Abstract
Steady-state fluorescence anisotropy and dynamic light scattering (DLS) were used to determine the thermotropic properties of lipid systems that act as models for bacterial membranes of Yersinia kristensenii and Proteus mirabilis. Lipid proportions of PE:PG:CL of 0.60:0.20:0.20 and 0.80:0.15:0.05, were used in order to mimic these two membranes respectively. We observed that the introduction of cardiolipin (CL) as a third lipid component of any PE:PG mixture, changes the system's properties considerably. The results obtained by these two techniques show that the main transition temperatures obtained are undoubtedly CL-dependent. Additionally AFM experiments were performed and these results show that even at small concentration CL produces important changes not only in the membrane thermotropic properties, but also in the bilayer structure. In summary, we were able to compare how low and high CL concentration affect bacterial membrane model system properties which can provide a further explanation for the different antibiotic susceptibilities reported for Y. kristensenii and P. mirabilis.
Introduction
Recently, much research has focused on bacterial membranes because antibiotic resistance is becoming an urgent public health problem and several antimicrobial agents were found to destabilize and destroy bacteria cells while leaving mammalian cells unaffected (Lohner Citation2001, Citation2009, Arouri et al. Citation2009, Hurdle et al. Citation2011). Lipid membrane composition appears to be the key to the ability of these agents to discriminate between microbial and mammalian cells. Membrane composition seems therefore to be one of the keys to the rational development of new antimicrobial agents to fight infectious diseases, and a current trend in antibiotic research is targeting of bacterial membranes in order to destabilize them or to induce the formation of membrane domains (Lohner Citation2001, Gong et al. Citation2008, Findlay et al. Citation2010, Huang et al. Citation2010, Epand and Epand Citation2011, Hurdle et al. Citation2011).
The mechanisms of action proposed for several classes of antimicrobial agents are membrane-related and involve phenomena such as membrane damage by pore formation (Leontiadou et al. Citation2006, Frantz et al. Citation2008, Bechinger Citation2010), lipid clustering (Epand et al. 2010a, 2010b, Polyansky et al. Citation2010), membrane disruption (Lohner Citation2009, Nicolas Citation2009, Findlay et al. Citation2010, Hurdle et al. Citation2011, Pathan et al. Citation2010) or membrane depolarization (Epand et al. 2010). Independently of an unambiguous understanding of the mechanisms of action of antimicrobial agents, the bacterial membrane poses a barrier and is therefore an essential site of interaction that needs to be either overcome or destroyed (Lohner and Blondelle Citation2005). Furthermore, one of the major factors that allow antimicrobial agents to discriminate between bacterial and mammalian cells is thought to be their different membrane lipid composition and consequently physical properties (Garidel and Blume Citation2000, Pozo Navas et al. Citation2005, Sanderson Citation2005, Hale and Hancock Citation2007, Ding et al. Citation2008, Lohner et al. Citation2008, Epand and Epand 2009a, 2009b). Mammalian cytoplasmic membranes contain mainly phosphatidylcholine (PC), sphingomyelin, phosphatidylserine, phosphatidylethanolamine (PE) and cholesterol (Yeagle Citation1987, Findlay and Evans Citation1990). Bacterial membranes, on the other hand, contain to a large extent PE, phosphatidylglycerol (PG) and cardiolipin (CL), but lack cholesterol (Epand and Epand 2009a, 2009b). Additionally, all bacterial membranes have anionic lipids in their composition which can be either PG or CL, or a mixture of both.
