Abstract
Constituting functional interactions between proteins and lipid membranes is one of the essential features of cellular membranes. The major challenge of quantitatively studying these interactions in living cells is the multitude of involved components that are difficult, if not impossible, to simultaneously control. Therefore, there is great need for simplified but still sufficiently detailed model systems to investigate the key constituents of biological processes. To specifically focus on interactions between membrane proteins and lipids, several membrane models have been introduced which recapitulate to varying degrees the complexity and physicochemical nature of biological membranes. Here, we summarize the presently most widely used minimal model membrane systems, namely Supported Lipid Bilayers (SLBs), Giant Unilamellar Vesicles (GUVs) and Giant Plasma Membrane Vesicles (GPMVs) and their applications for protein-membrane interactions.
Keywords::
Introduction
Biophysical studies generally aim at a quantitative determination of key parameters to characterize a functional biological system. A characteristic of many, if not all, biological systems is their enormous complexity. Therefore, the reconstitution of cellular or molecular subsystems of reduced complexity in cell-free, well-controlled environments has been, and remains, an important task in biological science. This approach thus refers to the modern field of Synthetic Biology, as it implies that a specific biological phenomenon may be approached ‘bottom-up', from a minimal assembly of its functional parts (Schwille and Diez Citation2009, Arumugam et al. Citation2011, Schwille Citation2011). In the present paper, we give a short overview over approaches to understand membrane related biological phenomena by employing suitable model systems simple enough to allow for quantitative analysis, but on the other side complex enough to retain the fundamental character of the phenomena to be observed.
The structural and functional characterization of lipid-protein interactions in biological membranes has always been an enormous challenge to cell biology. Although many biochemical techniques exist for measuring protein-protein interactions, there has been a lack of tools for protein-lipid interactions, since there are no specific targeting agents for lipids, as there are in the form of antibodies for proteins. Taking this into account, the possibility of addressing the specific lipid environment of a protein, or reversely, its segregation into membrane domains of a certain physical nature became an attractive approach. In addition to recent advances in microscopy and spectroscopy that have allowed investigation of protein-lipid or rather, protein-lipid membrane domain interactions in live cells (Sezgin and Schwille Citation2011), several advanced minimal model membrane systems have helped to address the issue from a ‘bottom-up' paradigm. Here, we summarize the presently most widely used model membrane systems, along with the key membrane properties relevant for protein-membrane interactions.
Historical background
The first experimental investigation of the remarkable physicochemical features of lipidic systems was documented by Benjamin Franklin, in order to understand the calming effect of ‘pouring oil on troubled water'. He remembers the first sparks of his curiosity, when he made the observation that emptying the ship kitchen's greasy cleaning water significantly smoothened their wake (Tanford Citation2004). In the description of the now-famous Clapham pond experiment, he noted how only a teaspoon of oil covered a significant region of the pond, making it look smooth as glass and explained the physical mechanisms of thin film formation on air-water interface (Tanford Citation2004). Thus, the first research on lipidic surfaces started with half a membrane – a monolayer (i.e., a monomolecular film formed by lipids on an air-water interface) – well before the concept of a cell membrane was proposed in 1899 by Overton (Overton Citation1899, Park Citation1968). Parallel with the discovery of the ultrathin structure of the plasma membrane in 1925 (Gorter and Grendel Citation1925), Langmuir undertook the first quantitative characterization of monolayers (Langmuir Citation1917). Decades later, liposomes, the first bilayer system, were discovered (Bangham and Horne Citation1964). These discoveries led to the ‘Fluid Mosaic' model of the cellular plasma membrane (Singer and Nicolson Citation1972), describing it as a homogenous lipid environment with embedded proteins, which appeared to be remarkably accurate.
The first major correction to the concept of a homogeneous fluid mosaic followed upon the discovery of detergent resistant membranes (DRMs), which required a model explaining a heterogeneous lipid structure of the plasma membrane (Yu et al. Citation1973). The 1980s were the golden age of research on pure lipid model membranes, when the phase separation phenomenon was established for monolayers, and supported lipid bilayers were first prepared (McConnell et al. Citation1984, Tamm and McConnell Citation1985). A few years later, Giant Unilamellar Vesicles were introduced as a membrane model system that allowed microscopic investigation of cell-sized membrane vesicles (Angelova and Dimitrov Citation1986). Meanwhile, the heterogeneous distribution of lipids in the apical, compared to the basolateral, plasma membrane in polarized epithelia was observed, strengthening the concept of heterogeneous membrane structure (Vanmeer et al. Citation1987). Although detergent resistant proteins were also observed in early 1990s (Brown and Rose Citation1992), it was 1997 when the raft concept was introduced, proposing functional lipid-induced domains (Simons and Ikonen Citation1997). To confirm and pinpoint the molecular details behind this phenomenon, phase separation was extensively investigated in synthetic membrane systems as models for lipid raft-mediated membrane heterogeneity (Korlach et al. Citation1999, Bagatolli and Gratton Citation2000). An important step to a more biological relevance of these phase-separating membrane models was the achievement of functional protein reconstitution into them (Bacia et al. Citation2004, Girard et al. Citation2004, Kahya et al. Citation2005). The most recent manifestation of this quest is the observation of liquid-liquid phase separation in isolated plasma membranes (GPMVs) (Baumgart et al. Citation2007), 30 years after their discovery (Scott Citation1976) ().
Membrane features involved in protein-lipid interactions
Membrane composition
As membranes are composed of a collective of lipids and proteins, the main determinant of membrane organization is their constituent molecular composition. However, thorough characterization of the molecular composition of cellular membranes has remained challenging, due to the lack of appropriate techniques. Recent advances in analytical technologies have addressed this issue. For example, mass spectrometric proteomics and lipidomics () have begun to define the compositions of individual cell compartments (Aebersold and Mann Citation2003, Marxer et al. Citation2005, Sampaio et al. Citation2011). Similarly, NMR and Raman spectroscopy have been used to determine the lipid/protein compositions of cell membranes (Wright et al. Citation1997, Aussenac et al. Citation2003, Boehme et al. Citation2009, Jakop et al. Citation2009, Estrada et al. Citation2010). The difficulties and ambiguities of determining lipid compositions of native cellular membranes have actually spurred the development of Giant Unilamellar Vesicles (GUVs), to be discussed in detail below, as a very controlled assembly of binary or ternary lipid mixtures into valuable model systems. It is foreseeable that in the same way as the improvement of analytical methods such as mass spectrometry will in the future allow for a better and more detailed lipidomics, the relevance of well-defined GUVs in biology will diminish.
Figure 2. Membrane features involved in protein-lipid interactions. (A) Lipid composition as determined by Mass Spectrometry. Lipid composition of MDCK cells varies during epithelial polarization. Individual lipid species can be quantified and have unique polarization-dependent behaviors (modified from Sampaio et al. Citation2011). (B) C-Laurdan spectra report cell-dependent membrane packing of plasma membranes (see Giant Plasma Membrane Vesicles) derived from different cell types. GP calculated as in Equation (1). (C) Membrane lateral pressure. Positive pressure at the head groups (1) and acyl chains (3) is balanced by lateral tension at the interface (2). (D) Hydrophobic match. Lipids adapt to the TMD length of the protein by interfacing long TMDs with long acyl chain lipids. (E) Phase separation. Confocal image of a Giant Unilamellar Vesicle (GUV) composed of DOPC/BSM/Chol shows Lo and Ld phases. Green is cholesterol (labeled with TopFluor) which prefers the Lo phase, while red is the Ld phase marker DiI. (F) Protein induced membrane curvature. This Figure is reproduced in colour in the online version of Molecular Membrane Biology.
