Abstract
Topical or transdermal drug delivery is challenging because the skin acts as a natural and protective barrier. Therefore, several methods have been examined to increase the permeation of therapeutic molecules into and through the skin. One approach is to use the nanoparticulate delivery system. Starting with liposomes and other vesicular systems, several other types of nanosized drug carriers have been developed such as solid lipid nanoparticles, nanostructured lipid carriers, polymer-based nanoparticles and magnetic nanoparticles for dermatological applications. This review article discusses how different particulate systems can interact and penetrate into the skin barrier. In this review, the effectiveness of nanoparticles, as well as possible mode of actions of nanoparticles, is presented. In addition to nanoparticles, cell-penetrating peptide (CPP)-mediated drug delivery into the skin and the possible mechanism of CPP-derived delivery into the skin is discussed. Lastly, the effectiveness and possible mechanism of CPP-modified nanocarriers into the skin are addressed.
Introduction: Skin as a barrier
The skin is a protective barrier against external mechanical, chemical, microbial and physical influences. Because of large surface area and easy accessibility, skin delivery has potential application in drug delivery. Skin delivery is mainly focused on topical delivery to treat local skin conditions or transdermal drug delivery, which involves the delivery of drugs through skin layers into systemic circulation. Topical or transdermal delivery offers several advantages over the conventional oral and intravenous dosage forms such as prevention of first pass metabolism, minimization of pain and possible controlled release of drugs (Teo et al. Citation2006). Although skin has large surface area, delivery of drug molecules into the skin is challenging as skin acts as a formidable barrier. Physiologically, skin is composed of four distinguishable layers: Stratum corneum (SC), viable epidermis, dermis and subcutaneous connective tissue. The SC is the outermost layer of the skin and is comprised of a 10–15 μm thick matrix of dehydrated and dead keratinocytes that are embedded in highly ordered lipid layers, which serve as a cover (Bouwstra et al. Citation2003). The SC is the foremost barrier which protects our body from the entry of external materials into the skin. The viable epidermis is approximately 100–150 μm thick and composed of multiple layers of keratinocytes and several other types of cells. The dermis contains a network of blood capillaries, lymphatic vessels and nerve endings. The subcutaneous tissue or hypodermis resides below the dermis and is composed of loose textured, white, fibrous connective tissue in which fat and elastic fibers are intermingled (Kanikkannan et al. Citation2000). In addition to four main layers, several pilosebaceous units and sweat glands are dispersed throughout the skin.
The micro and macromolecules can enter into the skin through three distinct pathways: (1) The intercellular pathway, which is through the lipid matrix occupying the intercellular spaces of the keratinocytes, (2) the transcellular pathway, which is through the keratinocytes, and (3) transappendageal pathway, which is across hair follicles, sebaceous glands and sweat glands. Earlier reported studies demonstrated that skin appendages are not a major penetration pathway as it covers only 0.1% of the skin surface area and permeation in the skin is mainly assumed to be through the lipid matrix of the SC (Hadgraft Citation2001). In contrast, recent investigations have demonstrated that the transappendageal route is an efficient penetration pathway and acts as a reservoir for topically applied substances (Lademann et al. Citation2008). Therefore, besides diffusional pathways, the transappendageal route appears to be one of the valuable pathways in skin permeation. Several attempts have been made, and are still under investigation, to develop efficient formulations to deliver micro and macromolecules into the skin or into systemic circulation. Substances smaller than 500 kDa, with sufficient oil solubility and high partition coefficient, can be absorbed into the skin. However, in contrast, larger molecules (molecular weight > 500 kDa) cannot pass the cutaneous barrier (Bos and Meinardi Citation2000).
One of the most important and extensively investigated strategies to enhance the drug delivery through skin is the introduction of structural alterations within the skin by addition of chemical enhancers such as fatty acids, surfactants, esters, alcohols, polyalcohols, pyrrolidones, amines, amides, sulphoxides, terpenes, alkanes and phospholipids (Kanikkannan et al. Citation2000, Naik et al. Citation2000). Chemical enhancers work through different mechanisms, which include: (a) Perturbation of the highly ordered structure of SC, (b) solubilization and extraction of keratin and/or lipid components of the SC, (c) fluidization of the crystalline structure of SC and dissolution of SC lipids, (d) simple hydration achieved by skin occlusion, and (e) improved partitioning of a drug, co-enhancer or solvent into SC (Kanikkannan et al. Citation2000, Naik et al. Citation2000, Kumar and Philip Citation2007). Nevertheless, the use of chemical enhancers is restricted because of the limited permeability of macromolecules and because the amount of chemical enhancer required to achieve pharmacologically effective drug concentration is often beyond the capability tolerated by skin (Kumar and Philip Citation2007). Microneedles, jet injectors, iontophoresis, ultrasound, electroporation, photomechanical waves, magnetophoresis, laser radiation and skin abrasions are some of the main mechanical, physical and active transport techniques available to enhance skin penetration of various drugs (Kumar and Philip Citation2007). Microneedle-based delivery has some disadvantages such as: (a) The needles are very small and thinner than the diameter of hair so the microneedle tip can be broken off and left under the skin, which can cause local inflammation, and (b) proper application is needed and self-administration is not easy. The efficiency of the most evolved and widely studied method, iontophoresis, is dependent on several factors such as polarity, valence, ionic mobility and composition of the formulation (Naik et al. Citation2000). Methods used for delivery of skin impermeable molecules, microneedles or electroporation, are invasive in nature and could damage the skin barrier properties. To overcome the limitations of chemical and physical enhancers, micro and nanoparticles are being developed which do not only enhance the absorption of drugs into the skin but also release the drug in a controlled manner for a prolonged period of time.
Nanoparticles as efficient carriers for cutaneous drug delivery
In recent years, the number of products containing nanosized material has increased because nanoparticles exhibit unique size-dependent physical and chemical properties that can be advantageous in delivery of drugs to the skin. Nanoparticles can be composed of lipids, sugars, degradable or non-degradable polymers, metals and organic or inorganic compounds. Nanoparticles have several advantages over chemical penetration enhancers such as sustained drug release for a prolonged period of time and protection of encapsulated materials from chemical degradation. For optimum topical delivery of drugs into the skin, it is essential that the carrier releases the encapsulated drug, which can be further absorbed through the skin layers and through sub skin structures that are involved in disease (de Leeuw et al. Citation2009).
To develop optimized nanoparticle formulation for skin delivery, there is a need to understand interactions of nanoparticles with the skin and their transport mechanisms into the skin. Following topical application of nanoparticle formulations, absorption of active compounds can follow transcellular, intercellular or transappendageal pathway (). Nanocarriers can translocate intact into the skin without being degraded or they can be degraded near the skin surface and the incorporated therapeutic molecule can penetrate into skin layers. Interaction of nanoparticles with skin depends on the physicochemical properties of nanoparticles such as size, surface charge, properties of nanomaterial used, drug-loading efficiency, lamellarity and mode of application. Nonetheless, there is convincing evidence that irrespective of nanomaterial used, most particles do not cross the SC and transappendageal route appears to be the dominant pathway of nanoparticle entry into skin.
Figure 1. Model for topical delivery of CPP-coated NLC delivery approach. (I) Structures of NLC with and without surface modifications. (a) Structure of NLC; (b) Structure of CPP-coated NLC (NLC-CPP); (c) Structure of non-transduction peptide, YKA, coated NLC (NLC-YKA). (II) Various pathways for the entry of nanoparticles into the skin. (a) This panel shows that due to occlusive properties, NLC particles entering into the stratum corneum via the paracellular route are unable to cross SC; (b) Surface modification of NLC particles with CPP favours permeation of NLC into the SC and subsequently into the epidermis; (c) YKA coating on NLC prevents entry of nanoparticles into the skin, (d) NLC and NLC-CPP enter the skin via hair follicle route. (III) An expanded view of the SC and NLC penetration mechanisms (see legend to , part II for description).