It is the exposure of these anionic lipids that seems to explain the selective toxicity of some antimicrobial agents against bacteria but not against mammalian cells (Hale and Hancock Citation2007). Moreover, different lipid composition alters global membrane properties, such as structure, packing density, surface charge density, among others (Yeagle Citation1987, Findlay and Evans Citation1990, Lopes et al. Citation2010). Although mixtures of PE and PG have been the most commonly used model system for bacterial membranes recently, as a consequence of cardiolipin's importance (Hoch Citation1992, Haines Citation2009, Mileykovskaya and Dowhan Citation2009, Romantsov et al. Citation2009, Schlame and Ren Citation2009, Raja Citation2010), namely in bacterial membrane properties (Mileykovskaya and Dowhan Citation2009, Romantsov et al. Citation2009), some studies have been made with binary lipid mixtures in which one of the components is CL (Lewis and McElhaney Citation2009, Epand et al. 2010a, Prossnigg et al. Citation2010, Cheng et al. Citation2011, Frias et al. Citation2011). The results reported show considerable impact on the bacterial model membrane properties when CL is added. Nevertheless, studies with mimetic systems that take into consideration the three main components of bacterial membranes are rather scarce (Domènech et al. 2007c, Hadjicharalambous et al. Citation2008) and, to the best of our knowledge, only one biophysical study of thermotropic properties has been carried out to elucidate the properties of a model system composed of 1-palmitoyl-2-oleoyl-sn-glycero-3-phosphoethanolamine/1-palmitoyl-2-oleoyl-sn-glycero-3-phosphoglycerol: cardiolipin (POPE:POPG:CL) (Lopes et al. Citation2010). Taking into account that: (1) Membrane properties are directly related with the proposed mechanisms of action of several antimicrobial agents (Bechinger and Lohner Citation2006, Sevcsik et al. Citation2007, Dennison et al. Citation2008, Epand et al. Citation2008), and (2) that an important current trend research is focusing on antimicrobial agents to specifically target bacterial membranes (Radzishevsky et al. Citation2007, Chongsiriwatana et al. Citation2008, Epand et al. Citation2008, Rotem et al. Citation2008) it seems therefore important to use mimetic systems close to those that exist in nature.
In this work, steady-state fluorescence anisotropy and dynamic light scattering (DLS) were used to determine lipid phase transition of systems that act as models for bacterial membranes of Yersinia kristensenii and Proteus mirabilis which have reported lipid proportions of PE:PG:CL of 0.60:0.20:0.20 and 0.80:0.15:0.05, respectively (Epand and Epand 2009a). Y. kristensendii is a Yersinia species and has been isolated from various environmental samples (fresh water, soil, etc.), foods, animals and both healthy and sick humans (Sulakvelidze Citation2000). Its pathogenicity to humans is still uncertain; however, it has been shown that in some conditions it is pathogenic in mice, leading to death (Robins-Browne et al. Citation1991). On the other hand P. mirabilis is a bacteria species which is an opportunistic human pathogen, responsible for many human urinary tract infections (Guentzel Citation1996). These bacteria present different membrane lipid composition and so different susceptibility profiles have been obtained for several antibiotic classes in studies comparing Escherichia coli, P. mirabilis and Y. kristensenii (Naber et al. Citation2000, Stock and Wiedemann Citation2003, Hawser et al. Citation2011). While Y. kristensenii has been reported as being naturally sensitive to ceftazidime, ampicillin, ciprofloxacin, norfloxacin (Stock and Wiedemann Citation2003), P. mirabilis presents higher sensitivity to cefepime, ertapenem and piperacilin/tazobactam (Hawser et al. Citation2011). Moreover, when studies are performed for groups of antibiotics to which both E. coli and P. mirabilis are susceptible, very different minimal inhibitory concentrations (MICs) are obtained for each bacteria (Naber et al. Citation2000, Adam et al. Citation2009). Furthermore, bacteria are known to be able to adjust their relative CL, PE and PG concentrations under osmotic stress conditions, further reason to study how changing PE:PG:CL relative concentrations affect model lipid membranes (Hoch Citation1992, Weber and de Bont Citation1996, Mileykovskaya Citation2007, Romantsov et al. Citation2009).