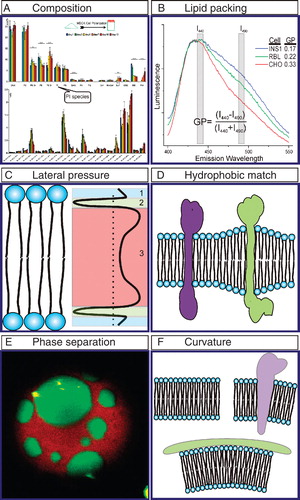
Clearly, the lipid composition of a membrane is the key determinant in all protein-membrane interactions, with many proteins using specific lipids, e.g., gangliosides (Coskun et al. Citation2011), as major targets for their membrane attachment. One lipid of particular importance for many cellular processes, involving cell polarization, but also as the key interface between the membrane and the cytoskeleton is the phosphatidylinositol, PIP2 (Ho et al. Citation2012), but other lipids may in the future be identified to provide similar hub functions for processes in and on membranes.
Lipid packing
In addition to the compositional complexity required for the many distinct functions of biological membranes, there exists a remarkable variety of possible lipid structures and consequently, local lipid packing. Lipids are generally grouped into classes by the structure of their hydrophilic head group (e.g., phosphatidylcholine), but the hydrophobic acyl chains also vary greatly in length and degree of saturation. These properties determine the relative packing of assembled membranes, with longer, more saturated lipids forming tightly packed, highly ordered membrane environments, whereas short and unsaturated lipids create looser membranes with more acyl chain flexibility. This packing can be studied by Electron Paramagnetic Resonance (EPR), Nuclear Magnetic Resonance (NMR) or fluorescence anisotropy (Baatz et al. Citation1990, Pincelli et al. Citation2000, Han et al. Citation2001, Vogel et al. Citation2005), although these methods typically report wholesale membrane properties, thus being of limited use to reveal the role of lateral heterogeneity. Recently, several fluorescent membrane probes have been developed and characterized (i.e., Laurdan, Prodan, C-Laurdan and Di-4-ANEPPDHQ) which are sensitive to water content, from which the relative packing of the membrane can be extrapolated at microscopic resolution (Parasassi et al. Citation1998, Jin et al. Citation2005, Kim et al. Citation2007). When these dyes are excited, their transition dipole moment increases, which results in a reorientation of the ambient water molecules, therefore their emission spectra depend on the aqueous content of the environment (Dietrich et al. Citation2001), with red-shifted emission indicative of more water and therefore less ordered membranes. A normalized, relative polarity index called generalized polarization (GP) characterizes the extent of emission shift between ordered (tightly packed, less aqueous) and disordered (loosely packed, more aqueous) environment. For example, the emission wavelength for Laurdan is 440 nm in highly ordered, and 490 nm in highly disordered environments (). The GP value is then calculated as:
with a theoretical range of −1 to +1, reflective of minimally and maximally ordered membranes, respectively.
Lipid packing, for instance, plays a role in apolipoprotein A-I (apoA-I) and high density lipoprotein (HDL) binding to membrane. By using Laurdan to probe the lipid packing, it was shown that apoA-I and HDL binding to the membrane prefer certain lipid packing (Sanchez et al. Citation2010). Dystrophin (a cytoplasmic protein which connects the cytoskeleton to the surrounding extracellular matrix) binding to di-oleyl phospholipids is also influenced by the lipid packing. Changing the lipid packing by introducing lipids such as phosphatidylethanolamine (PE) to the membrane leads to an approximately 7-fold increase in membrane binding affinity of Dystrophin, although Dystrophin does not bind directly to any PE lipid (Le Rumeur et al. Citation2007). Recently, it has been shown that cell membrane has the ability to form differential lipid packing which provides an important structural insight on the plasma membrane lateral organization (Levental et al. Citation2011, Sanchez et al. Citation2012).
Lateral pressure
Due to the amphiphilic nature of lipids, the pressure profile in the direction normal to the plane of the membrane is not constant, as shown in . A negative pressure (i.e., tension) is created at the interface between the water-rich polar headgroup region and the hydrophobic acyl chain region, in order to minimize the disfavored interactions between water molecules and the hydrophobic core. Meanwhile, a positive counteracting pressure is created by the conformational restriction of the hydrophobic acyl chains. These pressures and pressure gradients play important roles in the conformational states of transmembrane proteins (TMP), which may modulate their activity or interactions (Cantor Citation1997a, Citation1997b). As an example, dystrophin organization in the membrane was shown to be influenced by the lateral pressure profile (Sarkis et al. Citation2011). Although the lateral pressure profile, and therefore its effect on membrane-protein interactions, is difficult to measure experimentally, many simulation studies have investigated this phenomenon in detail (Marsh Citation2007, Ollila et al. Citation2011).
Hydrophobic (mis)matching
Transmembrane domains (TMDs) of TMPs are typically alpha helices with hydrophobic amino-acid side chains interfacing with the hydrophobic aliphatic tails of membrane lipids. To minimize the energetically unfavorable exposure of these hydrophobic residues to aqueous environments, lipids physically adapt to match TMDs of various lengths, a phenomenon described as hydrophobic matching. Hydrophobic matching brings together long TMD-containing proteins with long acyl chain lipids, resulting in local variations in membrane thickness (Jensen and Mouritsen Citation2004, Kaiser et al. Citation2011, Parton et al. Citation2011) (). This heterogeneity in bilayer thickness can serve to modulate membrane protein function (Andersen and Koeppe 2007). In addition, the thickening of the membrane due to enrichment of saturated and longer acyl chain lipids is supposed to be a characteristic feature of raft entities in cell membranes (Simons and Gerl Citation2010, Simons and Sampaio Citation2011), in the same way as the raft-like liquid-ordered phase in phase-separated model membranes (Heberle and Feigenson Citation2011, Mouritsen Citation2011). On the other hand, hydrophobic mismatching, i.e., a lack of match between TMD and membrane lipids, can also contribute to the organization of the membrane (Fattal and Benshaul Citation1993). In case of hydrophobic mismatch, proteins must either aggregate or change their conformation by reducing their effective hydrophobic length (Mouritsen and Bloom Citation1984, Schaefer et al. Citation2011), thereby affecting their functional state. The mismatch between POPC and the hydrophobic regions of BtuB (a bacterial outer-membrane beta barrel transport protein), around most of the BtuB circumference, is an example of hydrophobic mismatch which is thought to create the heterogeneity in bacterial outer membrane (Ellena et al. Citation2011).
