Abstract
Coat proteins orchestrate membrane budding and molecular sorting during the formation of transport intermediates. Coat protein complex I (COPI) vesicles shuttle between the Golgi apparatus and the endoplasmic reticulum and between Golgi stacks. The formation of a COPI vesicle proceeds in four steps: coat self-assembly, membrane deformation into a bud, fission of the coated vesicle and final disassembly of the coat to ensure recycling of coat components. Although some issues are still actively debated, the molecular mechanisms of COPI vesicle formation are now fairly well understood. In this review, we argue that physical parameters are critical regulators of COPI vesicle formation. We focus on recent real-time in vitro assays highlighting the role of membrane tension, membrane composition, membrane curvature and lipid packing in membrane remodelling and fission by the COPI coat.
Introduction
The coat protein complex I (COPI) coat mediates membrane transport at the level of the Golgi apparatus by generating 75–100 nm diameter coated vesicles (Orci et al. Citation1986, Malhotra et al. Citation1989) enriched in specific cargoes. COPI is involved in both initial membrane deformation and sorting of the cargo (Bonifacino and Glick Citation2004, Lee et al. Citation2004, Glick and Nakano Citation2009). After budding, membrane fission occurs and the coated vesicle separates form the Golgi membrane. Finally, the coat disassembles and coat components are recycled for another round of budding. The core COPI machinery is now well characterized and comprises the small G protein Arf1, proteins from the p23/p24 family, and the coat complex coatomer. Several reviews have been published recently that describe in detail the current understanding of the molecular mechanisms underlying COPI vesicle formation (Bethune et al. Citation2006, Spang Citation2008, Citation2009, Beck et al. Citation2009b, Hsu and Yang Citation2009, Jackson Citation2009). In vitro reconstitution of the COPI coat either on purified Golgi membranes or in liposome-based assays has been instrumental in identifying the key COPI players. But a fair amount of controversy still remains in the field concerning the nature of the COPI cargoes, the precise role of the three Arf1 GTPase activating proteins ArfGAP1-3, and on how fission of COPI vesicles is achieved (Beck et al. Citation2009b, Emr et al. Citation2009, Hsu and Yang Citation2009). While deciphering the molecular mechanisms of COPI assembly and disassembly is clearly of great importance, it is becoming increasingly evident that the physico-chemical parameters of the membrane, such as membrane composition, membrane tension or membrane curvature, must also play a crucial role (Antonny Citation2006, Sens et al. Citation2008). In this review, we focus on the physical aspects of COPI vesicle formation and on recent in vitro real-time assays that have been developed to study quantitatively the COPI coat. These new approaches bring fresh insights in COPI vesicle formation and could help solving some of the recurring controversies in the COPI field.
Relevant physical parameters
Membranes have been studied by soft matter physicists for more than 40 years and several concepts relevant for intracellular membranes have emerged. Since coat assembly, subsequent membrane deformation and fission and final coat disassembly all require interactions between a protein machinery and lipids, the physico-chemistry of the membrane should clearly play a major role. At the molecular level, the insertion of coat components into the membrane involves protein-lipid interactions. The coat is made of peripheral, membrane-anchored and transmembrane proteins, and lipid headgroups as well as acyl chains interact with the coat machinery through either electrostatic or hydrophobic interactions. The composition of the membrane is thus critical for efficient binding and correct localization of the coat. The cis-Golgi membranes on which the COPI coat assembles are weakly charged (Yeung et al. Citation2008, Leventis and Grinstein Citation2010) and the COPI coat can assemble on quite neutral membranes in vitro, suggesting that electrostatic interactions play only a minor role during COPI binding, in contrast with clathrin binding to the plasma membrane. A key concept here is lipid packing, which characterizes the spacing between lipid polar headgroups (van den Brink-van der Laan et al. Citation2004, Antonny Citation2006). Insertion of coat components should be facilitated if lipid packing is loose or if the membrane exhibits a high density of lipid packing defects. Lipid packing defects can be generated by high membrane curvature which opens space between headgroups or by the geometrical shape of the lipids. For instance, cone-shaped lipids, i.e., with a low headgroup/chains volume ratio, such as diacylglycerols (DAGs), are known to create lipid packing defects ().
Figure 1. Membrane physical parameters relevant for coat proteins interacting with membranes. (A) Lipid packing defects in an initially flat membrane can be created by a stretching force increasing membrane tension, by membrane curvature or by the presence of conical shaped lipids. (B) Relationships between the various physical parameters relevant for COPI vesicle formation. Global parameters (membrane rigidity and membrane tension, in red) and microscopic parameters (membrane composition and lipid packing, in green) impact on each other and on membrane curvature (in blue). An arrow drawn from parameter X to parameter Y means that the value of X affects Y.
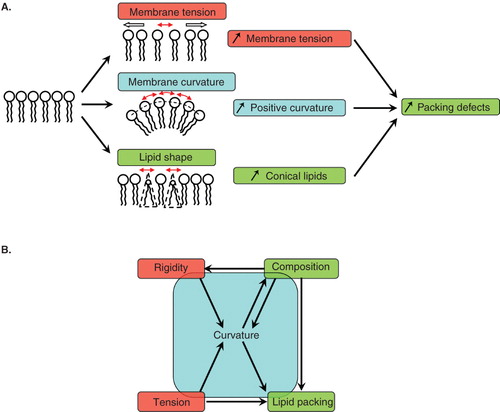
At a more macroscopic scale, the membrane can be described by its bending energy introduced by Canham, Helfrich and Evans in the 1970s (Evans Citation1973a, Citation1973b, Citation1974, Helfrich Citation1973). The bending energy represents the amount of energy needed to bend the membrane to achieve a given curvature. In its simplest form, two parameters contribute to the bending energy: The rigidity (also called bending stiffness or bending modulus) and the tension of the membrane. Intuitively, deforming a rigid and/or tensed membrane is more difficult than deforming a soft and/or floppy membrane. In other words, a low rigidity and a low tension both reduce the bending energy. Since bending the membrane is key to budding and fission events, membrane rigidity and membrane tension are also the two most relevant global parameters for coated vesicle formation.
Membranes made of a mixture of several types of lipids can exhibit phase separation between a liquid disordered (Ld) phase and a liquid ordered (Lo) phase. The typical example is the phosphatidylcholine/cholesterol/sphingomyelin ternary mixture, for which the Ld phase is enriched in phosphatidylcholine and the Lo phase is enriched in sphingomyelin. The Ld phase has a lower rigidity and is easier to bend than the Lo phase. When the two phases are separated, line tension between Ld and Lo domains can create membrane curvature (Julicher and Lipowsky Citation1993), although much weaker than the curvature of a coated bud. recapitulates how microscopic parameters, such as membrane composition and lipid packing, and global parameters, such as membrane tension and membrane rigidity, influence each other and impact on membrane curvature.
In vitro approaches to study coat proteins
About 20 years ago, pioneer studies have identified the COPI coat using an in vitro assay based on purified Golgi membranes. This approach has allowed to characterize the main molecular components of the COPI coat (Donaldson et al. Citation1992, Orci et al. Citation1993a, Citation1993b) and was later complemented by a small liposome based assay to identify the minimal machinery for COPI vesicle formation (Ostermann et al. Citation1993, Spang et al. Citation1998, Bremser et al. Citation1999, Nickel and Wieland Citation2001, Reinhard et al. Citation2003) (). Small liposomes are typically 50–500 nm in diameter and electron microscopy is necessary to observe them after fixation, precluding any time-resolved observation of COPI assembly, budding and fission. However, real-time data on COPI coat assembly and disassembly were obtained using fluorescence or light scattering techniques (Bigay and Antonny Citation2005).
Figure 2. In vitro assays developed to study membrane deformation and fission by coat proteins. Schematic drawings depicting each assay and the effects of coat components (red crescents) binding are shown in the left and middle columns, respectively. (A) Isolated Golgi membranes and small synthetic liposomes. (B) Giant unilamellar vesicles (GUVs) can be aspirated into a micropipette to control and measure membrane tension. Membrane nanotubes are pulled from GUVs either by molecular motors moving on cytoskeletal tracks or by an optical tweezers. Coupling micropipette aspiration with tube pulling by an optical tweezers allows fine tuning of the tube diameter (10–200 nm) via the control of membrane tension and of the force applied by the optical tweezers on the tube. (C) Flat membrane sheets assay. A drop of lipid mix is dried on a glass coverslip. After rehydration, a stack of multilamellar membranes forms with flat sheets at the rims. (D) SUPER (supported bilayers with excess membrane reservoir) templates. 5 μm beads are covered with a lipid bilayer that provides a membrane reservoir. SUPER templates have only been used so far to study the endocytic fission protein dynamin. Note that membrane structure in flat membrane sheets (C) and SUPER templates (D) has not been characterized and the multilayered and folded membranes shown on the drawings only schematically represent the excess membrane reservoir present in these assays. Only the techniques most widely used with each assay are indicated in the column on the right. Note that electron microscopy can also be performed on GUVs, flat membrane sheets or SUPER templates.