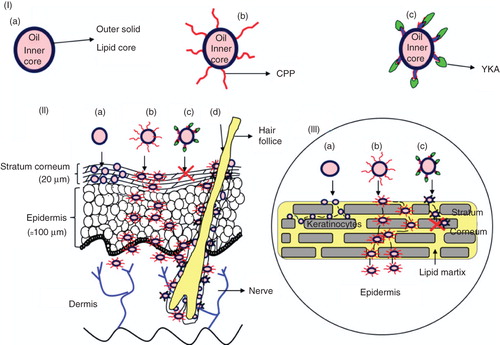
Lipid vesicles
Liposomes were the first particulate systems to be studied as an effective delivery system for the skin (Honeywell-Nguyen and Bouwstra Citation2005). Liposomes are lipid vesicles mainly composed of one or multiple lipid bilayers composed of mixtures of phosphatidylcholines with long or short hydrocarbon chains. They have high morphological diversity as a function of hydration, temperature and composition (Barbosa-Barros et al. Citation2009). In addition, transferosomes, niosomes, ethosomes and bicelles have also been developed to enhance the skin permeation of various molecules (Elsayed et al. Citation2007).
Transfersomes® are made up of phospholipids supplemented with single chain surfactant with a high radius of curvature which acts as edge activators to provide vesicle elasticity and deformability (Cevc et al. Citation1996). Ethosomes contains phospholipids, ethanol and water. Ethosomes are soft and malleable novel lipid carriers tailored for enhanced skin delivery (Touitou et al. Citation2000). Bicelles are recently studied spherical micelles and discoidal micelles, which are two-dimensional network of branched flattened cylindrical micelles (Barbosa-Barros et al. Citation2009).
Mechanism of lipid vesicles skin permeation
Liposomes can enter into the skin by several mechanisms (El Maghraby et al. Citation2008, de Leeuw et al. Citation2009). These include:
(a) Intact vesicular skin penetration;
(b) Penetration enhancing effect by trans-epidermal osmotic gradient and hydration force;
(c) Lateral diffusion of vesicles in the SC; and
(d) Transappendageal pathway.
Early reports suggested that the intact vesicles can reach dermal layers (Mezei and Gulasekharam Citation1980, Citation1982). However, contrary to early findings, Kirjavainen et al. (Citation1996) reported that the improved delivery was due to the penetration enhancement effect caused by changes in the structure of vesicle lipids. After occlusion, no penetration of liposomes occurs which supports the fact that liposomes can form trans-epidermal osmotic gradient and can penetrate into epidermis by hydration force (de Leeuw et al. Citation2009). Furthermore, penetration can also be due to adhesion of vesicle lipids to the skin surface followed by destabilization and finally fusion with the SC lipid matrix. Lipid exchange occurs upon fusion between human membrane and liposomes via molecular diffusion. Liposomes with particle size up to 0.60 μm diameter can penetrate the skin but those more than 1.0 μm in size could not penetrate the skin, suggesting that pilosebaceous unit may also play a role in the permeation (Schramlová et al. Citation1997).
The skin permeation of liposomes is dependent on lipid composition, size and surface charge. The positive charge of liposomes is helpful for binding in an ion-exchange manner with the negatively charged skin cells and hair follicles (Jung et al. Citation2006). Shanmugam et al. (Citation2009) showed that the steady state flux of drug through the skin is higher for cationic liposomes, followed by anionic and neutral liposomes. The enhanced skin permeation of cationic liposomes is attributed to the permeation selectivity of skin, which is known as ‘Donnan exclusion effect’ (Shanmugam et al. Citation2009). Human skin SC is made up of lipids such as ceramides, cholesterol, free fatty acids and cholesteryl sulfate. Ceramides are hydrophobic sphingolipids and account for approximately 50% of lipid weight in SC. Ceramide-based liposomes with high membrane fluidity and high fusion activity to SC lipids can deliver larger amounts of the active ingredient into the skin and also these liposomes can serve as a reservoirs in SC (Tokudome et al. Citation2009). The concentrations of lipids used to formulate liposomes can also affect the skin permeation. Liposomes made from more concentrated lipids have a less efficient penetration profile, while liposomes made from less concentrated lipids have a better penetration profile and can penetrate up to the epidermal-dermal junction (Carrer et al. Citation2008).
With regards to entering into the skin, either deformable vesicles carry drugs into the SC or vesicles act as penetration enhancers by modifying the intercellular lamellae of SC (Elsayed et al. Citation2007). An important difference between liposomes and transferosomes® is that the high stress dependent adaptability of such deformable liposomes allows them to squeeze between the cells of SC (Honeywell-Nguyen and Bouwstra Citation2005). The deformable non-ionic surfactant vesicles, when tested on human volunteers, partitioned deep into SC layers (Honeywell-Nguyen et al. Citation2002, Citation2003). This distribution of vesicles and drug suggests that the drug remains in association with the vesicular material in the middle and upper layers of SC but deformation occurs in the lowest layer. This implies that deformable non-ionic surfactant vesicles mainly remain in the SC, and from there drug molecules are released and enter in the viable layers of skin (Honeywell-Nguyen et al. Citation2004). Moreover, deformable liposomes were shown to carry both entrapped and attached hydrophilic fluorescent dye into the deeper layers of skin, suggesting the penetration enhancing effects (Verma et al. Citation2003). The deformable liposomes also bear the potential to follow the transappendageal route for the skin penetration (El Maghraby et al. Citation2001). Ethosomes can deliver most classes of drugs successfully into the skin but the exact mode of action of these vehicles remains unclear. Although the enhanced drug delivery in skin is much higher than expected from ethanol alone, synergistic effects were suggested between ethanol, vesicles and skin lipids (Touitou et al. Citation2000). The interaction of ethanol with the polar heads can increase membrane permeability of these vesicles. In addition to all these lipid vesicles, the penetration of bicellar systems through the narrow intercellular space of SC allows lipids from bicelles to interact with lipids of SC, and this can induce modification of the barrier function. Barbosa-Barros et al. (Citation2009) have recently demonstrated that gel-to-liquid phase transition temperature is one of the important factors to consider. When lipids undergo phase transition from gel-to-liquid crystalline, they can mix and modify the lateral organization of the SC lipids. However, when the bicellar system remains in the gel state, the gel state can minimize the mixing of bicellar lipids with SC and cannot modify the barrier function. This allows the transformation from the discoidal structures of bicelles to vesicular structure inside the SC by skin hydration. Hence, selection of an appropriate composition of lipids is a key factor for effective lipid nanostructures.
Lipid nanoparticles (SLN and NLC)
Besides lipid vesicles, solid lipid nanoparticles (SLNs) and nanostructure lipid carriers (NLCs) have been developed to increase the physicochemical stability of both incorporated drug molecules and particulate system for cosmetics and dermatological use. SLNs are made up of lipids that are solid at room temperature. The surface of SLN is covered by a surfactant shell which stabilizes the dispersion (Lucks and Müller Citation1991). When compared to liposomes and emulsions, SLNs have advantages such as modulated release capacity of the active compound and increased skin hydration. Nonetheless, SLN suffer from the drawbacks of uncontrolled drug drug expulsion from the carrier and limited drug loading capacity. To overcome these limitations, a second generation of lipid nanoparticles, NLCs, has been developed (Mehnert and Mäder Citation2001). NLCs compose of the mixture of solid lipids and liquid lipids (oils), in which the liquid phase is embedded into a solid lipid matrix or localized at the surface of the solid platelets with the surfactant layer (Patlolla et al. Citation2010a). NLCs have a higher loading capacity and lower drug leaching upon storage ascompared to SLNs (Mehnert and Mäder Citation2001). The SLN and NLC nanoparticles are widely used for topical drug delivery because they protect the encapsulated active molecules from enzymatic degradation, prevent trans-epidermal water loss and release the drugs in a controlled manner for prolonged periods, which in turn enhances the therapeutic effect of the active agent.