Main transition temperatures were determined by steady-state fluorescence anisotropy using two fluorescent probes that report different membrane regions (TMA-DPH and DPH), and DLS as proposed by Michel et al. (Citation2006). Fluorescence anisotropy is an invasive technique where two probes are used to measure changes in membrane fluidity, and which is commonly used to determine phase transitions in liposomes (Esquembre et al. Citation2007, Halling et al. Citation2008). DLS, a non-invasive technique, takes into account that a phase transition induces modifications in the system's optical properties. Such changes affect the measured scattering intensity, reported here as mean count rate. Thus, although the phases themselves cannot be detected by this technique, phase transitions can induce a change in the mean count rate. The results obtained show that the number of transitions and the temperature values obtained for the different transition temperatures from the two techniques are identical, within experimental error. Additionally Atomic Force Microscopy (AFM) experiments were performed, a technique that allows examination of hydrated surfaces morphology with nanometer-level resolution (Frederix et al. Citation2009, Spyratou et al. Citation2009, Sitterberg et al. Citation2010). The results clearly show that different POPE:POPG:CL proportions have a dramatic effect on the model system membrane properties. These results have important implications for validation of the most common PE:PG model bacteria membrane system used in the literature (Garidel and Blume Citation2000, Pozo Navas et al. Citation2005, Zhao et al. Citation2008, Arouri et al. Citation2009, Wydro and Witkowska Citation2009, Oszlánczi et al. Citation2010).
Materials and methods
N-(2-hydroxyethyl) piperazine-N′-ethanesulfonic acid (HEPES) was purchased from Sigma. 1,6-diphenyl-1,3,5-hexatriene (DPH) and trimethylammonium-diphenylhexa-triene (TMA-DPH) were also from Sigma, POPE, POPG and CL (natural extract from E. coli) were from Avanti Polar Lipids. The nominal fatty acid composition of CL was 14:0 (2.2%), 15:0 (4.8%), 16:0 (33.3%), 16:1 (10.2%), 17:0 (1.4%), cyclo 17:0 (27.0%), trans 18:1 (14.4%), 19:0 (5.3%) and unknown (1.4%) (Avanti Polar Lipids 2010 http://www.avantilipids.com). All other chemicals were from Merck. All solutions were prepared with 10 mM HEPES buffer (0.1 M NaCl; pH 7.4).
Liposome preparation
Chloroform solutions for POPE:POPG:CL mixtures containing the appropriate amount of lipids were dried under a stream of argon. The thin film obtained was kept under high vacuum for more than 3 h to remove organic solvent traces. Multilamellar vesicles (MLVs) were obtained after redispersion of the film in 10 mM HEPES buffer (0.1 M NaCl, pH 7.4) and vortexed above the phase transition temperature. Frozen and thawed MLVs were obtained by repeating five times the following cycle: freezing the vesicles in liquid nitrogen and thawing the sample in a water bath. Suspensions of MLVs were then extruded 10 times, on a Lipex Biomembranes extruder attached to a circulating water bath, through polycarbonate filters (100 nm) to produce large unilamellar vesicles (LUVs) for POPE:POPG:CL All lipid ratios presented in this work are molar lipid ratios.
Steady state anisotropy experiments
Fluorescence measurements were performed in a Varian Cary Eclipse spectrofluorometer, equipped with a constant-temperature cell holder (Peltier Multicell Holder, Cary Temperature Probe series II). The structural order of lipid membrane systems under study were investigated by measuring, as a function of temperature, the steady-state fluorescence anisotropy (rs) of DPH (1,6-phenyl-1,3,5-hexatriene) and TMA-DPH (1-(4-trimethylammoniumphenyl)-6-phenyl-1,3,5-hexatriene) incorporated into liposomes of POPE:POPG:CL phospholipid mixtures. The suspensions of liposomes were mixed with TMA-DPH or DPH for 30 min, to a final lipid-to-fluorescent probe ratio of 300:1 (mol/mol). The excitation and emission wavelengths used were 355/426 nm and 336/427 nm for TMA-DPH and DPH, respectively. The steady-state fluorescent anisotropy was recorded at 3°C intervals for all studied systems, using both probes, in the range of temperature from 3–80°C. Samples were left to equilibrate five minutes at each temperature, after the temperature was increased. Five independent experiments were performed for each system.