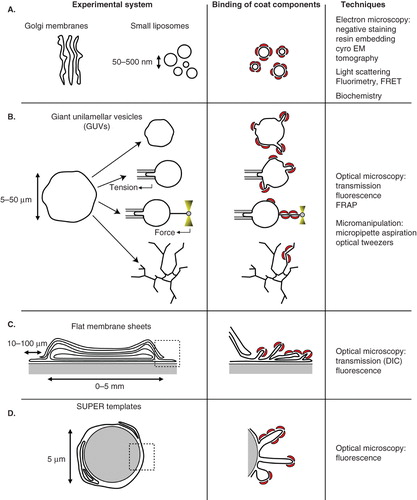
More recently, in vitro techniques using giant liposomes (or GUV for giant unilamellar vesicle) have been developed (reviewed in Walde et al. Citation2010). GUVs are typically 5–50 μm in diameter and well suited for optical microscopy and micromanipulation (). The downside of GUVs compared to classical small liposomes is the low number of vesicles, their weak resistance to mechanical stress and the difficulty to characterize them at the ultrastructural level by electron microscopy. Nevertheless, coupled to advanced fluorescence light microscopy techniques, GUVs are becoming an increasingly popular tool to study the mechanisms of membrane deformation and fission in a wide range of biological processes (Roux et al. Citation2006, Romer et al. Citation2007, Citation2010, Wollert et al. Citation2009, Anitei et al. Citation2010, Ewers et al. Citation2010, Long et al. Citation2010, Wollert and Hurley Citation2010). Besides allowing real-time visualization, one advantage of GUVs over other techniques is to permit control and quantitative measurements of most physical parameters listed on , such as bending rigidity, membrane tension, or membrane curvature. Micropipette aspiration has been widely used to control GUV tension (Kwok and Evans Citation1981, Evans and Rawicz Citation1990, Drury and Dembo Citation1999). More sophisticated set-ups have been devised to pull single membrane tubes (also called membrane tethers) with optical tweezers from GUVs held at a given membrane tension by a micropipette (Cuvelier et al. Citation2005, Sorre et al. Citation2009, Ambroggio et al. Citation2010, Heinrich et al. Citation2010, Roux et al. Citation2010). The tube curvature is set by the membrane tension and can be measured via the force exerted by the tweezers. Tube diameters range between 10 and 200 nm. The membrane composition can simultaneously be visualized with confocal microscopy using fluorescent lipids and fluorescently labelled proteins. Membrane tubes can also be pulled from GUVs using kinesin motors moving on microtubules (Roux et al. Citation2002, Koster et al. Citation2003). With this technique, networks of about 20 nm diameter tubes are formed, but their tension and curvature can not be precisely known.
The GUV membrane composition can be adjusted using controlled mixtures of synthetic lipids (Morales-Penningston et al. Citation2010). GUVs have been formed in buffers at physiological ionic strength (Montes et al. Citation2007, Citation2010, Meleard et al. Citation2009). It is possible to form charged GUVs (Carvalho et al. Citation2008) (up to 20–30% charged lipids, although all charged lipids may not be incorporated in GUVs grown with the electroformation technique) or asymmetric GUVs with a lipid composition in the inner leaflet different from that of the outer leaflet (Pautot et al. Citation2003) and to reconstitute transmembrane proteins in GUVs (Girard et al. Citation2004, Doeven et al. Citation2005, Kahya Citation2010, Varnier et al. Citation2010). GUVs have also been produced from native plasma membranes (Baumgart et al. Citation2007, Lingwood et al. Citation2008, Sengupta et al. Citation2008, Veatch et al. Citation2008), although this remains to be done with membranes purified from the Golgi apparatus or from any other intracellular organelle. Even if GUV assays are performed with physiological buffers and membrane compositions, the physiological relevance of the results may still be questioned. For instance, membrane deformations observed on GUVs incubated with the ESCRT machinery (Wollert et al. Citation2009, Wollert and Hurley Citation2010) are much larger (micrometer-sized) than the corresponding in vivo deformations (<50 nm). Similarly, long tubules (>1 μm) are induced by the COPI coat on GUVs, although few classical 60 nm coated buds were also observed by electron microscopy in this case (Manneville et al. Citation2008). This discrepancy may be due to the large excess area available in GUV membranes, to their low membrane tension, or to the absence in the in vitro assays of additional factors regulating the size of deformations in vivo.
Other real-time techniques involve multilayered membranes. The first consists in forming flat membrane sheets on a glass coverslip () and was originally developed to observe tubulation by BAR domain containing proteins and dynamin (Itoh et al. Citation2005, Roux et al. Citation2006). This assay was later used to study the effects of binding Arf family proteins to membranes (Beck et al. Citation2008, Krauss et al. Citation2008). The lipid composition appears to be more restricted than in the GUV assay. In most cases, strongly negatively charged lipid mixtures are necessary for efficient sheet formation. The second assay produces supported lipid bilayers around 5 μm beads (). These so-called SUPER (for supported bilayers with excess membrane reservoir) templates were introduced to follow membrane fission by dynamin in real-time (Pucadyil and Schmid Citation2008) but have not been involved in any COPI coat study to date. Both flat membranes and SUPER templates are convenient to observe morphological changes upon protein binding since they provide a large membrane reservoir at near zero membrane tension. However, as opposed to the GUV assay, control and quantification of membrane parameters, notably membrane tension, is difficult. Possible interactions with the substrate, either glass coverslip or silica bead, also makes the interpretation of the results more difficult. Similarly, experiments on membrane deformation of small liposomes should be interpreted with caution when negative staining on carbon-coated grids is used for electron microscopy. A precedent was set in a study showing that membrane fission by dynamin is observed only when liposomes are visualized by negative staining and not by cryo-EM, suggesting that adhesion to the substrate generates membrane tension and leads to fission (Danino et al. Citation2004).
Finally, recent advances in micro-fabrication allow the production of curved substrates that could prove useful in studying the binding of coat components to surfaces of controlled curvature (Parthasarathy and Groves Citation2007, Qin et al. Citation2010).
Assembly of the COPI coat
The first step in COPI coat assembly is the recruitment of Arf1 to Golgi membranes. When bound to GDP, Arf1 can interact with membranes indirectly by binding to transmembrane proteins of the p23/p24 family (Beck et al. Citation2009b). Upon GDP to GTP exchange, a conformational change in Arf1 exposes a short N-terminal amphipathic helix and a myristoyl modification that allows direct interaction of Arf1-GTP with the lipid bilayer (Antonny et al. Citation1997). At the cis-Golgi, the Arf1 exchange factor (GEF) GBF1 stimulates GDP to GTP exchange. The physical state of the membrane could play a role in both Arf1-GDP and Arf1-GTP recruitment by controlling Arf1 insertion and/or the localization of p23/p24 or GBF1.
Membrane composition is an obvious parameter that could influence COPI coat recruitment. COPI has mostly been involved in retrograde transport from the Golgi apparatus to the endoplasmic reticulum (ER). Consistently, its main localization is on the cis side of the Golgi apparatus (Oprins et al. Citation1993). But the COPI coat also mediates intra-Golgi and ERGIC (ER to Golgi intermediate compartment) to Golgi transport steps and should thus be able to assemble on most membranes of the early secretory pathway. The lipid composition of membranes in the early secretory pathway varies, with a progressive enrichment in sterols and sphingolipids from the cis to the trans side of the Golgi apparatus (van Meer Citation1998, Holthuis and Levine Citation2005, van Meer et al. Citation2008). COPI vesicles could play a role in such a sorting of lipids. A mass-spectrometry study showed that the lipid composition of COPI vesicles differs from that of the donor Golgi membranes (Brugger et al. Citation2000). COPI vesicles are enriched in phosphatidylcholines and depleted in sphingomyelin and cholesterol compared to Golgi membranes. The fact that COPI-mediated transport mainly drives vesicles from the cis-Golgi to the ER could explain why the ER contains less sphingomyelin and cholesterol than the cis-golgi. Two mechanisms have been invoked for lipid sorting. First, curvature has been shown to induce lipid sorting in a homogeneous membrane in vitro by enriching curved membranes in lipids of the Ld phase such as phosphatidylcholines and depleting them in cholesterol and sphingomyelin (Roux et al. Citation2005, Sorre et al. Citation2009, Tian and Baumgart Citation2009). During budding, curvature induced by the COPI coat could thus enrich the bud in phosphatidylcholine. This hypothesis fits with the increased phosphatidylcholine content reported for COPI vesicles. However, this mechanism is efficient only when the membrane composition is close to phase transition (Sorre et al. Citation2009), which may not be the case for cis-Golgi membranes, and several rounds of budding may be necessary to enhance sorting. Furthermore, it was shown recently that transport carriers from the trans-Golgi network (TGN) to the plasma membrane contain more sterols and sphingolipids than the trans-Golgi. These results are consistent with the increasing sterol and sphingolipids content of membranes down the secretory pathway, but they are difficult to reconcile with the curvature-mediated lipid sorting hypothesis except if Lo lipids can be clustered by a protein negatively coupled to membrane curvature (M. Safouane, P. Bassereau personal Communication). A second mechanism is based on demixing between small scale domains of Ld and Lo phases (). While evidence for nanometer-sized dynamic ‘rafts’ at the plasma membrane is accumulating (Lingwood and Simons Citation2010), the presence of similar domains on Golgi membranes is still actively debated. A model of transport through the Golgi apparatus has recently been developed based on partitioning of cargoes and Golgi enzymes within different lipid phases (Patterson et al. Citation2008). Experiments on GUVs have shown that the COPI coat specifically assembles on domains of the Ld phase and is excluded from the Lo phase, suggesting that if lipid domains exist on the Golgi membrane, COPI vesicles may specifically bud from Ld ‘non-raft’ domains (Manneville et al. Citation2008). However, lipid segregation in different domains is not necessarily required to explain sorting. Even on a homogeneous mixture of Ld and Lo lipids, preferential interactions of the COPI machinery with Ld lipids could induce sorting (). For instance, clustering of Lo lipids by Shiga toxin at the plasma membrane has been shown to favour sorting of these lipids into tubules (M. Safouane, P. Bassereau personal Communication). The binding of Arf1 on GUVs made of a homogeneous mixture of sphingomyelin, cholesterol and phosphatidylcholine increases with increasing amounts of Ld lipids (Manneville et al. unpublished results), consistent with the preferential binding of Arf1 to Ld phase in demixed GUVs. Moreover, when Arf1 and coatomer are bound to homogeneous GUVs, domains enriched in Arf1 and coatomer can form in which the local concentration of phosphatidylcholine appears to be increased (Manneville et al. Citation2008). Arf1 dimerization could further enhance phosphatidylcholine clustering by COPI. Although direct evidence is still lacking, these observations suggest that COPI binding could locally enrich the membrane in Ld lipids relative to Lo lipids creating ‘hot spots’ for COPI vesicle formation ().