SLNs and NLCs for the skin topical application are made from lipids such as glycerol behenate (Compritol® 888 ATO), glycerol palmitostearate (Precirol® ATO 5) or wax cetyl palmitate. In preparation of NLC, liquid lipid such as medium chain triglycerides (Miglyol® 812) is added (Schäfer-Korting et al. Citation2007). Generally 0.5–5% surfactant is added for physical stabilization of the nanoparticles. These nanocarriers have very low toxicity as the materials used in SLN and NLC preparation are biocompatible and biodegradable (Patlolla et al. Citation2010a).
Mechanisms of lipid nanoparticle skin permeation
SLN and NLC nanoparticles show adhesiveness, occlusion and skin hydration effects when applied topically on skin. SLN or NLC show adhesiveness by forming a monolayer on the skin when the particle size is less than 200 nm. Since this monolayer film is hydrophobic, it shows occlusive action on the skin and retards the loss of moisture as a result of evaporation, which can result in reduction of corneocyte packing and opening of inter-corneocyte gaps and thus facilitates the drug penetration into deeper layers of skin (Wissing and Müller Citation2003). The loss of water content from the SLN induces crystal modification of SLN matrix and this can induce drug expulsion and penetration (Lombardi Borgia et al. Citation2005). The occlusion effect of these nanoparticles is dependent on the applied sample volume, particle size, crystallinity and lipid concentration (Teeranachaideekul et al. Citation2008). Small size particles show higher barrier properties for evaporation and increase the occlusion, whereas with bigger size particles, more of the water is evaporated (Souto and Müller Citation2008). The intact rigid particles are not considered to cross SC but the extremely fluid vesicles pass in an intact state (Cevc Citation2004). The particulate system tends to follow the transappendageal pathway as high lipophilicity promotes follicular deposition.
SLNs that are made up of compritol have a platelet-like shape, which allows them to slide in between the superficial corneocytes by intense contact between skin lipids. The changes in lipid particles occur over the time when the particles are in contact with the skin surface (Küchler et al. Citation2009). Küchler et al. (Citation2010) recently showed that the interaction rate of the SLNs with the skin lipids and sebum is dependent on the lipophilicity of the incorporated molecule, formulation type (drug is incorporated into lipid matrix or it is on the nanoparticle surface) and type of interacting skin lipid. The highly lipophilic molecules can penetrate easily, while no diffusion occurs when a hydrophilic molecule is used. The release profile of the incorporated drug from the NLCs varies with the solid lipid to liquid lipid composition. Teeranachaideekul et al. (Citation2008) studied the effect of the oil content in the NLC on the skin permeation and observed that the NLC with the lowest oil content can penetrate with high intensity up to the upper dermal layer. In contrast, as the oil content in the NLC increases, the penetration decreases to only the upper viable epidermis).
Polymeric nanoparticles
For dermatological applications, polymeric nanoparticles can be prepared from natural and synthetic polymers. Chitosan is a widely studied natural polymer for nanoparticulate-based delivery. Chitosan is a natural biocompatible cationic polysaccharide extracted from crustacean shells and capable of efficient drug and gene delivery (Panos et al. Citation2008). Chitosan has been found to have many beneficial effects like anticoagulant activity (Huang et al. Citation2007), wound-healing and anti-microbial properties (Rossi et al. Citation2007, Kong et al. Citation2008). Apart from natural polymers, synthetic polymers are widely used in nanoparticle formulations. The widely used synthetic polymers in skin delivery are polyalkylcyanoacylates, poly-lactic acid (PLA), poly-ϵ-caprolactone (PCL), poly-glycolic acid (PGA) or their co-polymers as poly-lactic-co-glycolic acid (PLGA) (Alvarez-Román et al. Citation2004a, 2004b, Rancan et al. Citation2009). These polymers have excellent biocompatibility and degrade through the natural pathways. PCL nanoparticles containing sunscreen agents were able to permeate only into SC (Alvarez-Román et al. Citation2004a). PLGA nanoparticles can also successfully deliver encapsulated drug into the skin (Luengo et al. Citation2006).
Mechanism of polymeric nanoparticles skin permeation
The intact polymeric nanoparticles are only able to penetrate into the superficial layers of the SC and from there the encapsulated drug will be released into the deeper skin layers. In addition to this, polymeric nanoparticles can accumulate in the hair follicles and create high local concentrations of loaded drugs which can further diffuse to the viable layers of the skin. When non-biodegradable polystyrene nanoparticles of 20 and 200 nm diameters in size were evaluated topically, the 20 nm nanoparticles accumulated in the deeper hair follicular region, while 200 nm particles showed follicular penetration in a time dependent manner (Alvarez-Román et al. Citation2004b). By using multiphoton laser scanning microscopy, Stracke et al. (Citation2006) observed that intact PLGA nanoparticles do not permeate the SC. Similar findings were reported by Luengo et al. (Citation2006), where flufenamic acid-loaded PLGA nanoparticles were unable to permeate into viable skin layers. PLA nanoparticles can penetrate into 50% of the vellus hair follicles and from there it reaches the maximum depth and enters into 12–15% of the sebaceous glands (Rancan et al. Citation2009). PLA nanoparticles destabilize on the surface of skin and in follicular ducts. This deformation leads to cluster formation and aggregation that can further release the incorporated dye (Rancan et al. Citation2009). The released dye can diffuse into sebum and penetrate into viable epidermis and dermis. Therefore, it is clear that rigid polymeric nanoparticles do not permeate the entire SC in intact form, but they rather destabilize on the skin surface and permeate into the hair follicles and then release the incorporated drug.
Magnetic nanoparticles
The magnetic and/or metallic nanoparticles are smaller in size; this can be beneficial for aspects of skin targeting such as cell labeling or cell targeting, and can be helpful in early diagnosis of skin diseases (Baroli et al. Citation2007). Magnetic nanoparticles made up of iron derivatives (magnetic, paramagnetic or superparamagnetic) have potential application in skin delivery. Baroli et al. (Citation2007) showed that the rigid magnetic nanoparticles smaller than 10 nm can passively penetrate the skin through the SC lipid matrix and hair follicle orifices, and can reach up to the stratum granulosum. Further, they observed nanoparticle aggregates in SC and in the uppermost layers of epidermis. This can be explained by the tight junctions between the stratum granulosum and the stratum lucidum, non-degraded corneodesmosomes between corneocytes, and SC layers close to viable epidermal layers that are more compact in nature. In addition, nanoparticle aggregates are found below the SC-epidermis junction, which can be explained by the penetration through the hair infundibulum and through furrows.
Quantum dots
Recently, quantum dot (QD) nanoparticles have received attention with regards to interacting with skin. Due to their quantum confinement effects, QD nanoparticles have unique optical and electronic properties; specifically, they emit strong and photostable fluorescence and are suitable for biomedical imaging. QDs preferentially are collected in upper layers of SC and in hair follicles (Mortensen et al. Citation2008). QDs can penetrate the skin by getting through SC intracellular lipid lamellae along the edges of differentiated corneocytes (Mortensen et al. Citation2008).