Phase separation
Because of its unique interest to biophysics, but since the advent of the ‘lipid raft hypothesis' (Simons and Ikonen Citation1997) also to cell biology, one of the most widely investigated physicochemical phenomena of biological membranes is liquid-liquid phase separation resulting in lateral domain formation. Preferential interactions between saturated lipids and sterols can lead to the formation of a relatively tightly packed, ordered membrane environment, which readily separates from a relatively disordered phase enriched in unsaturated lipids (). The biological manifestation of this phenomenon, the ‘lipid raft hypothesis', proposes that segregated nanodomains in the cell membrane dynamically concentrate particular proteins/lipids in active platforms to take part in cellular processes (Lingwood and Simons Citation2010).
Phase separation can be readily visualized by fluorescent probes having a non-uniform partitioning between the coexisting phases. The phase preference of many such dyes, fluorescent lipid analogs, and fluorescently tagged proteins has been studied and utilized in the determination of membrane heterogeneity (Baumgart et al. Citation2007, Johnson et al. Citation2010, Juhasz et al. Citation2010, Sezgin et al. Citation2012b). Moreover, the membrane polarity-sensitive probes mentioned above are also extensively used for characterization of lipid domain properties (Bagatolli et al. Citation2003). Using these markers as references for the particular domain, phase preference of many proteins was tested in co-localization studies. The partitioning of SNAREs (Bacia et al. Citation2004), The β-secretase (BACE) (Kalvodova et al. Citation2005), the transmembrane linker for activation of T cells (LAT) (Levental et al. Citation2010b), Hemagglutinin of Influenza Virus (HA) (Nikolaus et al. Citation2010), GPI anchored proteins (Baumgart et al. Citation2007), inner leaflet-associated proteins (Sengupta et al. Citation2008) are some examples of protein partitioning in phase separated systems.
Membrane curvature
As a consequence of the chemical structure of the lipids, the activity of membrane proteins, or the presence of scaffolding proteins or the cytoskeleton, membranes can be curved at length scales that can be sensed by proteins and lipids (McMahon and Gallop Citation2005), (). Lipid-protein dynamics in a variety of membrane-related events (e.g., endocytosis and exocytosis) are directly driven by this membrane curvature (Kim et al. Citation1998, Biscari et al. Citation2002, Graham and Kozlov Citation2010, Baumgart et al. Citation2011). Pore formation by proapoptotic proteins (Basanez et al. Citation2001), interaction of the sterol carrier protein-2 with membranes (Huang et al. Citation1999), and binding of acyl-CoA binding protein (ACBP) to the membrane (Chao et al. Citation2002) are examples of processes in which curvature plays an important role. Moreover, membrane curvature itself may be regulated by particular protein-membrane interactions. BAR domain superfamily proteins, for example, are viewed as important regulators of membrane curvature (McMahon and Gallop Citation2005, Rao and Haucke Citation2011). BAR domain interaction with the membrane is accomplished by the amphipathic helices of the BAR domains (Bhatia et al. Citation2009) which proposes a general mechanism for curvature sensing and curvature-driven protein-membrane interactions (Hatzakis et al. Citation2009, Madsen et al. Citation2010).
Model systems for studying protein-lipid interactions
In the following, we summarize the three most frequently used biophysical assays to quantitatively address membrane-related phenomena. The first two (supported membranes, GUVs) were initially lipid-only systems, used to determine the physical chemistry of lipids. To specifically investigate the interactions of proteins with these membranes, several protocols have been developed (Kahya et al. Citation2001, Bacia et al. Citation2004, Richmond et al. Citation2011) in order to fully reconstitute the proteins into the membranes. GPMVs, on the other hand, represent a more top-down approach towards the study of lipid-protein systems, as they are derived from living cells by disrupting the membrane, and retain a large lipid complexity, along with an unknown number of integral and peripheral membrane proteins. Spanning from a single lipid bilayer (SLBs) to a complex cell membrane derived vesicle (GPMV), model membranes enabled membrane biologists to investigate the nature of protein-membrane interactions in a system with desired complexity, composition and structure.
Supported lipid bilayers (SLBs)
Supported lipid bilayers (SLBs) are flat membranes formed on a hydrophilic support (such as mica, glass or silica), containing a very thin (∼1 nm) layer of liquid between the support and the membrane. Thanks to the confinement on a solid support, changing physical conditions (e.g., buffer) without disrupting the membrane is much easier in SLBs than in free-standing membranes. Additionally, supported membranes are not only well accessible to all kinds of optical microscopy, but also to surface probe techniques such as atomic force microscopy (AFM), in contrast to free-standing bilayers (). Thus, in contrast to free-standing membranes, SLBs provide access to parameters of membrane thickness and mechanical stability (Chiantia et al. Citation2007) by AFM, and allow to resolve membrane structures far below the optical resolution limit (Chiantia et al. Citation2008).
Figure 3. Phase separation in Supported Lipid Bilayers (SLBs). (A) Confocal image of a SLB prepared from DOPC/BSM/C18Cer/Chol mixture and stained with DiD (Ld marker). (B) Topological image of the same membrane obtained with AFM. (C) Intensity and height profile of the membrane above. The DiD containing Ld patches are thinner than the patches Lo regions (Image courtesy of Grzegorz Chwastek). This Figure is reproduced in colour in the online version of Molecular Membrane Biology.
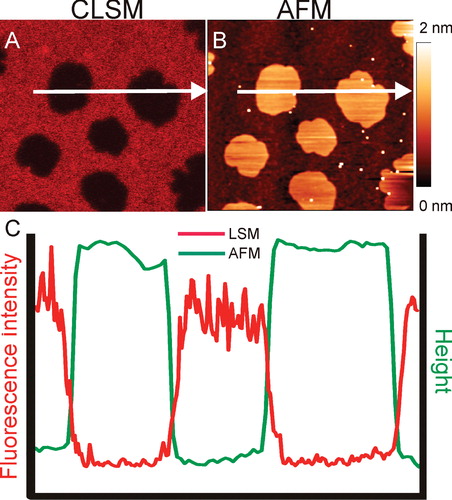
The first SLBs were achieved by the sequential transfer of monolayers from an air-water interface to a solid substrate (Tamm and McConnell Citation1985). Later attempts used the bursting of small vesicles (Small Unilamellar Vesicles [SUVs] or Large Unilamellar Vesicles [LUVs]) on a substrate. The vesicles are first adsorbed on the surface, then ruptured and spread into planar membranes (Richter et al. Citation2006). The most recent technological development has been SLBs deposited by spin coating membranes directly onto supports (Simonsen and Bagatolli Citation2004).
Although a solid-supported membrane is quite artificial compared to a free-standing one, the mobility of individual lipids can be preserved (Loose and Schwille Citation2009), making it a well-controlled system for studying lipid/protein dynamics. Some technical improvements have been introduced to arrive at a relatively unbiased mobility even of integral proteins reconstituted into SLBs. For example, polymer cushions have been placed on the support to increase the hydration layer, to avoid non-specific trapping of proteins with large extramembrane domains at the support (Wagner and Tamm Citation2000). Recently, to eliminate the effect of the surface/lipid interaction, a lipid bilayer was suspended between narrowly separated solid supports or at a small orifice, allowing the formation of a free-standing planar bilayer. This assay has been employed for simultaneously measuring optical and electrical properties of the membrane (Samsonov et al. Citation2001, Honigmann et al. Citation2010). Similarly, bursting vesicles on porous substrates (like alumina or Si3N4) is an alternative way to eliminate the effect of support but preserving the experimental advantages of flat SLBs (Hennesthal et al. Citation2002, Simon et al. Citation2007, Heinemann and Schwille Citation2011).
