensus output, with consistent plots also observed in HMMTOP, PHDhtm, TMHMM, and TMpred. This Figure is reproduced in colour in the online version of Molecular Membrane Biology.
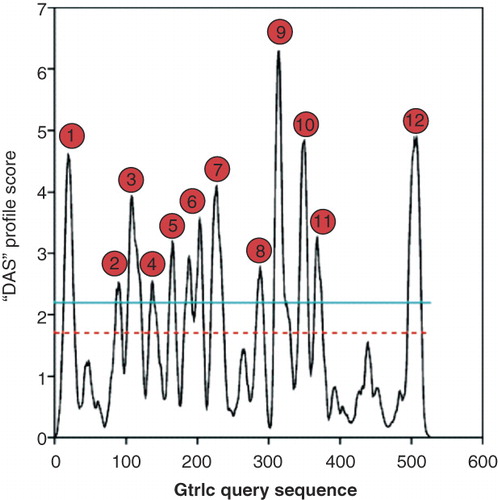
Figure 2. Determination of the GtrIc membrane topology. (a) Various strategies were used to construct pho-lac fusions at different codons of gtrIc. Replacement of the C-terminus of full length gtrIc by PCR (top); random truncation fusions obtained by nested deletion (middle); pho-lac inserted in-frame into gtrIc sequence as a sandwich fusion (bottom). (b) Experimentally verified topology model of GtrIc resolved through computerised modelling and corrected by scanning with pho-lac fusions. Truncation gtrIc/pho-lac fusions (spots) and sandwich gtrIc/pho-lac/gtrIc fusions (encapsulated spots) are coloured according to DI plate observations with their respective residue position. The location of D/ExD/E and KK motifs copies are also provided (green boxes). A larger periplasmic loop 2 was detected relative to the consensus model, along with a double membrane dipping loop centred between the N- and C- termini and ten transmembrane α-helices. This Figure is reproduced in colour in the online version of Molecular Membrane Biology.
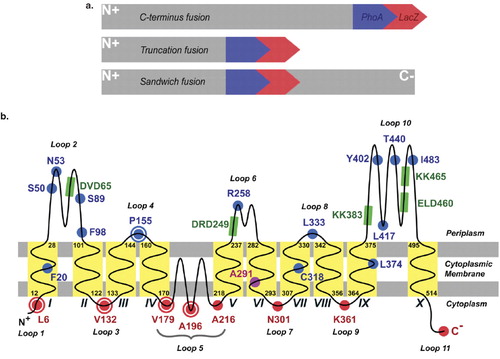
Twelve unique gtrIc/pho-lac fusions were randomly generated by nested deletion (F20, N53, S89, F98, R258, A291, C318, L374, Y402, L417, T440, and I482). Bias towards putative large periplasmic loops was observed when using the nested deletion approach, which yielded no cytoplasmic fusions. Therefore, six PCR fusions were constructed (S50, A216, N301, L333, K361, and C-terminus) for the specific targeting of protein domains of interest. According to DI screens in combination with PhoA and LacZ activity values (), all but one of these C-terminal truncation fusions were in gross agreement with the computerized working model. An ExoIII-mediated fusion at F98, residing in cytoplasmic consensus loop 3, showed blue DI colouration. Contrary to what was predicted by software, this indicated a periplasmic position. Its 1:1 NAR did not offer strong evidence for high PhoA activity since NARs greater than 3:1 or lower than 1:3 set the threshold for reliable pho-lac fusions [Citation16]. Similarly, the R258, A291 and L417 fusions also displayed low contrast in their NARs. Though DI colouration was clearly observed in the latter three fusions, it is possible that their obscure NARs were caused by steric interference of the PhoA and LacZ enzymes imposed by upstream GtrIc tertiary architecture. Membrane localization of the point of fusion (as predicted for A291) could also deny dual reporter access to either periplasmic or cytoplasmic substrates. It has been reported that purple fusions are more likely to localize within hydrophobic transmembrane segments [Citation14–16], though this is not an exclusive rule as seen in the present study with fusions F20, C318 and L374. To verify the subcellular localization of these NARs, adjacent fusions were used as topological references.