Figure 3. Role of lipid domains in COPI coat assembly, vesicle budding and fission. (A) The COPI coat preferentially assembles on Ld domains of a phase separated membrane exhibiting distinct Ld (green) and Lo (red) domains. Whether such domains exist on Golgi membranes is not clear, especially at the cis-Golgi where the concentration of sphingolipids is very low. After initial recruitment of COPI components in Ld domains, COPI bends the membrane into a bud. If the size of the Ld domain corresponds to a 50–60 nm bud, line tension between the Ld bud and the Lo flat membrane could facilitate membrane fission. (B) On a homogeneous membrane containing Ld and Lo lipids, COPI components interact preferentially with Ld lipids and cluster them in the nascent bud. Local enrichment of Ld lipids in COPI-coated areas may be enhanced by Arf1 dimerization. Positive curvature during budding further sorts Ld lipids in the COPI bud. Line tension between the Ld enriched bud and the comparatively Ld depleted donor membrane could help fission. As suggested in vitro and in clathrin-independent endocytosis, actin polymerization could participate in phase separation and trigger fission. Note that only Arf1 and coatomer are represented as coat components for clarity.
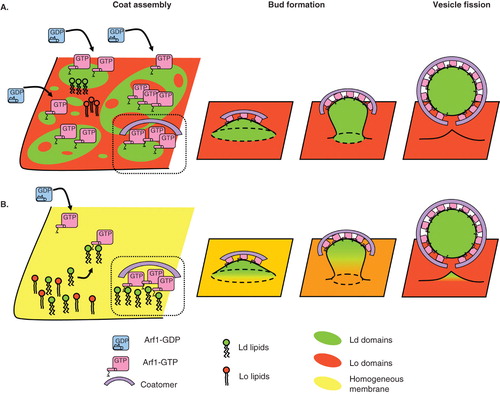
Membrane curvature could also influence the initial binding of the COPI coat. It was proposed in a small liposome assay that Arf1 functions as a GTP-dependent curvature sensor and inducer that could help initiate COPI vesicle assembly (Lundmark et al. Citation2008). However, when compared to the prototypical curvature sensor ArfGAP1 (see point 7 below), Arf1 is only weakly sensitive to curvature with a 5 fold increase in binding to membrane tubes pulled from GUVs compared to a 100 fold increase for ArfGAP1 when the tube diameter is decreased from 100–20 nm (Ambroggio et al. Citation2010). Even if the COPI coat can readily assemble on GUVs under high membrane tension into weakly curved coats (Manneville et al. Citation2008), ultrastructural observations of Golgi membranes show that COPI vesicles are mostly found near the curved rims of the Golgi cisternae (Weidman et al. Citation1993, Bouchet-Marquis et al. Citation2008), suggesting that, even if cisternae rims are curved only in one direction, budding may preferentially occur from curved membranes in vivo. As Arf1 binding does not appear to be restricted to the rims of the cisternae (Orci et al. Citation1993a), other COPI components could provide sensitivity to curvature. ArfGAP1 has been implicated in early stages of COPI assembly and could mediate localization of COPI budding to the rims. The full structure of the self-assembled seven subunit coatomer complex is not known to date and, in contrast with the COPII and clathrin coats, no electron cryomicroscopy image of COPI reconstituted cages is available. However, the X-ray crystal structures of the αβ′-COP and of the αϵ-COP subcomplexes were solved very recently (Hsia and Hoelz Citation2010, Lee and Goldberg Citation2010). From these data, a COPI cage design intermediate between the clathrin and the COPII cages was deduced (Lee and Goldberg Citation2010). Such a curved structure could energetically favour binding of coatomer at cisternae rims. Moreover, as mentioned above, enrichment of Ld lipids due to strong curvature could localize COPI binding at the rims. Finally, a dense Golgi matrix on the flat parts of the cisternae may make budding from these regions less likely (Short et al. Citation2005).
Budding of COPI-coated membranes
Binding of COPI components induces membrane deformation into curved 50–60 nm buds. Several generic mechanisms for membrane bending have been proposed, such as helix insertion, scaffolding by proteins, lipid shape, or action by molecular motors or cytoskeletal elements (Farsad and De Camilli Citation2003, McMahon and Gallop Citation2005). Most, if not all, of these mechanisms probably play a role during COPI vesicle budding. Clearly the easier the membrane will be to deform, the more budding will occur. As mentioned above, the energy to bend a membrane is dominated by its bending modulus and its tension and both parameters should be critical for efficient budding.
Here again, membrane composition has a central role. The Ld phase has a lower bending modulus than the Lo phase and is more prone to deformation (Roux et al. Citation2005). Since the COPI coat appears to specifically assemble on membranes rich in Ld lipids, membrane deformation should be facilitated. The insertion of amphipathic helices also depends on membrane composition and lipid packing. At least two COPI components, Arf1 and ArfGAP1, rely on amphipathic helices to bind to membranes, although the physico-chemical properties of these helices are quite different (Antonny Citation2006). Helix insertion has been proposed to induce membrane curvature by increasing the area of the outer leaflet according to the so-called ‘bilayer-couple’ mechanism (Sheetz and Singer Citation1974). In the case of Arf1, insertion of a short 7 amino-acid amphipathic helix and a myristoyl modification has been shown to tubulate small liposomes or flat membrane sheets (Beck et al. Citation2008, Krauss et al. Citation2008, Lundmark et al. Citation2008). Arf1 was proposed to induce initial membrane bending that would further be stabilized by coatomer (Lundmark et al. Citation2008). A similar mechanism was suggested for the initiation of clathrin-coated vesicle formation by FCHo proteins (Henne et al. Citation2010). Interestingly, Arf1 dimerization was shown to be critical for its membrane tubulating activity (Beck et al. Citation2008). In contrast, a study on GUVs showed that Arf1 alone is not sufficient to bend the membrane and that both Arf1 and coatomer are required for efficient membrane deformation (Manneville et al. Citation2008), in agreement with earlier work (Orci et al. Citation1993a, Citation1993b) and questioning the relevance of Arf1-induced tubulation. These conflicting results may be explained by the different membrane compositions and Arf1 concentrations used in the GUV study and in the small liposome and flat membrane sheets studies. While a Golgi-like composition was used in (Manneville et al. Citation2008), PIP2 and other charged lipids were present and Arf1 concentration was ten fold higher in (Beck et al. Citation2008, Krauss et al. Citation2008, Lundmark et al. Citation2008). The methods to bind Arf1-GTP also differ. Binding Arf1-GTP via the Arf6 exchange factor ARNO as in (Beck et al. Citation2008) requires PIP2 and may lead to higher local concentrations of Arf1-GTP than when Arf1-GTP is bound by lowering the free Mg2+ concentration with EDTA (Manneville et al. Citation2008). Arf1 dimerization is achieved with concentrations above 1 μM (Beck et al. Citation2008). The precise concentration of Arf1 on Golgi membranes during COPI budding in cells is not known. It is possible that coatomer clusters Arf1 and increases the local Arf1 concentration (Manneville et al. Citation2008). Arf1tubulating activity could then cooperate with coatomer to bend the membrane.