Interaction of Cytochrome C and alkaline phosphatase with the membrane was investigated using SLBs (Milhiet et al. Citation2002, Choi and Dimitriadis Citation2004). They were also used to figure out the effect of glycine to alanine substitution on interactions of antimicrobial peptide latarcin 2a with the membrane lipid bilayer (Idiong et al. Citation2011). Selective binding of HPA3, an antimicrobial and cell-penetrating peptide, to DMPC, DMPG, POPC and POPG is another example where SLBs were used as a model membrane system (Hirst et al. Citation2011). SLBs are also extensively used to mimic the bacterial membranes which provided valuable information about the prokaryotic cellular structures (Loose et al. Citation2008).
Giant Unilamellar Vesicles (GUVs)
In aqueous environments, lipid assemblies are easily transformed morphologically by changes of the physical parameters in the solution. Mild fluid convection, but also slowly alternating electric fields can lead to the vesiculation of a flat lipid multilayer, allowing the formation of unilamellar vesicles much larger than those prepared by sonication or injection methods, termed Giant Unilamellar Vesicles (GUVs >1000 nm). Originally, GUVs were formed using DC electric fields (Angelova and Dimitrov Citation1986), though AC electric fields were later found to increase formation efficiency (Angelova and Dimitrov Citation1988).
The basic protocol for GUV preparation involves the spreading of a lipid mixture (in organic solvent) on a platinum electrode to form the lipid multilayer. Electrodes are then dipped into aqueous buffer and the electric field (usually between 2 and 3 V) is applied. GUVs can also be prepared by gentle hydration, where charged lipids are used to create electrostatic repulsion between the bilayers, which eventually facilitates unilamellar vesicle formation. For details on GUV preparation refer to ref (Garcia-Saez et al. Citation2010, Morales-Penningston et al. Citation2010).
Besides having a great potential to selectively study single lipid species in a model membrane, it is also possible to form GUVs with complex lipid mixtures. This capability led to reconstitution of phenomena structurally and functionally similar to cellular lipid rafts in GUVs. One early assumption was that lipid rafts are related to more ordered, or more tightly packed, liquid membrane phases from the bulk membrane. The first attempts to observe phase separation in GUVs were made with binary mixtures of saturated phospholipids, namely DPPC/DLPC and DPPC/DPPE (Korlach et al. Citation1999, Bagatolli and Gratton Citation2000). These mixtures indeed phase separate under near-physiological conditions; however, while one of the phases retains the liquid-crystalline properties of biological membranes, the other is a solid crystal (in membranes, termed the ‘gel' phase) with little translational or rotational diffusion. Due to the limited biological relevance of the gel phase, another component was added to recapitulate liquid-liquid phase coexistence. The key ingredient turned out to be cholesterol-ternary mixtures of a saturated lipid, an unsaturated lipid and cholesterol readily resulted in coexisting liquid ordered (Lo) and liquid disordered (Ld) domains (Dietrich et al. Citation2001) ().
Figure 4. Phase Properties in GUVs. (A) 3D confocal image of phase separation in GUVs composed of DOPC/BSM/Chol (2:2:1; dyes are same as ). (B) Laurdan GP image of a phase separated GUV (same composition as [A]). Ordered phases have higher GP values. (C) Diffusion of a fluorescent molecule in Lo and Ld phases of Giant Unilamellar Vesicles (GUVs) quantified by fluorescence correlation spectroscopy (same composition as [A]). Diffusion is approximately an order of magnitude slower in the Lo phase. (D) Height profile of a burst GUV (same composition as [A]) obtained by AFM. The Lo phase is thicker than Ld phase by about 1 nm. This Figure is reproduced in colour in the online version of Molecular Membrane Biology.
![Figure 4. Phase Properties in GUVs. (A) 3D confocal image of phase separation in GUVs composed of DOPC/BSM/Chol (2:2:1; dyes are same as Figure 2A). (B) Laurdan GP image of a phase separated GUV (same composition as [A]). Ordered phases have higher GP values. (C) Diffusion of a fluorescent molecule in Lo and Ld phases of Giant Unilamellar Vesicles (GUVs) quantified by fluorescence correlation spectroscopy (same composition as [A]). Diffusion is approximately an order of magnitude slower in the Lo phase. (D) Height profile of a burst GUV (same composition as [A]) obtained by AFM. The Lo phase is thicker than Ld phase by about 1 nm. This Figure is reproduced in colour in the online version of Molecular Membrane Biology.](/cms/asset/94e6d21d-387c-45f9-9657-e4e2298676ed/imbc_a_700490_f0004_b.jpg)
In addition to their crucial role in defining the physical chemistry of raft-mimetic Lo phases, GUVs have been widely used as platforms to investigate the role of individual lipids in protein activity or interactions. Profilin interaction with phosphatidylinositol (4,5)-bisphosphate (Krishnan et al. Citation2009), caspase-8/Bid-FL complex binding to cardiolipin (Jalmar et al. Citation2010), tBID interaction with BCL(XL) (Garcia-Saez et al. Citation2009), and the activity of pore forming peptides (Fuertes et al. Citation2010) are some of the biological processes where GUVs have been used as model platforms. The distribution and the mobility of the lipid-anchored oligopeptide-binding protein OppA, the translocator complex OppBCDF of the oligopeptide ABC transporter, the mechanosensitive channel of MscL and the secondary lactose transport protein LacS were studied using GUVs (Doeven et al. Citation2005). SNAREs (Bacia et al. Citation2004) and beta secretase (BACE) proteins (Kalvodova et al. Citation2005) were also reconstituted into GUVs to elucidate their Lo/Ld phase preference and their lipid-modulated bioactivity.
Giant plasma membrane vesicles
Although non-lipid cellular components (such as functional membrane proteins) can be reconstituted into GUVs, these experiments are rather work-intensive and still only capable of reproducing a limited complexity, compared to the physiological situation. This is particularly true for the extensive variety of lipids in a native cellular membrane. An optimal complement to simple GUV models is a free-standing membrane which retains at least the compositional lipid complexity of a live cell membrane. Such a system is provided by so-called membrane blebs, which can be grown and harvested from native cellular membranes (Sezgin et al. Citation2012a).
Blebs are pure cell membrane regions, detached from the cell cortex, which can naturally form during cell division, apoptosis or cell migration, or are induced by some chemical or physical destruction of the cytoskeleton. In dividing cells, blebs provide an attractive system for studying the cell mechanics and biophysics of membrane-cytoskeleton interactions in live cells (Charras et al. Citation2008).