The existence of consensus helix 2 and 4 was not strongly evident in the hydrophobicity plots (). A reported blue F98 fusion indicated helix 2 was better suited as a non-transmembrane element, whereas sandwich fusions at V132 and P155 verified the position of helix 4. Consensus periplasmic loop 2 was thus made larger to accommodate for helix 2 and loop 3. This allowed for the relocation of F98 into the periplasm. Consequently, to prevent any unsubstantiated inversion of downstream topology, consensus helix 6 was reconstituted into a re-entrant loop. Hydrophobicity plots hinted for the presence of a Gtr-conserved re-entrant in the lateral center of GtrIc with a doublet signal at peak 6 (). However, sandwich gtrIc/pho-lac/gtrIc fusions were used to resolve this region and complete the topology of GtrIc by specifically targeting N-terminal sequences. Sandwich fusions offer greater confidence in their capacity to report topology [Citation14,Citation16,Citation27]. Unlike N-terminal truncation fusions, they avoid extensive degradation of native protein sequences ().
Sandwich fusions V179 and A196 and truncation fusion A216 were designed to target hydrophilic regions of the putative re-entrant loop. With all three fusions reporting red DI colouration and high LacZ NARs, a re-entrant loop that would completely traverse the cytoplasmic membrane twice was unlikely. Though such structures are seen in other S. flexneri Gtrs [Citation14,Citation15], our data suggested that GtrIc possessed either a large amphipathic cytoplasmic loop 5 or a double intramembrane dipping region at this position. It is widely understood that coupling of S. flexneri GtrA and GtrB is responsible for the translocation of cytoplasmic glucosyl groups across the plasma membrane and into the periplasm where they are used as substrate for Gtr[type]-mediated O-antigen glucosylation [Citation6–8]. Functionally active large cytoplasmic loops within Gtr[type] are counter-intuitive to this mechanism, as it would suggest redundancy in GtrA and GtrB cytoplasmic activities. With strong evidence from hydrophobicity plots and the lack of need for a large functional cytoplasmic loop, it was therefore deduced that loop 5 of GtrIc formed a double membrane dipping region. These membrane dipping loops are defined as protein segments that do not completely penetrate a bilipid layer but briefly enter and leave the same membrane face. They are often too difficult to be detected by computerized sequence analysis [Citation33,Citation34]. In prokaryotic multimeric aquaporins and ion channels, these loops are known to form membrane pores and act as filters to selectively translocate molecules across a membrane interface while preventing proton conductivity [Citation35,Citation36]. Because S. flexneri Gtrs have no known functions as membrane pores, we propose that the double membrane dipping region of GtrIc has instead a structural contribution. This attribute of membrane dipping loops is also noted in light-harvesting photosystems of cyanobacteria where, by deletion analysis, they are believed to be strictly structural elements and have minimal influence on catalytic potential [Citation37,Citation38]. In GtrII and GtrV, it is thought that a re-entrant loop is required for protein structural flexibility upon substrate binding [Citation14,Citation15]. Indeed, this may also hold true for the unique double membrane dipping region of GtrIc.
depicts the finalized GtrIc transmembrane topology. We have shown that GtrIc consists of 10 transmembrane helices, eleven hydrophilic loops, a double intramembrane dipping segment and a cytoplasmic N- and C-terminus. The final GtrIc model differed from the original consensus model (see Figure S2 in Supplementary material, online version only), indicating that computational methods for topological modeling are not sufficient alone and experimental verification is of paramount importance to produce a truthful topology map.
Compared with its Gtr[type] members, we have shown that GtrIc possesses an extra C-terminal membrane spanning domain and hydrophilic loop. These structural features are also observed in the predicted GtrIV topology [Citation15] but not in any other experimentally verified Gtr[type] members. The functional role of GtrIc helix X and loop 11 at the C-terminus remains elusive but its presence is in agreement with the protein length of GtrIc (and GtrIV) being comparably longer than other Gtr sequences. The overall structural divergence seen in GtrIc may account for its capacity to catalyze a unique Type Ic modification of the S. flexneri O-antigen.