QDs penetrate the skin in a size and coating dependent manner. To study these effects of size and coating of QDs on skin penetration, Ryman-Rasmussen et al. (Citation2006) used two core/shell sizes and shapes (spherical and ellipsoid) of QDs with three types of surface coatings: neutral, anionic and cationic. Spherical shaped 4.6 nm core/shell diameter QDs with neutral and anionic coatings penetrated only up to epidermal layers, while cationic charged spherical QDs were localized in dermis within 8 h. In contrast, neutral and cationic charged ellipsoid shaped 12 nm (major axis) by 6 nm core/shell diameter QDs penetrated into the skin within 8 h but anionic charged ellipsoid QDs needed 24 h to show penetration. In another investigation using confocal imaging and transmission electron microcopy, Zhang et al. (Citation2008) showed that nail shaped, PEG-coated QDs with diameter less than 40 nm can penetrate into uppermost layers of SC lipids (intercellular space) and outer root sheath of hair follicles. QD skin penetration and toxicity depends on physicochemical properties like size, shape, chemical structure of the core/shell and surface coating, charge and pH of the applied vehicle. Zhang and Monteiro-Riviere (Citation2008) further studied the potential risk of healthcare workers with alterations in their skin exposed to QD during medical applications. When spherical and ellipsoid QDs were applied to intact, tape-stripped, abraded and flexed skin, abraded samples were the most permeable followed by tape-stripped and intact specimens. The transdermal absorption of nanoscale materials depends on the skin barrier quality and lack of epidermis, which provides access to QD penetration.
Titanium dioxide and zinc oxide-based nanoparticles
Nanomaterials like titanium dioxide (TiO2) (particle size up to several μm) and zinc oxide (ZnO) (particle size 30–200 nm) are key ingredients for many cosmetics and sunscreen nanoparticle formulations as they reflect and scatter UV light. Penetration of TiO2 nanoparticles through the skin is restricted when applied as a suspended form in a cosmetic-type emulsion. The use of oils, as a vehicle, in nanoparticle formulations may prevent partitioning of nanoparticles from oils into SC lipid layers and reduce penetration into deep SC layers. Lademann et al. (Citation1999) showed that deeper SC layers do not contain TiO2 even after repetitive application. In the case of TiO2, physicochemical parameters such as size, shape and surface characteristics do not affect its penetration pattern and TiO2 is solely deposited on the outermost surface of SC and cannot penetrate into deeper skin layers (Gamer et al. Citation2006). Cross et al. (Citation2007) have demonstrated that the epidermal penetration of zinc from the 26–30 nm ZnO nanoparticles is negligible after the topical application to human skin in vitro and no particles were observed in viable epidermis, suggesting that minimal penetration occurs at the lower SC layers. Ultrafine ZnO particles application on intact human skin remains in upper SC layers. On the other hand, by removing the SC by tape-stripping, penetration of particles into deeper dermal layers does not occur and ZnO particles remain on the surface of skin (Szikszai et al. Citation2010).
Importance of transappendageal pathway in nanoparticulate delivery
One of the main features of nanoparticles in dermatological application is their tendency to penetrate and accumulate into hair follicles. However, the role of hair follicles in penetration process is often neglected based on the fact that orifices of hair follicles occupy only approximately 0.1% of the total skin surface area (Lademann et al. Citation2008). On the other hand, nanoparticles can increase the penetration of encapsulated drug through hair follicles (Lademann et al. Citation2008). Furthermore, it was calculated that the storage time of the particle-based drug delivery systems in the hair follicles was 10 days compared to short-term storage time in the SC. The rigid hair shaft acts as a geared pump because of the zig-zag structure of cuticular layers along the hair shaft, and moves particles deeper into hair follicles. The hair follicle acts as a long term reservoir for nanoparticles because of the depletion of stored nanoparticles can occur due to penetration into deeper tissue layers or by their release with the sebum production, but both of these processes are slow (Lademann et al. Citation2007). Topically applied substances can permeate faster with high concentrations into the skin containing hair follicles as compared to skin with hair follicle blockage (Otberg et al. Citation2008). The interfollilular epidermis and epithelium of acroinfundibulum form a tight barrier but the corneocytes in lower follicular tract is incomplete as the corneocytes in this area are small and not completely differentiated (Rancan et al. Citation2009). Therefore, particulate carrying the drug can have an increased drug penetration into the viable epidermis. The particles penetrate down to different depths depending on their size. Only 40 nm nanoparticles, but not 750 or 1500 nm nanoparticles, can deliver the vaccine compounds transcutaneously into human antigen-presenting cells (Vogt et al. Citation2006). Fluorescence microscopy and laser scanning microscopy showed that transcutaneously applied 40 nm nanoparticles penetrate through follicular pathway into the perifollicular dermis and therefore cross through the meshwork of follicular openings. The bigger nanoparticles of size 700 nm and 1500 nm aggregate superficially in follicular openings. Targeting several sites in the hair follicle such as the entry level of sebaceous gland or the bulge region can be achieved by the size dependent mechanism of nanoparticles (Toll et al. Citation2004). Not all hair follicles contain applied nanoparticles as some hair follicles remain closed and some remain open for topically applied nanoparticles (Lademann et al. Citation2008). The fluorescent dye can penetrate only into the open hair follicle which shows the activity such as sebum excretion or hair growth. Depending on the skin samples, approximately 50–70% of hair follicles are open for penetration (Lademann et al. Citation2001). Further massage can increase the penetration into hair follicle (Toll et al. Citation2004). Nanoparticles penetrating into hair follicles serve as a target for vaccines and other substances responsible for immune response, as hair follicles have a high concentration of immunocompetent cells in the infundibular part of root sheath and around the excretory duct of sebaceous gland. The environment of the follicular infundibulum produces a continuous stream of sebum from the outlet of the sebaceous gland that moves towards the follicular orifice. Therefore, nanoparticles that are able to penetrate beyond follicular infundibulum or below the excretory duct of the sebaceous gland, cannot flush out by sebum, and thus nanoparticles containing vaccine or other substances can elicit a higher immune response (Jung et al. Citation2006).
Cell-penetrating peptides (CPPs)
The efficient permeability of therapeutically active molecules through the biological membranes remains a most important hurdle for drug delivery. Protein transduction domains (PTDs) or cell-penetrating peptides (CPPs) or membrane transduction peptides (MTPs) consist of 30 or less amino acids, are classified as either cationic or amphipathic and have ability to cross the cell membrane and enter into cells. The research on CPPs mainly have focused on structural characteristics of CPPs that give them the capability to translocate the cellular membrane barriers and hence lead to the development and engineering of several new classes of CPPs with optimum activity. The ability of CPPs such as trans-acting activator of transcription (TAT) and pAntp (penetratin) to translocate is attributed to their amino acid sequence which is mainly contributed by basic amino acids. It has been also concluded that minimal peptide sequence is involved in translocation process. Overall, the translocating peptides can be basic, amphipathic, hydrophobic or chimera in nature and some of the CPPs are summarized in .
Table I. Examples of different cell-penetrating peptides (CPPs) and their sequences.
Skin penetration of CPPs
Because of highly organized structure of the SC, it is difficult to deliver macromolecules such as proteins and peptides across the skin. The topical use of peptides is widely studied due to their importance in treating the skin diseases, for topical vaccination and in cosmetics to improve skin conditions. In recent years, CPPs have been used to enhance the topical delivery of proteins and peptides.