Chemically-induced cell membrane vesiculation (so-called plasma membrane vesicles [PMVs]) was first observed in 1976, and used as a method to isolate plasma membrane (Scott Citation1976). Recently, phase separation was observed in Giant Plasma Membrane Vesicles (GPMVs) (Baumgart et al. Citation2007) (), mirroring the behavior of the three component GUVs described above in a system retaining the compositional complexity of the native plasma membrane. Measurements of temperature (Johnson et al. Citation2010) and composition dependence of phase separation in GPMVs (Keller et al. Citation2009) have further confirmed these similarities. A similar method was developed to separate intact Plasma Membrane Spheres (PMS) from the cell interior (Lingwood et al. Citation2008), and phase separation was shown by confocal imaging and fluorescence correlation spectroscopy.
Figure 5. Phase Properties in Giant Plasma Membrane Vesicles (GPMVs). (A) Phase separation of GPMVs at 10°C stained with Topfluor Cholesterol (ordered phase), DiI (disordered phase) or Alexa647-Choleratoxin (ordered phase). (B) Laurdan GP image of a phase separated GPMV at 10°C. The order difference between coexisting phases is less pronounced than in GUVs shown in . (C) The diffusion of a fluorescent molecule in two phases of GPMVs measured by FCS. Diffusion in the ordered phase is slower, but much closer to the disordered phase than in GUV shown in . (D) AFM image of a burst GMPV. The surface is covered by proteins. The inset shows the corresponding confocal image of the membrane stained with DiI (disordered phase). This Figure is reproduced in colour in the online version of Molecular Membrane Biology.
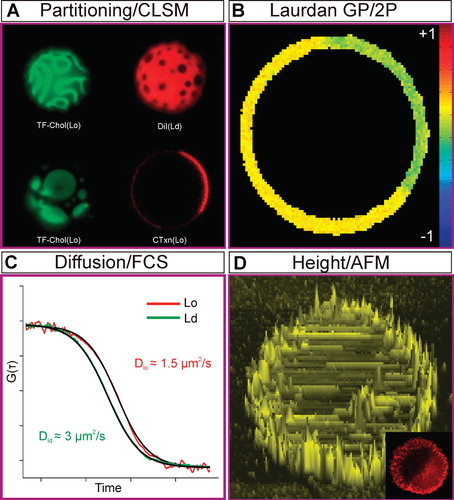
These systems, in particular the GPMVs, have been used to confirm and extend existing hypotheses about the roles of lipid modifications on membrane proteins, for instance (Levental et al. Citation2010a, Citation2010b, Thaa et al. Citation2011). Additionally, interactions between interleukin receptors and dimer formation of interleukin-4 receptor (Worch et al. Citation2010) were addressed using this system. Moreover, they have been beneficial to illuminate the physical packing and molecular composition of the cell membrane (Kaiser et al. Citation2009, Levental et al. Citation2011).
Outlook – the future of model membranes
As summarized in , there exist advantages and disadvantages of each membrane model system discussed above. Each system has its own difficulties and limitations and unfortunately, also produces its own artifacts. Moreover, all the model membranes mentioned above are at thermodynamic equilibrium, which does clearly not reflect the state of a living cell membrane. Similarly, membrane asymmetry, i.e., different compositions of the two opposing leaflets of a bilayer, is a central feature of biological membranes (Chiantia et al. Citation2011), while only the first steps have been taken to establish and investigate asymmetric model membranes (Collins and Keller Citation2008, Chiantia et al. Citation2011, Richmond et al. Citation2011) since the introduction of Montal-Muller membranes (Montal and Mueller Citation1972). Thus, the development and study of asymmetric membranes away from equilibrium presents the next challenge in the processive development toward truly biomimetic model membranes.
Table I. Pros and cons of model membranes.
Acknowledgements
We thank Grzegorz Chwastek and Fabian Heinemann for Atomic Force Microscopy images and Ilya Levental for his careful reading of and valuable comments on the manuscript. Financial support by the German Research Foundation (DFG Project Number SO-818/1-1 and SFB TRR 83/1-2010) is gratefully acknowledged.
Declaration of interest: The authors report no conflicts of interest. The authors alone are responsible for the content and writing of the paper.
References
- Aebersold R, Mann M. 2003. Mass spectrometry-based proteomics. Nature 422:98–207.
- Andersen OS, Koeppe RE II. 2007. Bilayer thickness and membrane protein function: An energetic perspective. Ann Rev Biophys Biomolec Struct 36:107–130.
- Angelova MI, Dimitrov DS. 1986. Liposome electroformation. Faraday Discuss 81:303–311.
- Angelova MI, Dimitrov DS. 1988. A mechanism of liposome electroformation. Prog Colloid Polymer Sci 76:59–67.
- Arumugam S, Chwastek G, Schwille P. 2011. Protein-membrane interactions: The virtue of minimal systems in systems biology. Wiley Interdisciplinary Rev-Syst Biol Med 3:269–280.
- Aussenac F, Tavares M, Dufourc EJ. 2003. Cholesterol dynamics in membranes of raft composition: A molecular point of view from H-2 and P-31 solid-state NMR. Biochemistry 42:1383–1390.
- Baatz JE, Elledge B, Whitsett JA. 1990. Surfactant protein SP-B induces ordering at the surface of model membrane bilayers. Biochemistry 29:6714–6720.
- Bacia K, Schuette CG, Kahya N, Jahn R, Schwille P. 2004. SNAREs prefer liquid-disordered over ‘raft' (liquid-ordered) domains when reconstituted into giant unilamellar vesicles. J Biol Chem 279:37951–37955.
- Bagatolli LA, Gratton E. 2000. Two photon fluorescence microscopy of coexisting lipid domains in giant unilamellar vesicles of binary phospholipid mixtures. Biophys J 78:290–305.
- Bagatolli LA, Sanchez SA, Hazlett T, Gratton E. 2003. Giant vesicles, laurdan, and two-photon fluorescence microscopy: Evidence of lipid lateral separation in bilayers. Biophotonics A 360:481–500.
- Bangham AD, Horne RW. 1964. Negative staining of phospholipids and their structural modification by surface-active agents as observed in the electron microscope. J Molec Biol 8:660–668.
- Basanez G, Zhang J, Chau BN, Maksaev G, Frolov V, Li HL, 2001. Proapoptotic proteins form large pores in pure lipid bilayers in a membrane curvature-dependent manner. Biophys J 80:238A.
- Baumgart T, Capraro BR, Zhu C, Das SL. 2011. Thermodynamics and mechanics of membrane curvature generation and sensing by proteins and lipids. Ann Rev Phys Chem 62:483–506.
- Baumgart T, Hammond AT, Sengupta P, Hess ST, Holowka DA, Baird BA, 2007. Large-scale fluid/fluid phase separation of proteins and lipids in giant plasma membrane vesicles. Proc Natl Acad Sci USA 104:3165–3170.
- Bhatia VK, Madsen KL, Bolinger P-Y, Kunding A, Hedegard P, Gether U, 2009. Amphipathic motifs in BAR domains are essential for membrane curvature sensing. Embo J 28:3303–3314.
- Biscari P, Bisi F, Rosso R. 2002. Curvature effects on membrane-mediated interactions of inclusions. J Mathemat Biol 45:37–56.