A similar debate regarding local concentrations applies to ArfGAP1. The Arf1 GTPase activating protein ArfGAP1 has been proposed to act as a stoechiometric component of the coat involved in cargo selection and vesicle formation (Yang et al. Citation2002, Hsu and Yang Citation2009) or as a catalytic enzyme dissociating Arf1-GTP from the Golgi membrane by GTP hydrolysis in Arf1-GTP and allowing coat disassembly (Tanigawa et al. Citation1993, Reinhard et al. Citation2003, Beck et al. Citation2009a, Hsu and Yang Citation2009). Knowing the ArfGAP1 concentration in the COPI coat, whether in stoechiometric or catalytic amounts with other COPI components such as Arf1 or coatomer, is crucial for in vitro reconstituted assays. ArfGAP1 inserts two long ∼30 amino-acid amphipathic helices known as the ALPS (amphipathic lipid packing sensor) domains when it binds to membranes exhibiting lipid packing defects either due to membrane curvature or to the presence of conical lipids (Bigay et al. Citation2003, Citation2005, Drin et al. Citation2007). When used in catalytic amounts (in the nM range), ArfGAP1 has been described as a curvature sensor since it specifically binds curved membranes. However, insertion of the ALPS motifs on initially flat membranes may be enforced by higher (in the μM range) ArfGAP1 concentrations. According to the ‘bilayer-couple’ mechanism, stoechiometric amounts of ArfGAP1 may thus induce membrane bending. ArfGAP1 could contribute to budding by acting in early steps of COPI assembly as a stoechiometric coat component. But this seems unlikely according to a recent study which revisited the experiments supporting a role of ArfGAP1 as a stoechiometric component of the coat (Beck et al. Citation2009a). The authors concluded that ArfGAP1 is not required for COPI vesicle formation and that Arf1, and not ArfGAP1, is a stoechiometric component of the coat.
Besides helix insertion, two other mechanisms, scaffolding and pulling by motor proteins, probably participate in membrane deformation. The electron-dense coat seen on COPI vesicles by electron microscopy has been attributed to a self-assembled lattice of coatomer. Whether coatomer forms cages such as clathrin (Fotin et al. Citation2004) or the Sec13/31 complex in the COPII coat (Stagg et al. Citation2006, Fath et al. Citation2007) is still not known. But it is tempting to speculate that coatomer does assemble in regular lattices of a given curvature by analogy with the other two coats, or at least that its scaffolding can generate curvature or stabilize a pre-existing curvature. After fission, vesicles are transported to their acceptor compartment by molecular motors moving on cytoskeletal tracks. Molecular motors could also act earlier and participate in membrane deformation since motors may directly interact with coat components, as it is the case for the clathrin and COPII coats (Nakagawa et al. Citation2000, Watson et al. Citation2005). By pulling on the membrane during budding, motors would not only help membrane deformation but also put the membrane under tension which may then facilitate membrane fission ( and next section).
Figure 4. COPI vesicle formation is regulated by membrane tension. (A) At low membrane tension (low σ), the COPI coat assembles with its preferred curved geometry and bends the membrane. From this point, an increase in membrane tension should prevent further deformation. If membrane tension stays low or drops, the COPI coat moulds the membrane into a bud. Tension could then participate in the final fission step. A sudden change in tension (either rise or drop) could destabilize the bud and facilitate fission. Actin polymerization or molecular motors moving on cytoskeletal elements (myosins on actin or kinesins/dynein on microtubules) and interacting with coat proteins could stretch the membrane in the bud neck, increase its tension and trigger fission. (B) At high membrane tension (high σ), the coat may not able to assemble in its curved geometry and form flat lattices. If tension stays high or increases further, the coat may disassemble in an unproductive round of COPI assembly. If tension drops, the coat probably stays in its flat geometry and has to disassemble completely before reassembling into a curved geometry. Dotted lines denote more hypothetical steps.
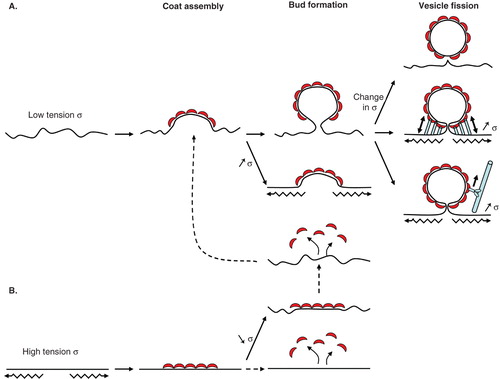
Concerning membrane tension during the initial steps of membrane deformation, it is intuitively clear that the COPI coat should deform membranes under low tension more easily than membranes under high tension (). Qualitative experiments on GUVs confirmed this idea (Manneville et al. Citation2008). The pore forming toxin streptolysin-O (SLO) was used to reduce membrane tension to almost zero. In the presence of SLO, Arf1 and coatomer induce almost 10 times more deformation than in the absence of SLO. These results suggest that a threshold tension exists below which COPI efficiently deforms the membrane. The measurement of this threshold is still lacking but could be obtained by micropipette aspiration experiments. It will then be interesting to compare the threshold value to in vitro data obtained by pulling tube networks from Golgi membranes showing that the tension of the Golgi apparatus is around 5 10−6 N/m (Upadhyaya and Sheetz Citation2004). At high membrane tension, the COPI coat appears to assemble as flat lattices on GUV membranes (Manneville et al. Citation2008). Although it cannot be ruled out that these lattices are actually composed of buds below the resolution of optical microscopy, these results suggest that, like clathrin (Harrison and Kirchhausen Citation1983, Ungewickell and Hinrichsen Citation2007), coatomer can self-assemble into different geometries. Here again, the determination of the high-resolution structure of the seven subunit self-assembled COPI cage should help resolve this issue (Hsia and Hoelz Citation2010, Lee and Goldberg Citation2010).
Fission of COPI vesicles
Despite intensive work, the fission step remains the most mysterious step of COPI vesicle formation. Arf1 and coatomer were initially thought to be sufficient to drive fission in vitro (Orci et al. Citation1993a). But the use of Golgi membranes, which may still contain bound proteins after washing, and/or cytosol in these early studies complicate the interpretation of the results. Later, small liposomes incubated with purified proteins were observed with electron microscopy (Spang et al. Citation1998, Bremser et al. Citation1999, Reinhard et al. Citation2003). Although COPI vesicle formation was reported in these conditions, acidic phospholipids such as phosphatidic acid (Spang et al. Citation1998) stimulated the yield of vesicle formation. Moreover the electron microscopy technique used did not allow to precisely discriminate separated vesicles from buds still attached to the liposome by a thin neck. More recently, real-time assays re-evaluated the fission step. Assembly of Arf1 and coatomer does not appear to drive fission on GUVs (Manneville et al. Citation2008). GUV membranes are deformed in long tubules or protrusions that stay connected to the GUV. As for Arf1-induced membrane tubulation, the concentration of Arf1 and its dimerization may be critical for fission, especially since the helix of Sar1, the small G protein equivalent to Arf1 in the COPII coat, has been shown to participate in fission (Bielli et al. Citation2005, Lee et al. Citation2005, Long et al. Citation2010). On GUV membranes, Arf1 may not be saturated thus preventing fission of membrane deformations.
In any case, more data, such as electron microscopy tomography of small liposomes, is needed to convincingly show that Arf1 and coatomer are the only components required for vesicle formation. Accessory proteins and actin are required to complete fission of a clathrin coated vesicle (Kirchhausen Citation2009). In clathrin-independent endocytosis, actin and membrane organization play a central role (Ewers et al. Citation2010). It is also not clear whether only Sar1 and the Sec23/24-Sec13/31 complex are sufficient for COPII vesicle fission. Similarly, many studies in the COPI field show that additional factors besides Arf1 and coatomer participate in COPI vesicle fission. Interestingly, these factors all impact either on membrane composition or on membrane tension.
First, membrane composition plays a clear role in fission, principally through the involvement of two lipid species, diacylglycerols and phosphatidic acid. It has recently been shown that COPI vesicle formation needs diacylglycerols (DAGs) in vivo (Fernandez-Ulibarri et al. Citation2007, Asp et al. Citation2009), although whether DAGs acts at a late fission step (Fernandez-Ulibarri et al. Citation2007) or earlier during budding (Asp et al. Citation2009) is not clear. In vitro reconstitution experiments should clarify this issue. Phosphatidic acid (PA) was originally involved in fission through its production from lyso-PA and acyl-CoAs by the acyltransferase activity of Brefeldin-A ADP-Ribosylated Substrate (CtBP/BARS) (Weigert et al. Citation1999). Although acyl-CoAs had been previously implicated in COPI fission (Ostermann et al. Citation1993) and BARS is able to tubulate small liposomes in the presence of PA (Yang et al. Citation2008), BARS acyltransferase activity was shown not to be intrinsic to BARS (Gallop et al. Citation2005, Hsu and Yang Citation2009). Rather PA production by phospholipase D type 2 (PLD2) is required for COPI vesicle formation at a late fission stage, as shown in vivo by PLD2 depletion using siRNA or in vitro with Golgi membranes (Yang et al. Citation2008). Interestingly, PKD-dependent fission at the trans-Golgi network (TGN) also requires DAGs (Bossard et al. Citation2007). Both DAGs and PA have a conical shape and create lipid packing defects in a flat lipid bilayer that could facilitate the insertion of COPI coat components such as ArfGAP1, or even Arf1 to a more minor extent as discussed above (see section entitled In vitro approaches to study coat proteins). This would explain a role of DAGs and PA in early steps of budding. But the conical shape of DAGs and PA could also play a role at later stages in fission by inducing local negative membrane curvature and further constricting the neck of the budding vesicle.