Rothbard et al. (Citation2000) were first to report the application of CPP for the delivery of peptides into the skin. Peptide R7 (Polyarginine-7) alone can cross the skin barrier and enter into the epidermis. The depth and intensity of skin penetration of arginine rich peptides depends on length and concentration of peptide applied. They studied the effects of non-releasable and releasable R7-CyclosporineA (CsA) conjugates on the skin. The non-releasable R7-CsA conjugate can permeate into epidermis and dermis. Releasable conjugate R7-CsA can release therapeutically-effective drug and its activity has been shown in a dose-dependent manner in vitro and in vivo. Wender et al. (Citation2002) have showed that biotin peptide can effectively penetrate across the SC and in all skin layers with intensely nuclear localization in epidermal cells when applied topically as biotin-transporter-9 conjugate.
Antioxidant enzymes like superoxide dismutase and catalase are very effective against the diseases mediated by reactive oxygen species such as skin inflammation, skin cancer, cutaneous autoimmune diseases, phototoxicity and skin ageing (Jin et al. Citation2001). Polylysine-9 (K9) (Park et al. Citation2002) and TAT (Kim et al. Citation2003, Eum et al. Citation2005) coupled antioxidant enzymes can translocate into epidermis and dermis. The level of enzyme activity in skin increases significantly when treated with the CPP-coupled catalases compared to untreated skin or skin treated only with catalases. TAT linked with the small peptide GKH (glycine-lysine-histidine) showed 36 times more absorption compared to naked GKH (Lim et al. Citation2003).
Schutze-Redelmeier et al. (Citation2004) reported that the antennapedia transduction sequence can also enhance the skin permeation of peptides. They observed enhanced skin permeation following application of antigenic peptide (OVA257–264) coupled with penetratin while non-conjugated antigenic peptide alone remained on the skin surface. A similar study to deliver P20 (Heat shock protein 20) peptide into skin by using CPPs such as YARA (YARAAARQARA), WLR (WLRRIKAWLRRIKAWLRRIKA) and TAT was conducted by Lopes et al. (Citation2005, Citation2008). They evaluated the ability of CPPs to carry conjugated peptides into the skin and compared that to a non-transducing peptide YKAc (YKALRISRKLAL). Chemical enhancers such as monoolein and oleic acid have no effect on the skin permeation of CPPs but these enhancers can increase the skin penetration of YKAc. In contrast to these studies, Hou et al. (Citation2007) have demonstrated that the oleic acid can enhance the transport of non-covalently attached CPP-Protein (GFP) into the skin but the effect is not synergistic. This may be because the chemical enhancers only show their effects when transduction ability of peptides is limited. Therefore YKAc when tested with enhancers showed enhanced permeation into the skin (Lopes et al. Citation2005). The CPP-mediated cutaneous delivery of proteins and peptides is dependent on the nature of CPP, conjugation method (covalent or non-covalent type of attachment), molar ratio and characteristics of the cargo protein.
Mechanism of CPP-mediated delivery into skin
Over the decades, CPPs have opened new avenues in the field of drug delivery but the mechanism by which CPPs enter into the cells has been a matter of debate. Firstly, it was suggested that CPPs can translocate across the cellular membrane by a receptor and energy independent mechanism, i.e., endosomal independent pathway (Vives et al. Citation1997). Although varieties of mechanisms for the translocation capability of CPPs have been suggested, parameters such as charge, primary sequence, length, linearity and chirality of the peptides can influence the translocation efficiency of CPPs. The presences of positive-charged arginine groups in CPPs enhances their binding to negatively charged cell surfaces by electrostatic interactions. A single CPP may use multiple pathways such as energy dependent endocytosis, energy independent direct translocation across membranes or utilize these alternate pathways concurrently.
It is known that the intercellular lipid domain of SC is different than any other cellular membrane by means of lipid composition, lipid/protein ratio and water content. Because of the non-viable nature of the SC the transport mechanism can be predicted to be different than that of the normal cell membrane (Rothbard et al. Citation2000). To understand the mode of action of CPPs in skin, Rothbard et al. (Citation2000) investigated the mechanism of transport of oligo-arginine conjugates into the cutaneous barrier and demonstrated that the conjugates penetrate intact into the skin. CPPs and conjugated cargoes can enter into the cell by active transport across lipid cellular membrane. Thus CPPs enter the layers of cells and get accumulated there, and then enter the adjacent layers presumably by forming a gradient. However, the exact mechanism of the transport of CPPs through skin layers remains unclear. The interaction of CPPs with lipids may be the main transport across SC as this interaction may destabilize SC resulting in an increase in the membrane permeability. Another mechanism of transport is suggested through the tight junctions of the skin. CPPs are proposed to disrupt these junctions and allow penetration into the viable skin layers (Lopes et al. Citation2008). Macropinocytosis is another possible transport pathway for the CPPs into mammalian cells. The cellular entry and transdermal delivery of CPP has been shown to involve the macropinocytosis and actin reorganization (Hou et al. Citation2007). However, this mechanism of transport is less likely to be involved in penetration of non-covalently attached CPPs-Cargoes (Lopes et al. Citation2008). Hence, further studies are required to understand the exact mechanism(s) of the CPP-mediated transport from non-viable to viable layers of the skin.
Cell-penetrating peptides-modified nanoparticles
From the published studies, it is clear that various pharmaceutical nanocarriers (like liposomes and nanoparticles have been modified with CPPs) present opportunities to increase crossing the skin barriers. CPPs have the potential to carry the lipid pay load such as lipid nanoparticles (liposomes and NLC) across the skin layers. As mentioned above, TAT has been widely exploited for intracellular delivery of peptides, proteins, nucleic acids, antibodies, imaging agents as well as nanoparticulate sytems (Torchilin Citation2008). Kang et al. (Citation2010) studied the in vitro and in vivo effect of TAT coated elastic liposomes (EL/T) in comparison with conventional cream (CC), TAT added conventional cream (CC/T), elastic liposomes (EL) and conventional liposomes (CL). In vitro skin penetration was in the following order: EL/T > EL > CC/T ≥ CL > CC. TAT peptide was demonstrated to increase the flux for CC or EL by about 20%. Therefore, TAT containing elastic liposomes are efficient delivery systems to enhance the skin penetration in vitro and in vivo. From these studies, however, it is not clear whether liposomes are conjugated to TAT or not. Our laboratory has also explored the ability of CPP to translocate lipid nanoparticles into the skin layers (Patlolla et al. Citation2010b). To understand the translocation of TAT peptide-coated nanoparticles into the skin, fluorescent dye, DID-Oil, encapsulated NLC were prepared and surface modification was carried out using DOGS-NTA-Ni lipid, where NTA-Ni has strong binding affinity towards the histidine groups of His-tagged TAT or control His–tagged YKA. Confocal microscopy and Raman spectroscopy studies revealed that TAT modification enhanced the skin penetration in a time-dependent manner. TAT coated and fluorescent dye encapsulated NLC increase the fluorescence intensity up to 120 μm of skin depth. Furthermore, our confocal microscopy studies show that the fluorescent dye localized in epidermal and upper dermal layers and also in the hair follicles (). NLC alone and NLC coated with control non-transduction YKA peptide localized mainly on SC surface and in appendages. Furthermore, surface modification of NLC with TAT peptide can significantly increase the skin permeation of celecoxib, a model lipophilic anti-inflammatory molecule, in all skin layers compared to control NLC alone and YKA-coated NLC formulations. The TAT peptide-mediated nanoparticle translocation into the skin follows a sequence of events. Firstly, the positive charge of the TAT peptide helps to bind with skin cells, and then water evaporation from the nanoparticles leads to formation of a thin lipid monolayer on the skin surface. This occlusive property of nanoparticles facilitates their entry into the SC and from this point TAT might enhance the penetration of nanoparticles into viable layers of the skin. This study indicates that CPP can deliver lipid pay loads into the skin and hence are useful in the delivery of small and large therapeutics having poor skin penetration.