- Boehme R, Richter M, Cialla D, Roesch P, Deckert V, Popp J. 2009. Towards a specific characterisation of components on a cell surface – combined TERS-investigations of lipids and human cells. J Raman Spectroscopy 40:1452–1457.
- Brown DA, Rose JK. 1992. Sorting of GPI-anchored proteins to glycolipid-enriched membrane subdomains during transport to the apical cell surface. Cell 68:533–544.
- Cantor RS. 1997a. The lateral pressure profile in membranes: A physical mechanism of general anesthesia. Biochemistry 36:2339–2344.
- Cantor RS.. 1997b. Lateral pressures in cell membranes: A mechanism for modulation of protein function. J Phys Chem B 101:1723–1725.
- Chao H, Martin GG, Russell WK, Waghela SD, Russell DH, Schroeder F, 2002. Membrane charge and curvature determine interaction with acyl-CoA binding protein (ACBP) and fatty acyl-CoA targeting. Biochemistry 41:10540–10553.
- Charras GT, Coughlin M, Mitchison TJ, Mahadevan L. 2008. Life and times of a cellular bleb. Biophys J 94:1836–1853.
- Chiantia S, Kahya N, Schwille P. 2007. Raft domain reorganization driven by short- and long-chain ceramide: A combined AFM and FCS study. Langmuir 23:7659–7665.
- Chiantia S, Ries J, Chwastek G, Carrer D, Li Z, Bittman R, 2008. Role of ceramide in membrane protein organization investigated by combined AFM and FCS. Biochim Biophys Acta 1778:1356–1364.
- Chiantia S, Schwille P, Klymchenko AS, London E. 2011. Asymmetric GUVs prepared by M beta CD-mediated lipid exchange: An FCS study. Biophys J 100:LO1–LO3.
- Choi EJ, Dimitriadis EK. 2004. Cytochrome c adsorption to supported, anionic lipid bilayers studied via atomic force microscopy. Biophys J 87:3234–3241.
- Collins MD, Keller SL. 2008. Tuning lipid mixtures to induce or suppress domain formation across leaflets of unsupported asymmetric bilayers. Proc Natl Acad Sci USA 105:124–128.
- Coskun U, Grzybek M, Drechsel D, Simons K. 2011. Regulation of human EGF receptor by lipids. Proc Natl Acad Sci USA 108:9044–9048.
- Dietrich C, Bagatolli LA, Volovyk ZN, Thompson NL, Levi M, Jacobson K, 2001. Lipid rafts reconstituted in model membranes. Biophys J 80:1417–1428.
- Doeven MK, Folgering JHA, Krasnikov V, Geertsma ER, van den Bogaart G, Poolman B. 2005. Distribution, lateral mobility and function of membrane proteins incorporated into giant unilamellar vesicles. Biophys J 88:1134–1142.
- Ellena JF, Lackowicz P, Mongomery H, Cafiso DS. 2011. Membrane thickness varies around the circumference of the transmembrane protein BtuB. Biophys J 100:1280–1287.
- Estrada R, Puppato A, Borchman D, Yappert MC. 2010. Reevaluation of the phospholipid composition in membranes of adult human lenses by (31)P NMR and MALDI MS. Biochim Biophys Acta 1798:303–311.
- Fattal DR, Benshaul A. 1993. A molecular model for lipid-protein interaction in membranes: The role of hydrophobic mismatch. Biophys J 65:1795–1809.
- Fuertes G, Gimenez D, Esteban-Martin S, Garcia-Saez AJ, Sanchez O, Salgado J. 2010. Role of membrane lipids for the activity of pore forming peptides and proteins. Proteins: Membrane Binding and Pore Formation 677:31–55.
- Garcia-Saez AJ, Carrer DC, Schwille P. 2010. Fluorescence correlation spectroscopy for the study of membrane dynamics and organization in giant unilamellar vesicles. Methods Mol Biol 606:493–508.
- Garcia-Saez AJ, Ries J, Orzaez M, Perez-Paya E, Schwille P. 2009. Membrane promotes tBID interaction with BCL(XL). Nat Struct Mol Biol 16:1178–1185.
- Girard P, Pecreaux J, Lenoir G, Falson P, Rigaud JL, Bassereau P. 2004. A new method for the reconstitution of membrane proteins into giant unilamellar vesicles. Biophys J 87:419–429.
- Gorter E, Grendel F. 1925. On bimolecular layers of lipoids on the chromocytes of the blood. J Exp Med 41:439–443.
- Graham TR, Kozlov MM. 2010. Interplay of proteins and lipids in generating membrane curvature. Curr Opin Cell Biol 22:430–436.
- Han X, Bushweller JH, Cafiso DS, Tamm LK. 2001. Membrane structure and fusion-triggering conformational change of the fusion domain from influenza hemagglutinin. Nat Struct Biol 8:715–720.
- Hatzakis NS, Bhatia VK, Larsen J, Madsen KL, Bolinger P-Y, Kunding AH, 2009. How curved membranes recruit amphipathic helices and protein anchoring motifs. Nat Chem Biol 5:835–841.
- Heberle FA, Feigenson GW. 2011. Phase separation in lipid membranes. Cold Spring Harbor Perspect Biol 3.
- Heinemann F, Schwille P. 2011. Preparation of micrometer-sized free-standing membranes. ChemPhysChem 12:2568–2571.
- Hennesthal C, Drexler J, Steinem C. 2002. Membrane-suspended nanocompartments based on ordered pores in alumina. ChemPhysChem 3:885–889.
- Hirst DJ, Lee TH, Swann MJ, Unabia S, Park Y, Hahm KS, 2011. Effect of acyl chain structure and bilayer phase state on binding and penetration of a supported lipid bilayer by HPA3. Eur Biophys J Biophys Lett 40:503–514.
- Ho CY, Alghamdi TA, Botelho RJ. 2012. Phosphatidylinositol-3,5-Bisphosphate: No longer the poor PIP2. Traffic 13:1–8.
- Honigmann A, Walter C, Erdmann F, Eggeling C, Wagner R. 2010. Characterization of horizontal lipid bilayers as a model system to study lipid phase separation. Biophys J 98:2886–2894.
- Huang H, Ball JM, Billheimer JT, Schroeder F. 1999. Interaction of the N-terminus of sterol carrier protein 2 with membranes: Role of membrane curvature. Biochem J 344:593–603.
- Idiong G, Won A, Ruscito A, Leung BO, Hitchcock AP, Ianoul A. 2011. Investigating the effect of a single glycine to alanine substitution on interactions of antimicrobial peptide latarcin 2a with a lipid membrane. Eur Biophys J Biophys Lett 40:1087–1100.
- Jakop U, Fuchs B, Suess R, Wibbelt G, Braun B, Mueller K, 2009. The solubilisation of boar sperm membranes by different detergents – a microscopic, MALDI-TOF MS, (31)P NMR and PAGE study on membrane lysis, extraction efficiency, lipid and protein composition. Lipids Health Dis 8.