Conserved Gtr motifs in GtrIc
Gtr[type] proteins have been widely accepted as determinants for serotype-specific glucosylation of the S. flexneri O-antigen yet their mechanism of glucosylation is still unsolved [Citation5]. Although each serotype harbours its own unique Gtr[type], past studies on GtrII and GtrV have revealed that shared functional elements exist within the Gtr[type] family [Citation15,Citation17]. These commonalities are represented as large N- and C-terminal periplasmic loops. Because of their extensive size relative to other adjacent hydrophilic loops, it is assume that these large periplasmic loops either possess catalytic sites for O-antigen modification or interact with GtrA, GtrB and/or other unidentified factors necessary for glucosylation. Functionally conserved peptide motifs also reside within these large periplasmic loops which further argue for their catalytic role. For instance, the N-terminal loop 2 D/ExD/E motif is critical for GtrII and GtrV function, and the C-terminal KK motif has been proved essential for GtrII activity [Citation15,Citation17]. Substitution of a single residue within these conserved moieties is known to cause functional ablation of Gtr[type]-mediated serotype conversion [Citation15,Citation17].
With limited sequence homology shared between Gtr[type] members and their variable protein size, identification of conserved moieties within GtrIc was achieved through manual sequence alignments (). Comparative sequence analyses revealed the Gtr-conserved loop 2 D/ExD/E motif (DVD65) along with two loop 10 KK motif copies (KK383 and KK465). The topological positions of these motifs are portrayed in and confirm their periplasmic compartment.
Figure 3. Alignment of Gtr[type] periplasmic sequences. The topologies of GtrII and GtrV were experimentally elucidated in previous studies [Citation14,Citation15]. Identification of loop 2 and large C-terminal loop sequences in GtrI, GtrIV and GtrX was based on preliminary analyses by Lehane et al. [Citation15] by using predictive software and sequence homologies between GtrI and GtrX with GtrII and GtrV, respectively. (a) The large N-terminal loop 2 of all known Gtr[type] members shows a conserved D/ExD/E motif. (b) Conserved KK (dilysine) motifs are seen in the large C-terminal loops of all Gtr[type] proteins except for GtrIV. Two KK motif copies are detected in the same loop of GtrI, GtrIc and GtrV. This Figure is reproduced in colour in the online version of Molecular Membrane Biology.
![Figure 3. Alignment of Gtr[type] periplasmic sequences. The topologies of GtrII and GtrV were experimentally elucidated in previous studies [Citation14,Citation15]. Identification of loop 2 and large C-terminal loop sequences in GtrI, GtrIV and GtrX was based on preliminary analyses by Lehane et al. [Citation15] by using predictive software and sequence homologies between GtrI and GtrX with GtrII and GtrV, respectively. (a) The large N-terminal loop 2 of all known Gtr[type] members shows a conserved D/ExD/E motif. (b) Conserved KK (dilysine) motifs are seen in the large C-terminal loops of all Gtr[type] proteins except for GtrIV. Two KK motif copies are detected in the same loop of GtrI, GtrIc and GtrV. This Figure is reproduced in colour in the online version of Molecular Membrane Biology.](/cms/asset/9f2bf423-fd39-4dc1-b203-f0fcf686efa1/imbc_a_455689_f0003_b.jpg)
The D/ExD/E motif is found conserved in many prokaryotic and eukaryotic N-terminal glucosyltransferase sequences, where it is involved in manganese coordination during co-substrate binding [Citation39–41]. In S. flexneri, it has been proposed that the loop 2 D/ExD/E motif interacts directly with the O-antigen through the deprotonation of a nucleophilic hydroxyl group of a specific acceptor sugar residue on the O-antigen polymer [Citation15,Citation17]. Conversely, the function of the C-terminal dilysine motif in S. flexneri Gtrs is still unclear. Lehane et al. [Citation15] argued that the KK motif in loop 10 of GtrII may facilitate contact with other Gtr partners based on evidence from eukaryotic membrane proteins where dilysine moieties are known to mediate protein-protein interactions essential for retrograde trafficking to the endoplasmic reticulum [Citation42,Citation43]. Thus far, there has been little focus placed on protein interactions involving S. flexneri O-antigen modifying Gtrs, despite functional complementation of Gtr[type] by co-transcribed GtrA and GtrB activities.