Phase separation between Ld and Lo phases could also be involved in fission (Liu et al. Citation2006). Lipid tubes pulled by kinesin motors from GUVs made of an initially homogeneous phosphatidylcholine/cholesterol/sphingomyelin composition close to phase separation undergo fission upon phase separation due to the build-up of line tension between Ld and Lo domains (Roux et al. Citation2005). Selective sorting of Ld lipids by the COPI coat during budding could favour phase separation and fission (). Actin polymerization induces phase separation of initially homogeneous vesicles (Liu and Fletcher Citation2006). Consistently, fission of Shiga-toxin induced plasma membrane invaginations is driven by actin-dependent domain formation (Romer et al. Citation2010). Although cholesterol is critical in this mechanism, a putative role for actin in Golgi membrane organization should not be overlooked.
Additional factors involved in COPI vesicle formation link fission to membrane tension. In the endocytic pathway, a high membrane tension was shown to be required for membrane fission by dynamin (Danino et al. Citation2004, Roux et al. Citation2006). In cells, depolymerization of the actin cytoskeleton facilitates deformation of the plasma membrane into tubules but inhibits fission by dynamin, suggesting that adhesion to the actin cortex tunes membrane tension to allow both deformation and fission (Itoh et al. Citation2005, Antonny Citation2006). Actin has also been involved in the biogenesis of Golgi-derived transport carriers (Chen et al. Citation2005, Egea et al. Citation2006). A signalling pathway linking COPI assembly and actin polymerization has been identified. Coatomer interacts with Golgi-associated Cdc42 to recruit actin via the actin nucleator N-Wasp and Arp2/3 (Wu et al. Citation2000, Matas et al. Citation2004). An in vitro GUV assay reproduced COPI-dependant recruitment of actin and showed that actin polymerization could squeeze the GUV membrane to push forward micron-sized daughter vesicles that can eventually separate from the mother GUV (Heuvingh et al. Citation2007). The forces generated by actin polymerization pushing between the COPI bud away from the membrane could be sufficient to increase membrane tension and cause the fission of the COPI coated vesicle (). Alternatively, tension could be provided in vivo by molecular motors moving on cytoskeletal tracks. Several myosins connect the Golgi apparatus to F-actin (Allan et al. Citation2002) and actomyosin contractility induces fission of transport carriers from the TGN via Myosin II (Miserey-Lenkei et al. Citation2010) and via the unconventional myosin MYO18 (Dippold et al. Citation2009). Evidence for such interactions between actin motors and the COPI machinery at the cis side of the Golgi apparatus is still lacking. Nevertheless, the dynein complex interacts directly with the COPII coat (Watson et al. Citation2005) and coatomer-bound Cdc42 recruits dynein to COPI vesicles (Chen et al. Citation2005) suggesting that microtubule-associated motors may provide tension on membranes of the early secretory pathway.
Disassembly of the COPI coat
The final step in COPI vesicle formation is the disassembly of the coat to allow further transport by molecular motors along the cytoskeleton, and tethering and fusion to the acceptor membrane (Spang Citation2008, Citation2009). The current model is that ArfGAPs act after fission to trigger GTP hydrolysis in Arf1-GTP and dissociate the coat. It is still not clear which ArfGAP(s) is/are involved and what the timing of coat disassembly is. Since ArfGAP2 and 3, but not ArfGAP1, catalyse GTP hydrolysis in a coatomer-dependent manner (Weimer et al. Citation2008, Kliouchnikov et al. Citation2009, Luo et al. Citation2009), ArfGAP2 and 3 may be better candidates than ArfGAP1 to mediate coat disassembly (Beck et al. Citation2009b).
Although ArfGAP1 catalytic activity has been involved in early stages of COPI vesicle biogenesis, ArfGAP1 has also been shown to be an exquisite curvature sensor, suggesting a role at later steps when the membrane is strongly bent (Bigay et al. Citation2005). Because ArfGAP1 activity increases when curvature increases in a small liposome assay, Arf1 should dissociate from the forming bud. The coat could stay bound to the bud via lateral interactions within coatomer and via a ring of Arf1-GTP that resists the action of ArfGAP1 at the base of the bud due to an inverse membrane curvature in this area. When fission occurs, curvature in the neck inverts and ArfGAP1 can hydrolyse the remaining Arf1-GTP. ArfGAP1 would thus prepare the coat in a metastable state for rapid uncoating triggered by fission (Bigay et al. Citation2003). This model was recently refined by quantitative experiments on membranes tubes pulled from GUVs by optical tweezers, a geometry in which the curved and flat membranes are continuous, more representative of the budding stage of coated vesicle formation (Ambroggio et al. Citation2010). A threshold radius of 35 nm was found below which the ALPS domains of ArfGAP1 are able to bind the tube membrane, while Arf1 binds independently of membrane curvature. Surprisingly, when ArfGAP1 is added in catalytic amounts (10 nM) on membrane tubes of diameter below 35 nm, Arf1 is not completely dissociated from the tube but its concentration decreases from the base to the tip of the tube. This result suggests that Arf1-GTP can replace hydrolyzed Arf1 by diffusion from the GUV into the tube. The competition between GTP hydrolysis in Arf1 by ArfGAP1 activity and diffusion of new Arf1-GTP from the GUV sets the size of the Arf1 concentration gradient. The gradient extends up to several μm in the in vitro experiments, suggesting that, on the scale of a COPI bud, diffusion of Arf1-GTP should win over hydrolysis by ArfGAP1. The initial model described above should be revisited to take into account that Arf1 may continuously cycle between GTP-bound and GDP-bound forms inside the budding vesicle, adding stability to the coat before fission. In this new model, fission again triggers coat disassembly. When the COPI vesicle is separated from the Golgi membrane, diffusion of Arf1-GTP into the bud is impaired and ArfGAP1 can rapidly dissociate the coat by one round of hydrolysis of all Arf1-GTP present in the vesicle (Ambroggio et al. Citation2010).
Coat disassembly is required for or coupled to tethering and subsequent fusion of COPI vesicles (Jackson Citation2009, Ren et al. Citation2009, Zink et al. Citation2009). Membrane curvature sensing has been proposed to orient tethering (Drin et al. Citation2008). The golgin GMAP-210 is a long coiled-coiled protein that could tether specifically via an N-terminal ALPS motif uncoated curved vesicles to the flat Golgi cisternae by interacting with Arf1 through a C-terminal GRAB motif. Tethering between vesicles is prevented by ArfGAP1 which dissociates Arf1 and hence GMAP-210 from budding vesicles. Such a physical self-organizing mechanism could play a role in the maintenance of the Golgi architecture (Drin et al. Citation2008).
Concluding remarks
Despite recent progresses, several controversies still remain in the COPI field. Notably, how membrane fission of a COPI vesicle is achieved is still not fully elucidated. ArfGAP1 has been involved in most steps of COPI vesicle formation, including fission (Yang et al. Citation2006), but its precise role is not clear. ArfGAP1 recruitment strongly depends on membrane curvature and on the presence of DAGs. Its enzymatic activity dissociates Arf1-GTP and coatomer from membranes making a budding coat very unstable. ArfGAP1 also interacts with many partners (Inoue and Randazzo Citation2007) including BARS (Yang et al. Citation2005). New real-time in vitro assays based in particular on GUVs should be helpful in clarifying the role of ArfGAP1 and reconciling these observations.
During the complex process of COPI vesicle formation, it is becoming clear that not only molecular factors are important but also that membrane physical parameters actively participate. An increasing number of in vitro experiments supports a role for membrane tension, membrane composition or membrane curvature at each step of COPI vesicle assembly. The key issue is now to test these hypotheses in vivo. Because intracellular compartments are difficult to access, new experimental tools have to be developed to probe membrane tension, composition or curvature inside the cell.
Acknowledgements
We thank the Bruno Antonny lab, the Felix Wieland lab and the Patricia Bassereau lab for stimulating discussions and Patricia Bassereau for comments on the manuscript. We apologize to colleagues whose work could not be cited due to space limitations.
Declaration of interest: The authors report no conflicts of interest. The authors alone are responsible for the content and writing of the paper.
References
- Allan VJ, Thompson HM, McNiven MA. 2002. Motoring around the Golgi. Nat Cell Biol 4:E236–242.
- Ambroggio E, Sorre B, Bassereau P, Goud B, Manneville JB, Antonny B. 2010. ArfGAP1 generates an Arf1 gradient on continuous lipid membranes displaying flat and curved regions. Embo J 29:292–303.