- Jalmar O, Garcia-Saez AJ, Berland L, Gonzalvez F, Petit PX. 2010. Giant unilamellar vesicles (GUVs) as a new tool for analysis of caspase-8/Bid-FL complex binding to cardiolipin and its functional activity. Cell Death Dis 1.
- Jensen MO, Mouritsen OG. 2004. Lipids do influence protein function – the hydrophobic matching hypothesis revisited. Biochim Biophys Acta 1666:205–226.
- Jin L, Millard AC, Wuskell JP, Clark HA, Loew LM. 2005. Cholesterol-enriched lipid domains can be visualized by di-4-ANEPPDHQ with linear and nonlinear optics. Biophys J 89:L4–L6.
- Johnson SA, Stinson BM, Go MS, Carmona LM, Reminick JI, Fang X, 2010. Temperature-dependent phase behavior and protein partioning in giant plasma membrane vesicles. Biochim Biophys Acta 1798:1427–1435.
- Juhasz J, Davis JH, Sharom FJ. 2010. Fluorescent probe partitioning in giant unilamellar vesicles of ‘lipid raft' mixtures. Biochem J 430:415–423.
- Kahya N, Brown DA, Schwille P. 2005. Raft partitioning and dynamic behavior of human placental alkaline phosphatase in giant unilamellar vesicles. Biochemistry 44:7479–7489.
- Kahya N, Pecheur EI, de Boeij WP, Wiersma DA, Hoekstra D. 2001. Reconstitution of membrane proteins into giant unilamellar vesicles via peptide-induced fusion. Biophys J 81:1464–1474.
- Kaiser H-J, Lingwood D, Levental I, Sampaio JL, Kalvodova L, Rajendran L, 2009. Order of lipid phases in model and plasma membranes. Proc Natl Acad Sci USA 106:16645–16650.
- Kaiser H-J, Orlowski A, Rog T, Nyholm TKM, Chai W, Feizi T, 2011. Lateral sorting in model membranes by cholesterol-mediated hydrophobic matching. Proc Natl Acad Sci USA 108:16628–16633.
- Kalvodova L, Kahya N, Schwille P, Ehehalt R, Verkade P, Drechsel D, 2005. Lipids as modulators of proteolytic activity of BACE – involvement of cholesterol, glycosphingolipids, and anionic phospholipids in vitro. J Biol Chem 280:36815–36823.
- Keller H, Lorizate M, Schwille P. 2009. PI(4,5)P-2 degradation promotes the formation of cytoskeleton-free model membrane systems. ChemPhysChem 10:2805–2812.
- Kim HM, Choo H-J, Jung S-Y, Ko Y-G, Park W-H, Jeon S-J, 2007. A two-photon fluorescent probe for lipid raft imaging: C-laurdan. ChemBioChem 8:553–559.
- Kim KS, Neu J, Oster G. 1998. Curvature-mediated interactions between membrane proteins. Biophys J 75:2274–2291.
- Korlach J, Schwille P, Webb WW, Feigenson GW. 1999. Characterization of lipid bilayer phases by confocal microscopy and fluorescence correlation spectroscopy. Proc Natl Acad Sci USA 96:8461–8466.
- Krishnan K, Holub O, Gratton E, Clayton AHA, Cody S, Moens PDJ. 2009. Profilin interaction with Phosphatidylinositol (4,5)-bisphosphate destabilizes the membrane of giant unilamellar vesicles. Biophys J 96:5112–5121.
- Langmuir I. 1917. The constitution and fundamental properties of solids and liquids. II. Liquids. J Am Chem Soc 39:1848–1906.
- Le Rumeur E, Pottier S, Da Costa G, Metzinger L, Mouret L, Rocher C, 2007. Binding of the dystrophin second repeat to membrane di-oleyl phospholipids is dependent upon lipid packing. Biochim Biophys Acta 1768:648–654.
- Levental I, Grzybek M, Simons K. 2010a. Greasing their way: Lipid modifications determine protein association with membrane rafts. Biochemistry 49:6305–6316.
- Levental I, Grzybek M, Simons K. 2011. Raft domains of variable properties and compositions in plasma membrane vesicles. Proc Natl Acad Sci USA 108:11411–11416.
- Levental I, Lingwood D, Grzybek M, Coskun U, Simons K. 2010b. Palmitoylation regulates raft affinity for the majority of integral raft proteins. Proc Natl Acad Sci USA 107:22050–22054.
- Lingwood D, Ries J, Schwille P, Simons K. 2008. Plasma membranes are poised for activation of raft phase coalescence at physiological temperature. Proc Natl Acad Sci USA 105:10005–10010.
- Lingwood D, Simons K. 2010. Lipid rafts as a membrane-organizing principle. Science 327:46–50.
- Loose M, Fischer-Friedrich E, Ries J, Kruse K, Schwille P. 2008. Spatial regulators for bacterial cell division self-organize into surface waves in vitro. Science 320:789–792.
- Loose M, Schwille P. 2009. Biomimetic membrane systems to study cellular organization. J Struct Biol 168:143–151.
- Madsen KL, Bhatia VK, Gether U, Stamou D. 2010. BAR domains, amphipathic helices and membrane-anchored proteins use the same mechanism to sense membrane curvature. Febs Lett 584:1848–1855.
- Marsh D.. 2007. Lateral pressure profile, spontaneous curvature frustration, and the incorporation and conformation of proteins in membranes. Biophys J 93:3884–3899.
- Marxer CG, Kraft ML, Weber PK, Hutcheon ID, Boxer SG. 2005. Supported membrane composition analysis by secondary ion mass spectrometry with high lateral resolution. Biophys J 88:2965–2975.
- McConnell HM, Tamm LK, Weis RM. 1984. Periodic structures in lipid monolayer phase transitions. Proc Natl Acad Sci USA 81:3249–3253.
- McMahon HT, Gallop JL. 2005. Membrane curvature and mechanisms of dynamic cell membrane remodelling. Nature 438:590–596.
- Milhiet PE, Giocondi MC, Baghdadi O, Ronzon F, Roux B, Le Grimellec C. 2002. Spontaneous insertion and partitioning of alkaline phosphatase into model lipid rafts. Embo Rep 3:485–490.
- Montal M, Mueller P. 1972. Formation of bimolecular membranes from lipid monolayers and a study of their electrical properties. Proc Natl Acad Sci USA 69:3561–3566.
- Morales-Penningston NF, Wu J, Farkas ER, Goh SL, Konyakhina TM, Zheng JY, 2010. GUV preparation and imaging: Minimizing artifacts. Biochim Biophys Acta 1798:1324–1332.
- Mouritsen OG. 2011. Model answers to lipid membrane questions. Cold Spring Harbor Perspect Biol 3.
- Mouritsen OG, Bloom M. 1984. Mattress model of lipid-protein interactions in membranes. Biophys J 46:141–153.
- Nikolaus J, Scolari S, Bayraktarov E, Jungnick N, Engel S, Plazzo AP, 2010. Hemagglutinin of influenza virus partitions into the nonraft domain of model membranes. Biophys J 99:489–498.
- Ollila OHS, Louhivuori M, Marrink SJ, Vattulainen I. 2011. Protein shape change has a major effect on the gating energy of a mechanosensitive channel. Biophys J 100:1651–1659.