To determine if the GtrIc loop 2 D/ExD/E and loop 10 KK motifs were functionally critical for O-antigen modification, we introduced single missense point mutations into resident codons by site-directed mutagenesis before assaying for the retention of enzymatic function in a serotype 1a background (SFL1416). Copies of the loop 2 D/ExD/E motif were also identified in periplasmic loops 6 and 10 (DRD249 and ELD460, respectively) (). The D65 codon of the loop 2 D/ExD/E motif was substituted with alanine by site-directed mutagenesis. A single lysine from the two loop 10 dilysine sites was also mutated into alanine. This was done individually in both KK motifs and in combination. Functional analyses of GtrIc mutants, achieved through colony immunoblots and slide agglutination using MASF1c antibody, demonstrated that all introduced mutations were tolerated ().
Figure 4. Functional analysis of GtrIc mutants. Transformed SFL1416 serotype 1a harbouring mutated GtrIc constructs were assayed for host 1a to 1c serotype conversion. GtrIc point mutations are annotated in parentheses with their SFL1416-derived host. Comparisons of mutant strains can be made with the SFL1961 containing empty pBCSK+ vector and with SFL1905 containing wild-type gtrIc in pNV1650. (a) Colony immunoblots showing detection of S. flexneri serotype 1c LPS in all mutant strains. (b) Slide agglutinations complemented colony immunoblot readings, showing GtrIc activity in all mutants. Binary scoring was used for positive and negative agglutination tests.
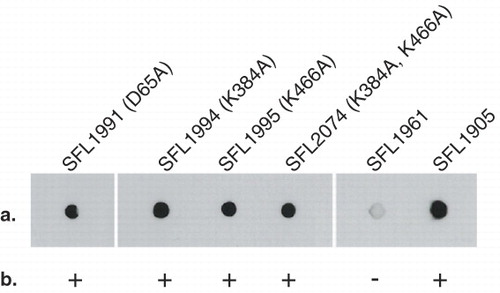
Maintenance of GtrIc activity with a D65A mutation in SFL1991 demonstrated that the N-terminal DVD65 motif is dispensable for protein function. This is unseen in other S. flenxeri Gtr[type] members. Though it remains possible that downstream motif copies are compensating for loss of GtrIc function in SFL1991, strict dependence of the conserved loop 2 moiety was not observed. Similarly, strains containing GtrIc loop 10 KK motif mutations also showed protein activity when both KK sites were individually targeted (SFL1994 and SFL1995) or simultaneously mutated (SFL2074). Because both KK copies colocalized in loop 10, we conclude that GtrIc does not require C-terminal dilysine sites for Type 1c O-antigen modification.
With the absence of a functionally critical N-terminal D/ExD/E motif and C-terminal KK sites, the mechanism in which GtrIc mediates O-antigen glucosylation stands in opposition to the conserved mode of action of other S. flexneri Gtr[type] proteins. Taken together with the unique GtrIc membrane topology highlighted in this study, our findings are consistent with proposals that the gtrIc operon was only recently, from an evolutionary perspective, introduced into the serotype 1a genome from a novel bacteriophage external to the S. flexneri species [Citation9].
The overall structural and functional distinction in GtrIc is, to date, the first of its kind proven experimentally within the Gtr family of related proteins. The full extent to which GtrIc differs from its Gtr[type] counterparts remains unknown. Understanding of the biomechanisms behind S. flexneri serotype switching and identifying variability in these processes, as seen in GtrIc from the distinct 1c strain, may offer crucial insight for the development of a multivalent and cross-reactive vaccine against shigellosis causing strains.
Supplementary Material
Download PDF (118.4 KB)Acknowledgements
We thank Herbert Winkler for providing pho-lac constructs and Nils Carlin for donating MASF1c antibody.
Declaration of interest: The authors report no conflicts of interest. The authors alone are responsible for the content and writing of the paper.
References
- Kotloff KL, Winickoff JP, Ivanoff B, Clemens JD, Swerdlow DL, Sansonetti PJ, 1999. Global burden of Shigella infections: Implications for vaccine development and implementation of control strategies. Bull World Health Organ 77(8):651–666.
- Ram PK, Crump JA, Gupta SK, Miller MA, Mintz ED. 2008. Analysis of data gaps pertaining to Shigella infections in low and medium human development index countries, 1984–2005. Epidemiol Infect 136(5):577–603.
- Li Y, Cao B, Liu B, Liu D, Gao Q, Peng X, 2009. Molecular detection of all 34 distinct O-antigen forms of Shigella. J Med Microbiol 58(Pt 1):69–81.