- Anitei M, Stange C, Parshina I, Baust T, Schenck A, Raposo G, Kirchhausen T, Hoflack B. 2010. Protein complexes containing CYFIP/Sra/PIR121 coordinate Arf1 and Rac1 signalling during clathrin-AP-1-coated carrier biogenesis at the TGN. Nat Cell Biol 12:330–340.
- Antonny B. 2006. Membrane deformation by protein coats. Curr Opin Cell Biol 18:386–394.
- Antonny B, Beraud-Dufour S, Chardin P, Chabre M. 1997. N-terminal hydrophobic residues of the G-protein ADP-ribosylation factor-1 insert into membrane phospholipids upon GDP to GTP exchange. Biochemistry 36:4675–4684.
- Asp L, Kartberg F, Fernandez-Rodriguez J, Smedh M, Elsner M, Laporte F, Barcena M, Jansen KA, Valentijn JA, Koster AJ, Bergeron JJ, Nilsson T. 2009. Early stages of Golgi vesicle and tubule formation require diacylglycerol. Mol Biol Cell 20:780–790.
- Baumgart T, Hammond AT, Sengupta P, Hess ST, Holowka DA, Baird BA, Webb WW. 2007. Large-scale fluid/fluid phase separation of proteins and lipids in giant plasma membrane vesicles. Proc Natl Acad Sci USA 104:3165–3170.
- Beck R, Adolf F, Weimer C, Bruegger B, Wieland FT. 2009a. ArfGAP1 activity and COPI vesicle biogenesis. Traffic 10:307–315.
- Beck R, Rawet M, Wieland FT, Cassel D. 2009b. The COPI system: molecular mechanisms and function. FEBS Lett 583:2701–2709.
- Beck R, Sun Z, Adolf F, Rutz C, Bassler J, Wild K, Sinning I, Hurt E, Brugger B, Bethune J, Wieland F. 2008. Membrane curvature induced by Arf1-GTP is essential for vesicle formation. Proc Natl Acad Sci USA 105:11731–11736.
- Bethune J, Wieland F, Moelleken J. 2006. COPI-mediated transport. J Membr Biol 211:65–79.
- Bielli A, Haney CJ, Gabreski G, Watkins SC, Bannykh SI, Aridor M. 2005. Regulation of Sar1 NH2 terminus by GTP binding and hydrolysis promotes membrane deformation to control COPII vesicle fission. J Cell Biol 171:919–924.
- Bigay J, Antonny B. 2005. Real-time assays for the assembly-disassembly cycle of COP coats on liposomes of defined size. Methods Enzymol 404:95–107.
- Bigay J, Casella JF, Drin G, Mesmin B, Antonny B. 2005. ArfGAP1 responds to membrane curvature through the folding of a lipid packing sensor motif. Embo J 24:2244–2253.
- Bigay J, Gounon P, Robineau S, Antonny B. 2003. Lipid packing sensed by ArfGAP1 couples COPI coat disassembly to membrane bilayer curvature. Nature 426:563–566.
- Bonifacino JS, Glick BS. 2004. The mechanisms of vesicle budding and fusion. Cell 116:153–166.
- Bossard C, Bresson D, Polishchuk RS, Malhotra V. 2007. Dimeric PKD regulates membrane fission to form transport carriers at the TGN. J Cell Biol 179:1123–1131.
- Bouchet-Marquis C, Starkuviene V, Grabenbauer M. 2008. Golgi apparatus studied in vitreous sections. J Microsci 230:308–316.
- Bremser M, Nickel W, Schweikert M, Ravazzola M, Amherdt M, Hughes CA, Sollner TH, Rothman JE, Wieland FT. 1999. Coupling of coat assembly and vesicle budding to packaging of putative cargo receptors. Cell 96:495–506.
- Brugger B, Sandhoff R, Wegehingel S, Gorgas K, Malsam J, Helms JB, Lehmann WD, Nickel W, Wieland FT. 2000. Evidence for segregation of sphingomyelin and cholesterol during formation of COPI-coated vesicles. J Cell Biol 151:507–518.
- Carvalho K, Ramos L, Roy C, Picart C. 2008. Giant unilamellar vesicles containing phosphatidylinositol(4,5)bisphosphate: Characterization and functionality. Biophys J 95:4348–4360.
- Chen JL, Fucini RV, Lacomis L, Erdjument-Bromage H, Tempst P, Stamnes M. 2005. Coatomer-bound Cdc42 regulates dynein recruitment to COPI vesicles. J Cell Biol 169:383–389.
- Cuvelier D, Derenyi I, Bassereau P, Nassoy P. 2005. Coalescence of membrane tethers: Experiments, theory, and applications. Biophys J 88:2714–2726.
- Danino D, Moon KH, Hinshaw JE. 2004. Rapid constriction of lipid bilayers by the mechanochemical enzyme dynamin. J Struct Biol 147:259–267.
- Dippold HC, Ng MM, Farber-Katz SE, Lee SK, Kerr ML, Peterman MC, Sim R, Wiharto PA, Galbraith KA, Madhavarapu S, Fuchs GJ, Meerloo T, Farquhar MG, Zhou H, Field SJ. 2009. GOLPH3 bridges phosphatidylinositol-4- phosphate and actomyosin to stretch and shape the Golgi to promote budding. Cell 139:337–351.
- Doeven MK, Folgering JH, Krasnikov V, Geertsma ER, van den Bogaart G, Poolman B. 2005. Distribution, lateral mobility and function of membrane proteins incorporated into giant unilamellar vesicles. Biophys J 88:1134–1142.
- Donaldson JG, Cassel D, Kahn RA, Klausner RD. 1992. ADP-ribosylation factor, a small GTP-binding protein, is required for binding of the coatomer protein beta-COP to Golgi membranes. Proc Natl Acad Sci USA 89:6408–6412.
- Drin G, Casella JF, Gautier R, Boehmer T, Schwartz TU, Antonny B. 2007. A general amphipathic alpha-helical motif for sensing membrane curvature. Nat Struct Mol Biol 14:138–146.
- Drin G, Morello V, Casella JF, Gounon P, Antonny B. 2008. Asymmetric tethering of flat and curved lipid membranes by a golgin. Science 320:670–673.
- Drury JL, Dembo M. 1999. Hydrodynamics of micropipette aspiration. Biophys J 76:110–128.
- Egea G, Lazaro-Dieguez F, Vilella M. 2006. Actin dynamics at the Golgi complex in mammalian cells. Curr Opin Cell Biol 18:168–178.
- Emr S, Glick BS, Linstedt AD, Lippincott-Schwartz J, Luini A, Malhotra V, Marsh BJ, Nakano A, Pfeffer SR, Rabouille C, Rothman JE, Warren G, Wieland FT. 2009. Journeys through the Golgi – taking stock in a new era. J Cell Biol 187:449–453.
- Evans E, Rawicz W. 1990. Entropy-driven tension and bending elasticity in condensed-fluid membranes. Phys Rev Lett 64:2094–2097.
- Evans EA. 1973a. A new material concept for the red cell membrane. Biophys J 13:926–940.
- Evans EA. 1973b. New membrane concept applied to the analysis of fluid shear- and micropipette-deformed red blood cells. Biophys J 13:941–954.
- Evans EA. 1974. Bending resistance and chemically induced moments in membrane bilayers. Biophys J 14:923–931.
- Ewers H, Romer W, Smith AE, Bacia K, Dmitrieff S, Chai W, Mancini R, Kartenbeck J, Chambon V, Berland L, Oppenheim A, Schwarzmann G, Feizi T, Schwille P, Sens P, Helenius A, Johannes L. 2010. GM1 structure determines SV40-induced membrane invagination and infection. Nat Cell Biol 12:11–18; (Suppl.):1–12.
- Farsad K, De Camilli P. 2003. Mechanisms of membrane deformation. Curr Opin Cell Biol 15:372–381.
- Fath S, Mancias JD, Bi X, Goldberg J. 2007. Structure and organization of coat proteins in the COPII cage. Cell 129:1325–1336.
- Fernandez-Ulibarri I, Vilella M, Lazaro-Dieguez F, Sarri E, Martinez SE, Jimenez N, Claro E, Merida I, Burger KN, Egea G. 2007. Diacylglycerol is required for the formation of COPI vesicles in the Golgi-to-ER transport pathway. Mol Biol Cell 18:3250–3263.
- Fotin A, Cheng Y, Sliz P, Grigorieff N, Harrison SC, Kirchhausen T, Walz T. 2004. Molecular model for a complete clathrin lattice from electron cryomicroscopy. Nature 432:573–579.
- Gallop JL, Butler PJ, McMahon HT. 2005. Endophilin and CtBP/BARS are not acyl transferases in endocytosis or Golgi fission. Nature 438:675–678.
- Girard P, Pecreaux J, Lenoir G, Falson P, Rigaud JL, Bassereau P. 2004. A new method for the reconstitution of membrane proteins into giant unilamellar vesicles. Biophys J 87:419–429.
- Glick BS, Nakano A. 2009. Membrane traffic within the Golgi apparatus. Annu Rev Cell Dev Biol 25:113–1132.