Figure 2. Topical delivery of CPP-coated NLC in rat skin: Lipophilic fluorescent dye (DID) was encapsulated in various NLC preparations and the particles were incubated with rat skin in vitro. The vertical skin sections with a thickness of 30 μm were made with cryotome and were observed under confocal microscope for skin associated fluorescence. Left panel, DID fluorescence; right panel, overlay of bright field and fluorescence. Results indicate that surface modification of nanoparticles with TAT enhances the skin permeation, whereas addition of non-transduction peptide (YKA) inhibits the entry of nanoparticles into the skin.
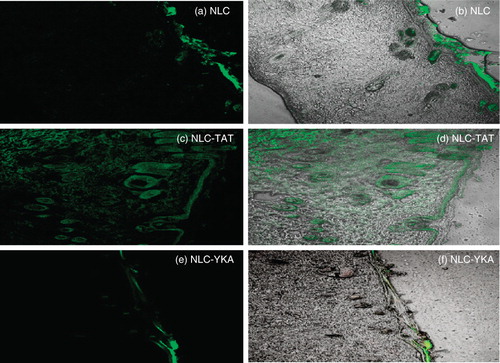
Conclusion
The nature of skin offers a formidable barrier to the delivery of small and large molecular active therapeutic agents. The SC plays a pivotal role in the skin's barrier function. Despite different skin penetration enhancement techniques, topical and transdermal delivery remains a challenge because of the toxicity associated with the high concentrations of chemical enhancers and inconvenience of using electrical methods associated with high production costs. From the myriad published studies involving nanoparticles, it is clear that nanoparticles have the potential to effectively deliver drugs across the skin barrier. Conventional liposomes, flexible liposomes and ultradeformable liposomes offer potential value as dermal and transdermal drug delivery. SLN and NLC nanoparticles have improved drug stability and high cutaneous absorption of incorporated drugs. The rigid nanoparticles do not penetrate the entire SC but they can follow transappendageal pathway. Hair follicles represent an efficient penetration pathway and reservoir for topically applied substances. However, an understanding of the exact mechanism(s) by which these carriers act as skin drug delivery systems remains to be fully explored. In addition to nanoparticles, CPPs present another class of promising candidates as carrier molecules to deliver the therapeutics in the skin. The CPP-based delivery system into skin has extended from proteins to nanocarriers. CPP can translocate nanoparticles with their payloads into the viable layers of skin.
Acknowledgements
We thank Ruth Didier, College of Medicine, Florida State University Tallahassee for her kind help in Confocal Microscopy studies. The work carried out in the authors' laboratory was funded by the financial assistance provided by National Institute of Arthritis and Musculoskeletal and Skin Diseases (NIAMS) – National Institutes of Health (NIH) grant number AR47455-02 and Research Centers in Minority Institutions (RCMI) – National Institutes of Health (NIH) grant number G12RR03020.
Declaration of interest : The authors report no conflicts of interest. The authors alone are responsible for the content and writing of the paper.
References
- Alvarez-Román R, Naik A, Kalia YN, Guy RH, Fessi H. 2004a. Enhancement of topical delivery from biodegradable nanoparticles. Pharm Res 21:1818–1825.
- Alvarez-Román R, Naik A, Kalia YN, Guy RH, Fessi H. 2004b. Skin penetration and distribution of polymeric nanoparticles. J Control Release 99:53–62.
- Barbosa-Barros L, Barba C, Rodríguez G, Cócera M, Coderch L, López-Iglesias C, de la Maza A, López O. 2009. Lipid nanostructures: Self-assembly and effect on skin properties. Mol Pharm 6:1237–1245.
- Baroli B, Ennas MG, Loffredo F, Isola M, Pinna R, López-Quintela MA. 2007. Penetration of metallic nanoparticles in human full-thickness skin. J Invest Dermatol 127:1701–1712.
- Bos JD, Meinardi MMHM. 2000. The 500 Dalton rule for the skin penetration of chemical compounds and drugs. Exp Dermatol 9:165–169.
- Bouwstra JA, Honeywell-Nguyen PL, Gooris GS, Ponec M. 2003. Structure of the skin barrier and its modulation by vesicular formulations. Prog Lipid Res 42:1–36.
- Carrer DC, Vermehren C, Bagatolli LA. 2008. Pig skin structure and transdermal delivery of liposomes: A two photon microscopy study. J Control Release 132:12–20.
- Cevc G, Blume G, Schatzlein A, Gebauer D, Paul A. 1996. The skin: A pathway for systemic treatment with patches and lipid-based agent carriers. Adv Drug Deliv Rev 18:349–378.
- Cevc G. 2004. Lipid vesicles and other colloids as drug carriers on the skin. Adv Drug Deliv Rev 56:675–711.
- Cross SE, Innes B, Roberts MS, Tsuzuki T, Robertson TA, McCormick P. 2007. Human skin penetration of sunscreen nanoparticles: In-vitro assessment of a novel micronized zinc oxide formulation. Skin Pharmacol Physiol 20:148–154.
- de Leeuw J, de Vijlder HC, Bjerring P, Neumann HA. 2009. Liposomes in dermatology today. J Eur Acad Dermatol Venereol 23:505–516.
- Derossi D, Joliot AH, Chassaing G, Prochiantz A. 1994. The third helix of the Antennapedia homeodomain translocates through biological membranes. J Biol Chem 269:10444–10450.
- El Maghraby GM, Barry BW, Williams AC. 2008. Liposomes and skin: From drug delivery to model membranes. Eur J Pharm Sci 34:203–222.
- El Maghraby GM, Williams AC, Barry BW. 2001. Skin hydration and possible shunt route penetration in controlled estradiol delivery from ultradeformable and standard liposomes. J Pharm Pharmacol 53:1311–1322.
- Elliott E, O'Hare P. 1997. Intercellular trafficking and protein delivery by a herpesvirus structural protein. Cell 88:223–233.
- Elsayed MM, Abdallah OY, Naggar VF, Khalafallah NM. 2007. Lipid vesicles for skin delivery of drugs: Reviewing three decades of research. Int J Pharm 332:1–16.
- Eum WS, Jang SH, Kim DW, Choi HS, Choi SH, Kim SY, An JJ, Lee SH, Han K, Kang JH, Kang TC, Won MH, Cho YJ, Choi JH, Kim TY, Park J, Choi SY. 2005. Enhanced transduction of Cu,Zn-superoxide dismutase with HIV-1 Tat protein transduction domains at both termini. Mol Cells 19:191–197.
- Futaki S. 2002. Arginine-rich peptides: Potential for intracellular delivery of macromolecules and the mystery of the translocation mechanisms. Int J Pharm 245:1–7.
- Gamer AO, Leibold E, van Ravenzwaay B. 2006. The in vitro absorption of microfine zinc oxide and titanium dioxide through porcine skin. Toxicol In Vitro 20:301–307.
- Hadgraft J. 2001. Modulation of the barrier function of the skin. Skin Pharmacol Appl Skin Physiol 14:72–81.
- Ho A, Schwarze SR, Mermelstein SJ, Waksman G, Dowdy SF. 2001. Synthetic protein transduction domains: Enhanced transduction potential in vitro and in vivo. Cancer Res 61:474–477.
- Honeywell-Nguyen PL, Bouwstra JA. 2005. Vesicles as a tool for transdermal and dermal delivery. Drug Disc Today: Technol 2:67–74.
- Honeywell-Nguyen PL, de Graaff AM, Groenink HW, Bouwstra JA. 2002. The in vivo and in vitro interactions of elastic and rigid vesicles with human skin. Biochim Biophys Acta 1573:130–140.