- Levine MM, Kotloff KL, Barry EM, Pasetti MF, Sztein MB. 2007. Clinical trials of Shigella vaccines: Two steps forward and one step back on a long, hard road. Nat Rev Microbiol 5(7):540–553.
- Allison GE, Verma NK. 2000. Serotype-converting bacteriophages and O-antigen modification in Shigella flexneri. Trends Microbiol 8(1):17–23.
- Guan S, Bastin DA, Verma NK. 1999. Functional analysis of the O antigen glucosylation gene cluster of Shigella flexneri bacteriophage SfX. Microbiology 145(Pt 5):1263–1273.
- Korres H, Mavris M, Morona R, Manning PA, Verma NK. 2005. Topological analysis of GtrA and GtrB proteins encoded by the serotype-converting cassette of Shigella flexneri. Biochem Biophys Res Commun 328(4):1252–1260.
- Mavris M, Manning PA, Morona R. 1997. Mechanism of bacteriophage SfII-mediated serotype conversion in Shigella flexneri. Mol Microbiol 26(5):939–950.
- Stagg RM, Tang SS, Carlin NI, Talukder KA, Cam PD, Verma NK. 2009. A novel glucosyltransferase involved in O-antigen modification of Shigella flexneri serotype 1c. J Bacteriol 191(21):6612–6617.
- Wehler T, Carlin NI. 1988. Structural and immunochemical studies of the lipopolysaccharide from a new provisional serotype of Shigella flexneri. Eur J Biochem 176(2):471–476.
- Stagg RM, Cam PD, Verma NK. 2008. Identification of newly recognized serotype 1c as the most prevalent Shigella flexneri serotype in northern rural Vietnam. Epidemiol Infect 136(8):1134–1140.
- von Seidlein L, Kim DR, Ali M, Lee H, Wang X, Thiem VD, 2006. A multicentre study of Shigella diarrhoea in six Asian countries: Disease burden, clinical manifestations, and microbiology. PLoS Med 3(9):e353.
- Talukder KA, Islam Z, Islam MA, Dutta DK, Safa A, Ansaruzzaman M, 2003. Phenotypic and genotypic characterization of provisional serotype Shigella flexneri 1c and clonal relationships with 1a and 1b strains isolated in Bangladesh. J Clin Microbiol 41(1):110–117.
- Korres H, Verma NK. 2004. Topological analysis of glucosyltransferase GtrV of Shigella flexneri by a dual reporter system and identification of a unique reentrant loop. J Biol Chem 279(21):22469–22476.
- Lehane AM, Korres H, Verma NK. 2005. Bacteriophage-encoded glucosyltransferase GtrII of Shigella flexneri: Membrane topology and identification of critical residues. Biochem J 389(Pt 1):137–143.
- Alexeyev MF, Winkler HH. 1999. Membrane topology of the Rickettsia prowazekii ATP/ADP translocase revealed by novel dual pho-lac reporters. J Mol Biol 285(4):1503–1513.
- Korres H, Verma NK. 2006. Identification of essential loops and residues of glucosyltransferase V (GtrV) of Shigella flexneri. Mol Membr Biol 23(5):407–419.
- Cserzo M, Wallin E, Simon I, von Heijne G, Elofsson A. 1997. Prediction of transmembrane alpha-helices in prokaryotic membrane proteins: The dense alignment surface method. Protein Eng 10(6):673–676.
- Tusnady GE, Simon I. 1998. Principles governing amino acid composition of integral membrane proteins: Application to topology prediction. J Mol Biol 283(2):489–506.
- Rost B, Sander C. 1993. Prediction of protein secondary structure at better than 70% accuracy. J Mol Biol 232(2):584–599.
- Hirokawa T, Boon-Chieng S, Mitaku S. 1998. SOSUI: Classification and secondary structure prediction system for membrane proteins. Bioinformatics 14(4):378–379.
- Sonnhammer EL, von Heijne G, Krogh A. 1998. A hidden Markov model for predicting transmembrane helices in protein sequences. Proc Int Conf Intell Syst Mol Biol 6:175–182.
- Hofmann K, Stoffel W. 1993. TMbase – a database of membrane spanning protein segments. Biol Chem Hoppe-Seyler 374:166.