- Harrison SC, Kirchhausen T. 1983. Clathrin, cages, and coated vesicles. Cell 33:650–652.
- Heinrich M, Tian A, Esposito C, Baumgart T. 2010. Dynamic sorting of lipids and proteins in membrane tubes with a moving phase boundary. Proc Natl Acad Sci USA 107:7208–7213.
- Helfrich W. 1973. Elastic properties of lipid bilayers: Theory and possible experiments. Z Naturforsch [C] 28:693–703.
- Henne WM, Boucrot E, Meinecke M, Evergren E, Vallis Y, Mittal R, McMahon HT. 2010. FCHo proteins are nucleators of clathrin-mediated endocytosis. Science 328:1281–1284.
- Heuvingh J, Franco M, Chavrier P, Sykes C. 2007. ARF1-mediated actin polymerization produces movement of artificial vesicles. Proc Natl Acad Sci USA 104:16928–16933.
- Holthuis JC, Levine TP. 2005. Lipid traffic: Floppy drives and a superhighway. Nat Rev Mol Cell Biol 6:209–220.
- Hsia KC, Hoelz A. 2010. Crystal structure of alpha-COP in complex with epsilon-COP provides insight into the architecture of the COPI vesicular coat. Proc Natl Acad Sci USA 107:11271–11276.
- Hsu VW, Yang JS. 2009. Mechanisms of COPI vesicle formation. FEBS Lett 583:3758–3763.
- Inoue H, Randazzo PA. 2007. Arf GAPs and their interacting proteins. Traffic 8:1465–1475.
- Itoh T, Erdmann KS, Roux A, Habermann B, Werner H, De Camilli P. 2005. Dynamin and the actin cytoskeleton cooperatively regulate plasma membrane invagination by BAR and F-BAR proteins. Dev Cell 9:791–804.
- Jackson CL. 2009. Mechanisms of transport through the Golgi complex. J Cell Sci 122:443–452.
- Julicher F, Lipowsky R. 1993. Domain-induced budding of vesicles. Phys Rev Lett 70:2964–2967.
- Kahya N. 2010. Protein-protein and protein-lipid interactions in domain-assembly: Lessons from giant unilamellar vesicles. Biochim Biophys Acta 1798:1392–1398.
- Kirchhausen T. 2009. Imaging endocytic clathrin structures in living cells. Trends Cell Biol 19:596–605.
- Kliouchnikov L, Bigay J, Mesmin B, Parnis A, Rawet M, Goldfeder N, Antonny B, Cassel D. 2009. Discrete determinants in ArfGAP2/3 conferring Golgi localization and regulation by the COPI coat. Mol Biol Cell 20:859–869.
- Koster G, VanDuijn M, Hofs B, Dogterom M. 2003. Membrane tube formation from giant vesicles by dynamic association of motor proteins. Proc Natl Acad Sci USA 100:15583–15588.
- Krauss M, Jia JY, Roux A, Beck R, Wieland FT, De Camilli P, Haucke V. 2008. Arf1-GTP-induced tubule formation suggests a function of Arf family proteins in curvature acquisition at sites of vesicle budding. J Biol Chem 283:27717–27723.
- Kwok R, Evans E. 1981. Thermoelasticity of large lecithin bilayer vesicles. Biophys J 35:637–652.
- Lee C, Goldberg J. 2010. Structure of coatomer cage proteins and the relationship among COPI, COPII, and clathrin vesicle coats. Cell 142:123–132.
- Lee MC, Miller EA, Goldberg J, Orci L, Schekman R. 2004. Bi-directional protein transport between the ER and Golgi. Annu Rev Cell Dev Biol 20:87–123.
- Lee MC, Orci L, Hamamoto S, Futai E, Ravazzola M, Schekman R. 2005. Sar1p N-terminal helix initiates membrane curvature and completes the fission of a COPII vesicle. Cell 122:605–617.
- Leventis PA, Grinstein S. 2010. The distribution and function of phosphatidylserine in cellular membranes. Annu Rev Biophys 39:407–427.
- Lingwood D, Ries J, Schwille P, Simons K. 2008. Plasma membranes are poised for activation of raft phase coalescence at physiological temperature. Proc Natl Acad Sci USA 105:10005–10010.
- Lingwood D, Simons K. 2010. Lipid rafts as a membrane-organizing principle. Science 327:46–50.
- Liu AP, Fletcher DA. 2006. Actin polymerization serves as a membrane domain switch in model lipid bilayers. Biophys J 91:4064–4070.
- Liu J, Kaksonen M, Drubin DG, Oster G. 2006. Endocytic vesicle scission by lipid phase boundary forces. Proc Natl Acad Sci USA 103:10277–10282.
- Long KR, Yamamoto Y, Baker AL, Watkins SC, Coyne CB, Conway JF, Aridor M. 2010. Sar1 assembly regulates membrane constriction and ER export. J Cell Biol 190:115–128.
- Lundmark R, Doherty GJ, Vallis Y, Peter BJ, McMahon HT. 2008. Arf family GTP loading is activated by, and generates, positive membrane curvature. Biochem J 414:189–194.
- Luo R, Ha VL, Hayashi R, Randazzo PA. 2009. Arf GAP2 is positively regulated by coatomer and cargo. Cell Signal 21:1169–1179.
- Malhotra V, Serafini T, Orci L, Shepherd JC, Rothman JE. 1989. Purification of a novel class of coated vesicles mediating biosynthetic protein transport through the Golgi stack. Cell 58:329–336.
- Manneville JB, Casella JF, Ambroggio E, Gounon P, Bertherat J, Bassereau P, Cartaud J, Antonny B, Goud B. 2008. COPI coat assembly occurs on liquid-disordered domains and the associated membrane deformations are limited by membrane tension. Proc Natl Acad Sci USA 105:16946–16951.
- Matas OB, Martinez-Menarguez JA, Egea G. 2004. Association of Cdc42/N-WASP/Arp2/3 signaling pathway with Golgi membranes. Traffic 5:838–846.
- McMahon HT, Gallop JL. 2005. Membrane curvature and mechanisms of dynamic cell membrane remodelling. Nature 438:590–596.
- Meleard P, Bagatolli LA, Pott T. 2009. Giant unilamellar vesicle electroformation from lipid mixtures to native membranes under physiological conditions. Methods Enzymol 465:161–176.
- Miserey-Lenkei S, Chalancon G, Bardin S, Formstecher E, Goud B, Echard A. 2010. Rab and actomyosin dependent fission of transport vesicles at the Golgi complex. Nat Cell Biol 12: 645-654.
- Montes LR, Ahyayauch H, Ibarguren M, Sot J, Alonso A, Bagatolli LA, Goni FM. 2010. Electroformation of giant unilamellar vesicles from native membranes and organic lipid mixtures for the study of lipid domains under physiological ionic-strength conditions. Methods Mol Biol 606:105–114.
- Montes LR, Alonso A, Goni FM, Bagatolli LA. 2007. Giant unilamellar vesicles electroformed from native membranes and organic lipid mixtures under physiological conditions. Biophys J 93:3548–3554.
- Morales-Penningston NF, Wu J, Farkas ER, Goh SL, Konyakhina TM, Zheng JY, Webb WW, Feigenson GW. 2010. GUV preparation and imaging: Minimizing artifacts. Biochim Biophys Acta 1798:1324–1332.
- Nakagawa T, Setou M, Seog D, Ogasawara K, Dohmae N, Takio K, Hirokawa N. 2000. A novel motor, KIF 13A, transports mannose-6-phosphate receptor to plasma membrane through direct interaction with AP-1 complex. Cell 103:569–581.
- Nickel W, Wieland FT. 2001. Receptor-dependent formation of COPI-coated vesicles from chemically defined donor liposomes. Methods Enzymol 329:388–404.
- Oprins A, Duden R, Kreis TE, Geuze HJ, Slot JW. 1993. Beta-COP localizes mainly to the cis-Golgi side in exocrine pancreas. J Cell Biol 121:49–59.
- Orci L, Glick BS, Rothman JE. 1986. A new type of coated vesicular carrier that appears not to contain clathrin: Its possible role in protein transport within the Golgi stack. Cell 46:171–184.
- Orci L, Palmer DJ, Amherdt M, Rothman JE. 1993a. Coated vesicle assembly in the Golgi requires only coatomer and ARF proteins from the cytosol. Nature 364:732–734.
- Orci L, Palmer DJ, Ravazzola M, Perrelet A, Amherdt M, Rothman JE. 1993b. Budding from Golgi membranes requires the coatomer complex of non-clathrin coat proteins. Nature 362:648–652.
- Ostermann J, Orci L, Tani K, Amherdt M, Ravazzola M, Elazar Z, Rothman JE. 1993. Stepwise assembly of functionally active transport vesicles. Cell 75:1015–1025.
- Parthasarathy R, Groves JT. 2007. Curvature and spatial organization in biological membranes. Soft Matter 3:24–33.
- Patterson GH, Hirschberg K, Polishchuk RS, Gerlich D, Phair RD, Lippincott-Schwartz J. 2008. Transport through the Golgi apparatus by rapid partitioning within a two-phase membrane system. Cell 133:1055–1067.