- Overton E.. 1899. The probable origin and physiological significance of cellular osmotic properties. Vierteljahrschrift der Naturforschende Gesselschaft 44:88–135.
- Parasassi T, Krasnowska EK, Bagatolli L, Gratton E. 1998. LAURDAN and PRODAN as polarity-sensitive fluorescent membrane probes. J Fluoresc 8:365–373.
- Park RB. 1968. Translation of ‘The probable origin and physiological significance of cellular osmotic properties'. Biological Membrane Structure. Boston MA: Little, Brown & Co. pp 45–52.
- Parton DL, Klingelhoefer JW, Sansom MSP. 2011. Aggregation of model membrane proteins, modulated by hydrophobic mismatch, membrane curvature, and protein class. Biophys J 101:691–699.
- Pincelli MM, Levstein PR, Fidelio GD, Gennaro AM. 2000. Cholesterol-induced alterations of the packing properties of gangliosides: An EPR study. Chem Phys Lipids 104:193–206.
- Rao Y, Haucke V. 2011. Membrane shaping by the Bin/amphiphysin/Rvs (BAR) domain protein superfamily. Cell Mol Life Sci 68:3983–3993.
- Richmond DL, Schmid EM, Martens S, Stachowiak JC, Liska N, Fletcher DA. 2011. Forming giant vesicles with controlled membrane composition, asymmetry, and contents. Proc Natl Acad Sci USA 108:9431–9436.
- Richter RP, Berat R, Brisson AR. 2006. Formation of solid-supported lipid bilayers: An integrated view. Langmuir 22:3497–3505.
- Sampaio JL, Gerl MJ, Klose C, Ejsing CS, Beug H, Simons K, 2011. Membrane lipidome of an epithelial cell line. Proc Natl Acad Sci USA 108:1903–1907.
- Samsonov AV, Mihalyov I, Cohen FS. 2001. Characterization of cholesterol-sphingomyelin domains and their dynamics in bilayer membranes. Biophys J 81:1486–1500.
- Sanchez SA, Alejandra Tricerri M, Ossato G, Gratton E. 2010. Lipid packing determines protein-membrane interactions: Challenges for apolipoprotein A-I and high density lipoproteins. Biochim Biophys Acta 1798:1399–1408.
- Sanchez SA, Tricerri MA, Gratton E. 2012. Laurdan generalized polarization fluctuations measures membrane packing micro-heterogeneity in vivo. Proc Natl Acad Sci USA 109:7314–7319.
- Sarkis J, Hubert J-F, Legrand B, Robert E, Cheron A, Jardin J, 2011. Spectrin-like repeats 11–15 of human dystrophin show adaptations to a lipidic environment. J Biol Chem 286:30481–30491.
- Schaefer LV, de Jong DH, Holt A, Rzepiela AJ, de Vries AH, Poolman B, 2011. Lipid packing drives the segregation of transmembrane helices into disordered lipid domains in model membranes. Proc Natl Acad Sci USA 108:1343–1348.
- Schwille P. 2011. Bottom-up synthetic biology: Engineering in a tinkerer's world. Science 333:1252–1254.
- Schwille P, Diez S. 2009. Synthetic biology of minimal systems. Crit Rev Biochem Mol Biol 44:223–242.
- Scott RE. 1976. Plasma-membrane vesiculation – new technique for isolation of plasma-membranes. Science 194:743–745.
- Sengupta P, Hammond A, Holowka D, Baird B. 2008. Structural determinants for partitioning of lipids and proteins between coexisting fluid phases in giant plasma membrane vesicles. Biochim Biophys Acta 1778:20–32.
- Sezgin E, Kaiser H-J, Baumgart T, Schwille P, Simons K, Levental I. 2012a. Elucidating membrane structure and protein behavior using giant plasma membrane vesicles. Nat Protoc 7:1042–1051.
- Sezgin E, Levental I, Grzybek M, Schwarzmann G, Mueller V, Honigmann A, 2012b. Partitioning, diffusion, and ligand binding of raft lipid analogs in model and cellular plasma membranes. Biochim Biophys Acta 1818:1777–1784.
- Sezgin E, Schwille P. 2011. Fluorescence techniques to study lipid dynamics. Cold Spring Harb Perspect Biol 3.
- Simon A, Girard-Egrot A, Sauter F, Pudda C, D'Hahan NP, Blum L, 2007. Formation and stability of a suspended biomimetic lipid bilayer on silicon submicrometer-sized pores. J Colloid Interface Sci 308:337–343.
- Simons K, Gerl MJ. 2010. Revitalizing membrane rafts: New tools and insights. Nat Rev Mol Cell Biol 11:688–699.
- Simons K, Ikonen E. 1997. Functional rafts in cell membranes. Nature 387:569–572.
- Simons K, Sampaio JL. 2011. Membrane organization and lipid rafts. Cold Spring Harbor Perspect Biol 3.
- Simonsen AC, Bagatolli LA. 2004. Structure of spin-coated lipid films and domain formation in supported membranes formed by hydration. Langmuir 20:9720–9728.
- Singer SJ, Nicolson GL. 1972. The fluid mosaic model of the structure of cell membranes. Science 175:720–731.
- Tamm LK, McConnell HM. 1985. Supported phospholipid-bilayers. Biophys J 47:105–113.
- Tanford C. 2004. Ben Franklin stilled the waves: An informal history of pouring oil on water with reflections on the ups and downs of scientific life in general. New York: Oxford University Press.
- Thaa B, Levental I, Herrmann A, Veit M. 2011. Intrinsic membrane association of the cytoplasmic tail of influenza virus M2 protein and lateral membrane sorting regulated by cholesterol binding and palmitoylation. Biochem J 437:389–397.
- Vanmeer G, Stelzer EHK, Wijnaendtsvanresandt RW, Simons K. 1987. Sorting of sphingolipids in epithelial (Madin-Darby canine kidney) cells. J Cell Biol 105:1623–1635.
- Vogel A, Katzka CP, Waldmann H, Arnold K, Brown MF, Huster D. 2005. Lipid modifications of a Ras peptide exhibit altered packing and mobility versus host membrane as detected by 2H solid-state NMR. J Am Chem Soc 127:12263–12272.
- Wagner ML, Tamm LK. 2000. Tethered polymer-supported planar lipid bilayers for reconstitution of integral membrane proteins: Silane-polyethyleneglycol-lipid as a cushion and covalent linker. Biophys J 79:1400–1414.
- Worch R, Bokel C, Hofinger S, Schwille P, Weidemann T. 2010. Focus on composition and interaction potential of single-pass transmembrane domains. Proteomics 10:4196–4208.
- Wright LC, NouriSorkhabi MH, May GL, Danckwerts LS, Kuchel PW, Sorrell TC. 1997. Changes in cellular and plasma membrane phospholipid composition after lipopolysaccharide stimulation of human neutrophils, studied by P-31 NMR. Eur J Biochem 243:328–335.
- Yu J, Fischman DA, Steck TL. 1973. Selective solubilization of proteins and phospholipids from red blood cell membranes by nonionic detergents. J Supramol Struct 1:233–248.