- Honeywell-Nguyen PL, Gooris GS, Bouwstra JA. 2004. Quantitative assessment of the transport of elastic and rigid vesicle components and a model drug from these vesicle formulations into human skin in vivo. J Invest Dermatol 123:902–910.
- Honeywell-Nguyen PL, Wouter Groenink HW, de Graaff AM, Bouwstra JA. 2003. The in vivo transport of elastic vesicles into human skin: Effects of occlusion, volume and duration of application. J Control Release 90:243–255.
- Hou YW, Chan MH, Hsu HR, Liu BR, Chen CP, Chen HH, Lee HJ. 2007. Transdermal delivery of proteins mediated by non-covalently associated arginine-rich intracellular delivery peptides. Exp Dermatol 16:999–1006.
- Huang SS, Sung SH, Chiang CE. 2007. Chitosan potentiation of warfarin effect. Ann Pharmacother 41:1912–1914.
- Jin LH, Bahn JH, Eum WS, Kwon HY, Jang SH, Han KH, Kang TC, Won MH, Kang JH, Cho SW, Park J, Choi SY. 2001. Transduction of human catalase mediated by an HIV-1 TAT protein basic domain and arginine-rich peptides into mammalian cells. Free Radic Biol Med 31:1509–1519.
- Jung S, Otberg N, Thiede G, Richter H, SterryW, Panzner S, Lademann J. 2006. Innovative liposomes as a transfollicular drug delivery system: Penetration into porcine hair follicles. J Invest Dermatol 126:1728–1732.
- Kang MJ, Eum JY, Jeong MS, Choi SE, Park SH, Cho HI, Cho CS, Seo SJ, Lee MW, Choi YW. 2010. Facilitated skin permeation of oregonin by elastic liposomal formulations and suppression of atopic dermatitis in NC/Nga mice. Biol Pharm Bull 33:100–106.
- Kanikkannan N, Kandimalla K, Lamba SS, Singh M. 2000. Structure-activity relationship of chemical penetration enhancers in transdermal drug delivery. Curr Med Chem 7:593–608.
- Kim DW, Eum WS, Jang SH, Yoon CS, Choi HS, Choi SH, Kim YH, Kim SY, Lee ES, Baek NI, Kwon HY, Choi JH, Choi YC, Kwon OS, Cho SW, Han K, Lee KS, Park J, Choi SY. 2003. Ginsenosides enhance the transduction of tat-superoxide dismutase into mammalian cells and skin. Mol Cells 16:402–406.
- Kirjavainen M, Urtti A, Jaaskelainen I, SuhonenTM, Paronen P, Valjakka-Koskela R, Kiesvaara J, Monkkonen J. 1996. Interaction of liposomes with human skin in vitro – the influence of lipid composition and structure. Biochim Biophys Acta 1304:179–189.
- Kong M, Chen XG, Liu CS, Liu CG, Meng XH, Yu le J. 2008. Antibacterial mechanism of chitosan microspheres in a solid dispersing system against E. coli. Colloids Surf B Biointerfaces 65:197–202.
- Küchler S, Herrmann W, Panek-Minkin G, Blaschke T, Zoschke C, Kramer KD, Bittl R, Schäfer-Korting M. 2010. SLN for topical application in skin diseases. Characterization of drug-carrier and carrier-target interactions. Int J Pharm (in press), PMID: 20153414.
- Küchler S, Radowski MR, Blaschke T, Dathe M, Plendl J, Haag R, Schäfer-Korting M, Kramer KD. 2009. Nanoparticles for skin penetration enhancement – a comparison of a dendritic core-multishell-nanotransporter and solid lipid nanoparticles. Eur J Pharm Biopharm 71:243–250.
- Kumar R, Philip A. 2007. Modified transdermal technologies: Breaking the barriers of drug permeation via the skin. Trop J Pharm Res 6:633–644.
- Lademann J, Knorr F, Richter H, Blume-Peytavi U, Vogt A, Antoniou C, Sterry W, Patzelt A. 2008. Hair follicles – an efficient storage and penetration pathway for topically applied substances. Skin Pharmacol Physiol 21:150–155.
- Lademann J, Otberg N, Richter H, Weigmann H-J, Lindemann U, Schaefer H. 2001. Investigation of follicular penetration of topically applied substances. Skin Pharmacol Appl Skin Physiol 14:17–22.
- Lademann J, Richter H, Teichmann A, Otberg N, Blume-Peytavi U, Luengo J, Weiss B, Schaefer UF, Lehr CM, Wepf R, Sterry W. 2007. Nanoparticles – an efficient carrier for drug delivery into the hair follicles. Eur J Pharm Biopharm 66:159–164.
- Lademann J, Weigmann H, Rickmeyer C, Barthelmes H, Schaefer H, Mueller G, Sterry W. 1999. Penetration of titanium dioxide microparticles in a sunscreen formulation into the horny layer and the follicular orifice. Skin Pharmacol Appl Skin Physiol 12:247–256.
- Lim JM, Chang MY, Park SG, Kang NG, Song YS, Lee YH, Yoo YC, Cho WG, Choi SY, Kang SH. 2003. Penetration enhancement in mouse skin and lipolysis in adipocytes by TAT-GKH, a new cosmetic ingredient. J Cosmet Sci 54:483–491.
- Lin YZ, Yao SY, Veach RA, Torgerson TR, Hawiger J. 1995. Inhibition of nuclear translocation of transcription factor NFkB by a synthetic peptide containing a cell membranepermeable motif and nuclear localization sequence. J Biol Chem 270:14255–14258.
- Lins L, Decaffmeyer M, Thomas A, Brasseur R. 2008. Relationships between the orientation and the structural properties of peptides and their membrane interactions. Biochim Biophys Acta 1778:1537–1544.
- Lombardi Borgia S, Regehly M, Sivaramakrishnan R, Mehnert W, Korting HC, Danker K, Röder B, Kramer KD, Schäfer-Korting M. 2005. Lipid nanoparticles for skin penetration enhancement-correlation to drug localization within the particle matrix as determined by fluorescence and parelectric spectroscopy. J Control Release 110:151–163.
- Lopes LB, Brophy CM, Furnish E, Flynn CR, Sparks O, Komalavilas P, Joshi L, Panitch A, Bentley MV. 2005. Comparative study of the skin penetration of protein transduction domains and a conjugated peptide. Pharm Res 22:750–757.
- Lopes LB, Furnish E, Komalavilas P, Seal BL, Panitch A, Bentley MV, Brophy CM. 2008. Enhanced skin penetration of P20 phosphopeptide using protein transduction domains. Eur J Pharm Biopharm 68:441–445.
- Lucks JS, Müller RH. 1991. Medication vesicles made of solid lipid particles (solid lipid Nanospheres SLN). EP0000605497.
- Luengo J, Weiss B, Schneider M, Ehlers A, Stracke F, König K, Kostka KH, Lehr CM, Schaefer UF. 2006. Influence of nanoencapsulation on human skin transport of flufenamic acid. Skin Pharmacol Physiol 19:190–197.
- Mehnert W, Mäder K. 2001. Solid lipid nanoparticles: Production, characterization and applications. Adv Drug Deliv Rev 47:165–196.
- Mezei M, Gulasekharam V. 1980. Liposomes – a selective drug delivery system for the topical route of administration. Lotion dosage form. Life Sci 26:1473–1477.
- Mezei M, Gulasekharam V. 1982. Liposomes – a selective drug delivery system for the topical route of administration: Gel dosage form. J Pharm Pharmacol 34:473–474.
- Mitchell DJ, Kim DT, Steinman L, Fathman CG, Rothbard JB. 2000. Polyarginine enters cells more efficiently than other polycationic homopolymers. J Pept Res 56:318–325.