- Pautot S, Frisken BJ, Weitz DA. 2003. Engineering asymmetric vesicles. Proc Natl Acad Sci USA 100:10718–10721.
- Pucadyil TJ, Schmid SL. 2008. Real-time visualization of dynamin-catalyzed membrane fission and vesicle release. Cell 135:1263–1275.
- Qin D, Xia Y, Whitesides GM. 2010. Soft lithography for micro- and nanoscale patterning. Nat Protoc 5:491–502.
- Reinhard C, Schweikert M, Wieland FT, Nickel W. 2003. Functional reconstitution of COPI coat assembly and disassembly using chemically defined components. Proc Natl Acad Sci USA 100:8253–8257.
- Ren Y, Yip CK, Tripathi A, Huie D, Jeffrey PD, Walz T, Hughson FM. 2009. A structure-based mechanism for vesicle capture by the multisubunit tethering complex Dsl1. Cell 139:1119–1129.
- Romer W, Berland L, Chambon V, Gaus K, Windschiegl B, Tenza D, Aly MR, Fraisier V, Florent JC, Perrais D, Lamaze C, Raposo G, Steinem C, Sens P, Bassereau P, Johannes L. 2007. Shiga toxin induces tubular membrane invaginations for its uptake into cells. Nature 450:670–675.
- Romer W, Pontani LL, Sorre B, Rentero C, Berland L, Chambon V, Lamaze C, Bassereau P, Sykes C, Gaus K, Johannes L. 2010. Actin dynamics drive membrane reorganization and scission in clathrin-independent endocytosis. Cell 140:540–553.
- Roux A, Cappello G, Cartaud J, Prost J, Goud B, Bassereau P. 2002. A minimal system allowing tubulation with molecular motors pulling on giant liposomes. Proc Natl Acad Sci USA 99:5394–5399.
- Roux A, Cuvelier D, Nassoy P, Prost J, Bassereau P, Goud B. 2005. Role of curvature and phase transition in lipid sorting and fission of membrane tubules. Embo J 24:1537–1545.
- Roux A, Koster G, Lenz M, Sorre B, Manneville J-B, Nassoy P, Bassereau P. 2010. Membrane curvature controls dynamin polymerization. Proc Natl Acad Sci USA 107:4141–4146.
- Roux A, Uyhazi K, Frost A, De Camilli P. 2006. GTP-dependent twisting of dynamin implicates constriction and tension in membrane fission. Nature 441:528–531.
- Sengupta P, Hammond A, Holowka D, Baird B. 2008. Structural determinants for partitioning of lipids and proteins between coexisting fluid phases in giant plasma membrane vesicles. Biochim Biophys Acta 1778:20–32.
- Sens P, Johannes L, Bassereau P. 2008. Biophysical approaches to protein-induced membrane deformations in trafficking. Curr Opin Cell Biol 20:476–482.
- Sheetz MP, Singer SJ. 1974. Biological membranes as bilayer couples. A molecular mechanism of drug-erythrocyte interactions. Proc Natl Acad Sci USA 71:4457–4461.
- Short B, Haas A, Barr FA. 2005. Golgins and GTPases, giving identity and structure to the Golgi apparatus. Biochim Biophys Acta 1744:383–395.
- Sorre B, Callan-Jones A, Manneville JB, Nassoy P, Joanny JF, Prost J, Goud B, Bassereau P. 2009. Curvature-driven lipid sorting needs proximity to a demixing point and is aided by proteins. Proc Natl Acad Sci USA 106:5622–5626.
- Spang A. 2008. The life cycle of a transport vesicle. Cell Mol Life Sci 65:2781–2789.
- Spang A. 2009. On vesicle formation and tethering in the ER-Golgi shuttle. Curr Opin Cell Biol 21:531–536.
- Spang A, Matsuoka K, Hamamoto S, Schekman R, Orci L. 1998. Coatomer, Arf1p, and nucleotide are required to bud coat protein complex I-coated vesicles from large synthetic liposomes. Proc Natl Acad Sci USA 95:11199–11204.
- Stagg SM, Gurkan C, Fowler DM, LaPointe P, Foss TR, Potter CS, Carragher B, Balch WE. 2006. Structure of the Sec13/31 COPII coat cage. Nature 439:234–238.
- Tanigawa G, Orci L, Amherdt M, Ravazzola M, Helms JB, Rothman JE. 1993. Hydrolysis of bound GTP by ARF protein triggers uncoating of Golgi-derived COP-coated vesicles. J Cell Biol 123:1365–1371.
- Tian A, Baumgart T. 2009. Sorting of lipids and proteins in membrane curvature gradients. Biophys J 96:2676–2688.
- Ungewickell EJ, Hinrichsen L. 2007. Endocytosis: Clathrin-mediated membrane budding. Curr Opin Cell Biol. 19:417–425.
- Upadhyaya A, Sheetz MP. 2004. Tension in tubulovesicular networks of Golgi and endoplasmic reticulum membranes. Biophys J 86:2923–2928.
- van den Brink-van der Laan E, Killian JA, de Kruijff B. 2004. Nonbilayer lipids affect peripheral and integral membrane proteins via changes in the lateral pressure profile. Biochim Biophys Acta 1666:275–288.
- van Meer G. 1998. Lipids of the Golgi membrane. Trends Cell Biol 8:29–33.
- van Meer G, Voelker DR, Feigenson GW. 2008. Membrane lipids: Where they are and how they behave. Nat Rev Mol Cell Biol 9:112–124.
- Varnier A, Kermarrec F, Blesneac I, Moreau C, Liguori L, Lenormand JL, Picollet-D'hahan N. 2010. A simple method for the reconstitution of membrane proteins into giant unilamellar vesicles. J Membr Biol 233:85–92.
- Veatch SL, Cicuta P, Sengupta P, Honerkamp-Smith A, Holowka D, Baird B. 2008. Critical fluctuations in plasma membrane vesicles. ACS Chem Biol 3:287–293.
- Walde P, Cosentino K, Engel H, Stano P. 2010. Giant vesicles: Preparations and applications. Chembiochemistry 11:848–865.
- Watson P, Forster R, Palmer KJ, Pepperkok R, Stephens DJ. 2005. Coupling of ER exit to microtubules through direct interaction of COPII with dynactin. Nat Cell Biol 7:48–55.
- Weidman P, Roth R, Heuser J. 1993. Golgi membrane dynamics imaged by freeze-etch electron microscopy: Views of different membrane coatings involved in tubulation versus vesiculation. Cell 75:123–133.
- Weigert R, Siletta MG, Spano S, Turacchio G, Cericola A, Colanzi A, Senatore S, Mancici R, Polishchuk EV, Salmona M, Facchiano F, Burger KN, Mironov A, Luini A, Corda D. 1999. CtBP/BARS induces fission of Golgi membranes by acylating lysophosphatidic acid. Nature 402:429–433.
- Weimer C, Beck R, Eckert P, Reckmann I, Moelleken J, Brugger B, Wieland F. 2008. Differential roles of ArfGAP1, ArfGAP2, and ArfGAP3 in COPI trafficking. J Cell Biol 183:725–735.
- Wollert T, Hurley JH. 2010. Molecular mechanism of multivesicular body biogenesis by ESCRT complexes. Nature 464:864–869.
- Wollert T, Wunder C, Lippincott-Schwartz J, Hurley JH. 2009. Membrane scission by the ESCRT-III complex. Nature 458:172–177.
- Wu WJ, Erickson JW, Lin R, Cerione RA. 2000. The gamma-subunit of the coatomer complex binds Cdc42 to mediate transformation. Nature 405:800–804.
- Yang JS, Gad H, Lee SY, Mironov A, Zhang L, Beznoussenko GV, Valente C, Turacchio G, Bonsra AN, Du G, Baldanzi G, Graziani A, Bourgoin S, Frohman MA, Luini A, Hsu VW. 2008. A role for phosphatidic acid in COPI vesicle fission yields insights into Golgi maintenance. Nat Cell Biol 10:1146–1153.
- Yang JS, Lee SY, Gao M, Bourgoin S, Randazzo PA, Premont RT, Hsu VW. 2002. ARFGAP1 promotes the formation of COPI vesicles, suggesting function as a component of the coat. J Cell Biol 159:69–78.
- Yang JS, Lee SY, Spano S, Gad H, Zhang L, Nie Z, Bonazzi M, Corda D, Luini A, Hsu VW. 2005. A role for BARS at the fission step of COPI vesicle formation from Golgi membrane. Embo J 24:4133–4143.
- Yang JS, Zhang L, Lee SY, Gad H, Luini A, Hsu VW. 2006. Key components of the fission machinery are interchangeable. Nat Cell Biol 8:1376–1382.
- Yeung T, Gilbert GE, Shi J, Silvius J, Kapus A, Grinstein S. 2008. Membrane phosphatidylserine regulates surface charge and protein localization. Science 319:210–213.
- Zink S, Wenzel D, Wurm CA, Schmitt HD. 2009. A link between ER tethering and COP-I vesicle uncoating. Dev Cell 17:403–416.