- von Heijne G. 1992. Membrane protein structure prediction. Hydrophobicity analysis and the positive-inside rule. J Mol Biol 225(2):487–494.
- Sambrook J, Russell WD. 2001. Molecular cloning: A laboratory manual. 3rd ed. New York: Cold Spring Harbor Laboratory Press.
- Sugiyama JE, Mahmoodian S, Jacobson GR. 1991. Membrane topology analysis of Escherichia coli mannitol permease by using a nested-deletion method to create mtlA-phoA fusions. Proc Natl Acad Sci USA 88(21):9603–9607.
- Ehrmann M, Boyd D, Beckwith J. 1990. Genetic analysis of membrane protein topology by a sandwich gene fusion approach. Proc Natl Acad Sci USA 87(19):7574–7578.
- Thanweer F, Tahiliani V, Korres H, Verma NK. 2008. Topology and identification of critical residues of the O-acetyltransferase of serotype-converting bacteriophage, SF6, of Shigella flexneri. Biochem Biophys Res Commun 375(4):581–585.
- Manoil C. 1991. Analysis of membrane protein topology using alkaline phosphatase and beta-galactosidase gene fusions. Methods Cell Biol 34:61–75.
- Miller JH. 1992. A short course in bacterial genetics: A laboratory manual and handbook for Escherichia Coli and related bacteria. New York: Cold Spring Harbor Laboratory Press.
- Carlin NI, Rahman M, Sack DA, Zaman A, Kay B, Lindberg AA. 1989. Use of monoclonal antibodies to type Shigella flexneri in Bangladesh. J Clin Microbiol 27(6):1163–1156.
- Heijne GV. 1986. The distribution of positively charged residues in bacterial inner membrane proteins correlates with the trans-membrane topology. EMBO J 5(11):3021–3027.
- Lasso G, Antoniw JF, Mullins JG. 2006. A combinatorial pattern discovery approach for the prediction of membrane dipping (re-entrant) loops. Bioinformatics 22(14):e290–297.
- Viklund H, Granseth E, Elofsson A. 2006. Structural classification and prediction of reentrant regions in alpha-helical transmembrane proteins: Application to complete genomes. J Mol Biol 361:591–603.
- Jiang Y, Lee A, Chen J, Cadene M, Chait BT, MacKinnon R. 2002. Crystal structure and mechanism of a calcium-gated potassium channel. Nature 417(6888):515–522.
- Savage DF, Egea PF, Robles-Colmenares Y, O'Connell JD 3rd, Stroud RM. 2003. Architecture and selectivity in aquaporins: 2.5 an X-ray structure of aquaporin Z. PLoS Biol 1(3):E72.
- Jordan P, Fromme P, Witt HT, Klukas O, Saenger W, Krauss N. 2001. Three-dimensional structure of cyanobacterial photosystem I at 2.5 A resolution. Nature 411(6840):909–917.
- Xu Q, Yu L, Chitnis VP, Chitnis PR. 1994. Function and organization of photosystem I in a cyanobacterial mutant strain that lacks PsaF and PsaJ subunits. J Biol Chem 269(5):3205–3211.
- Busch C, Hofmann F, Selzer J, Munro S, Jeckel D, Aktories K. 1998. A common motif of eukaryotic glycosyltransferases is essential for the enzyme activity of large clostridial cytotoxins. J Biol Chem 273(31):19566–19572.
- Saxena IM, Brown RM Jr, Fevre M, Geremia RA, Henrissat B. 1995. Multidomain architecture of beta-glycosyl transferases: Implications for mechanism of action. J Bacteriol 177(6):1419–1424.
- Unligil UM, Rini JM. 2000. Glycosyltransferase structure and mechanism. Curr Opin Struct Biol 10(5):510–517.
- Contreras I, Ortiz-Zapater E, Aniento F. 2004. Sorting signals in the cytosolic tail of membrane proteins involved in the interaction with plant ARF1 and coatomer. Plant J 38(4):685–698.
- Zerangue N, Malan MJ, Fried SR, Dazin PF, Jan YN, Jan LY, 2001. Analysis of endoplasmic reticulum trafficking signals by combinatorial screening in mammalian cells. Proc Natl Acad Sci USA 98(5):2431–2436.