- Mortensen LJ, Oberdörster G, Pentland AP, Delouise LA. 2008. In vivo skin penetration of quantum dot nanoparticles in the murine model: The effect of UVR. Nano Lett 8:2779–2787.
- Naik A, Kalia Y, Guy R. 2000. Transdermal drug delivery: Overcoming the skin's barrier function. Pharm Sci Technolo Today 3:318–326.
- Otberg N, Patzelt A, Rasulev U, Hagemeister T, Linscheid M, Sinkgraven R, Sterry W, Lademann J. 2008. The role of hair follicles in the percutaneous absorption of caffeine. Br J Clin Pharmacol 65:488–492.
- Panos I, Acosta N, Heras A. 2008. New drug delivery systems based on chitosan. Curr Drug Discov Technol 5:333–341.
- Park J, Ryu J, Jin LH, Bahn JH, Kim JA, Yoon CS, Kim DW, Han KH, Eum WS, Kwon HY, Kang TC, Won MH, Kang JH, Cho SW, Choi SY. 2002. 9-polylysine protein transduction domain: Enhanced penetration efficiency of superoxide dismutase into mammalian cells and skin. Mol Cells 13:202–208.
- Patel LN, Zaro JL, Shen WC. 2007. Cell penetrating peptides: Intracellular pathways and pharmaceutical perspectives. Pharm Res 24:1977–1992.
- Patlolla RR, Chougule M, Patel AR, Jackson T, Tata PN, Singh M. 2010a. Formulation, characterization and pulmonary deposition of nebulized celecoxib encapsulated nanostructured lipid carriers. J Control Release 144:233–241.
- Patlolla RR, Desai P, Belay K, Singh M. 2010b. Translocation of cell penetrating peptide engrafted nanoparticles across skin layers. Biomaterials 31:5598–5607.
- Rancan F, Papakostas D, Hadam S, Hackbarth S, Delair T, Primard C, Verrier B, Sterry W, Blume-Peytavi U, Vogt A. 2009. Investigation of polylactic acid (PLA) nanoparticles as drug delivery systems for local dermatotherapy. Pharm Res 26:2027–2036.
- Rossi S, Marciello M, Sandri G, Ferrari F, Bonferoni MC, Papetti A, Caramella C, Dacarro C, Grisoli P. 2007. Wound dressings based on chitosans and hyaluronic acid for the release of chlorhexidine diacetate in skin ulcer therapy. Pharm Dev Technol 12:415–422.
- Rothbard JB, Garlington S, Lin Q, Kirschberg T, Kreider E, McGrane PL, Wender PA, Khavari PA. 2000. Conjugation of arginine oligomers to cyclosporin A facilitates topical delivery and inhibition of inflammation. Nat Med 6:1253–1257.
- Ryman-Rasmussen JP, Riviere JE, Monteiro-Riviere NA. 2006. Penetration of intact skin by quantum dots with diverse physicochemical properties. Toxicol Sci 91:159–165.
- Schäfer-Korting M, Mehnert W, Korting HC. 2007. Lipid nanoparticles for improved topical application of drugs for skin diseases. Adv Drug Deliv Rev 59:427–443.
- Schramlová J, Blazek K, Bartácková M, Otová B, Mardesicová L, Zizkovský V, Hulínská D. 1997. Electron microscopic demonstration of the penetration of liposomes through skin. Folia Biol (Praha) 43:165–169.
- Schutze-Redelmeier MP, Kong S, Bally MB, Dutz JP. 2004. Antennapedia transduction sequence promotes anti tumour immunity to epicutaneously administered CTL epitopes. Vaccine 22:1985–1991.
- Shanmugam S, Song CK, Nagayya-Sriraman S, Baskaran R, Yong CS, Choi HG, Kim DD, Woo JS, Yoo BK. 2009. Physicochemical characterization and skin permeation of liposome formulations containing clindamycin phosphate. Arch Pharm Res 32:1067–1075.
- Souto EB, Müller RH. 2008. Cosmetic features and applications of lipid nanoparticles (SLN, NLC). Int J Cosmet Sci 30:157–165.
- Stracke F, Weiss B, Lehr CM, König K, Schaefer UF, Schneider M. 2006. Multiphoton microscopy for the investigation of dermal penetration of nanoparticle-borne drugs. J Invest Dermatol 126:2224–2233.
- Szikszai Z, Kertész Zs, Bodnár E, Major I, Borbíró I, Kiss AZ, Hunyadi J. 2010. Nuclear microprobe investigation of the penetration of ultrafine zinc oxide into intact and tape-stripped human skin. Nuclr Instru and Meth B 268:2160–2163.
- Teeranachaideekul V, Boonme P, Souto EB, Müller RH, Junyaprasert VB. 2008. Influence of oil content on physicochemical properties and skin distribution of Nile red-loaded NLC. J Control Release 128:134–141.
- Teo LA, Shearwood C, Chye Ng K, Lu J, Moochhala S. 2006. Transdermal microneedles for drug delivery applications. Mater Sci Eng: B 132:151–154.
- Tokudome Y, Saito Y, Sato F, Kikuchi M, Hinokitani T, Goto K. 2009. Preparation and characterization of ceramide-based liposomes with high fusion activity and high membrane fluidity. Colloids Surf B Biointerfaces 73:92–96.
- Toll R, Jacobi U, Richter H, Lademann J, Schaefer H, Blume-Peytavi U. 2004. Penetration profile of microspheres in follicular targeting of terminal hair follicles. J Invest Dermatol 123:168–176.
- Torchilin VP. 2008. Cell penetrating peptide-modified pharmaceutical nanocarriers for intracellular drug and gene delivery. Biopolymers 90:604–610.
- Touitou E, Dayan N, Bergelson L, Godin B, Eliaz M. 2000. Ethosomes – novel vesicular carriers for enhanced delivery: Characterization and skin penetration properties. J Control Release 65:403–418.
- Verma DD, Verma S, Blume G, Fahr A. 2003. Liposomes increase skin penetration of entrapped and non-entrapped hydrophilic substances into human skin: A skin penetration and confocal laser scanning microscopy study. Eur J Pharm Biopharm 55:271–277.
- Vives E, Brodin P, Lebleu B. 1997. A truncated HIV-1 Tat protein basic domain rapidly translocates through the plasma membrane and accumulates in the cell nucleus. J Biol Chem 272:16010–16017.
- Vogt A, Combadiere B, Hadam S, Stieler KM, Lademann J, Schaefer H, Autran B, Sterry W, Blume-Peytavi U. 2006. 40 nm, but not 750 or 1500 nm, nanoparticles enter epidermal CD1a+ cells after transcutaneous application on human skin. J Invest Dermatol 126:1316–1322.
- Weller K, Lauber S, Lerch M, Renaud A, Merkle HP and Zerbe O. 2005. Biophysical and biological studies of end-group-modified derivatives of Pep-1. Biochemistry 44:15799–15811.
- Wender PA, Rothbard IB, Jessop TC, Kreider EL, Wylie BL. 2002. Oligocarbamate molecular transporters: Design, synthesis, and biological evaluation of a new class of transporters for drug delivery. J Am Chem Soc 124:13382–13383.
- Wissing SA, Müller RH. 2003. Cosmetic applications for solid lipid nanoparticles (SLN). Int J Pharm 254:65–68.
- Zhang LW, Monteiro-Riviere NA. 2008. Assessment of quantum dot penetration into intact, tape-stripped, abraded and flexed rat skin. Skin Pharmacol Physiol 21:166–180.
- Zhang LW, Yu WW, Colvin VL, Monteiro-Riviere NA. 2008. Biological interactions of quantum dot nanoparticles in skin and in human epidermal keratinocytes. Toxicol Appl Pharmacol 228:200–211.