The steady state fluorescence anisotropy (rs) is defined by the following equation (Lakowicz Citation1999):
where IVV and IVH are the intensities measured in directions parallel and perpendicular to the excitation beam. The correction factor G is the ratio of the detection system sensitivity for vertically and horizontally polarized light, which is given by the ratio of vertical to horizontal components when the excitation light is polarized in the horizontal direction, G = IHV/IHH (Lakowicz Citation1999).
The typical temperature dependence of DPH and TMA-DPH anisotropy in liposomes are depicted in . The following equation was fitted to the anisotropy versus temperature data
Figure 1. Steady-state fluorescence anisotropy, using TMA-DPH and DPH, and dynamic light scattering results showing the thermotropic lipid phase transitions of POPE:POPG:CL liposomes in HEPES buffer 10 mM, pH 7.4, 0.1 M NaCl, for two different lipid ratios.
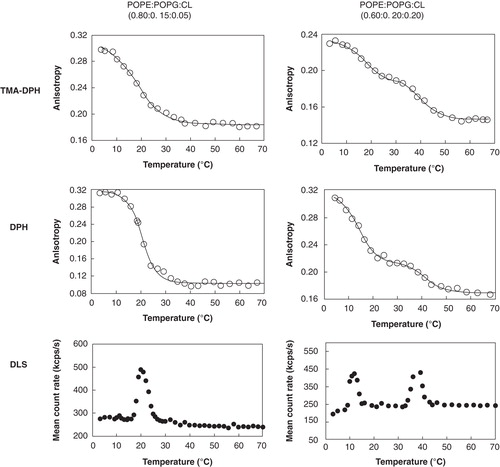
where T is the absolute temperature, Tm is the midpoint phase transition and rs1 and rs2 are the upper and lower values of rs; B′ is the slope factor which is correlated with the extent of cooperativity (B) by B= [1-1/(1+B′)]; the introduction of B yields a convenient scale of cooperativity ranging from 0–1 (Merino-Montero et al. Citation2006).
Dynamic light scattering
A Zetasizer Nano ZS dynamic light scattering instrument (Malvern, UK) was used to perform size distribution and mean count rate measurements. The methodology described by Michel et al. (Citation2006) and already tested (Lopes et al. Citation2010) was adopted to determine transition temperature of the lipidic systems under study with small modifications. These small changes were the following: (1) Measurements were performed in the temperature range from 3–70°C, with temperature steps of 1–3°C; (2) samples were left to equilibrate 5 min at each temperature, and each point was measured repeatedly so as to achieve accurate reproducibility in the intensity of scattered light. Data were collected as ‘mean count rate versus temperature' or ‘normalized mean count rate versus temperature' for comparison purposes and treated with Boltzmann regression curves
with A1, the initial y value (initial count rate of lipids in Phase 1), A2, the final y value (lipids in Phase 2), x0, the center of the distribution (y value at x0 being half way between the two limiting values A1 and A2: y(x0) = (A1 + A2)/2) and x, the width of the slope.
The mean count rate (average number of photons detected per second) is representative of an emerging macroscopic phenomenon, which is not directly size-dependent, in which changes in the measured scattering intensity reflect changes in the optical properties of the material. Therefore, discontinuity in the mean count rate as the temperature is altered, corresponds to a change in the optical properties of the material studied (i.e., transition from one state to another) (Michel et al. Citation2006).
Atomic Force Microscopy (AFM) experiments
Samples for AFM were prepared by depositing a 20 μl volume of the liposome solution in HEPES buffer on freshly cleaved mica at room temperature. Twenty minutes was allowed for the vesicles to fuse, and then the sample was washed three times with fresh buffer, finally leaving a volume of around 15 μl in which to image. Imaging was carried out at room temperature in buffer using tapping mode. NP-S probes with a spring constant of approximately 0.06 N/m (Veeco, CA, USA) were used at an oscillation frequency of approximately 8 kHz. All imaging was carried out with a multimode AFM and NanoscopeIVa electronics (Veeco). In all cases, the presence of the lipid bilayer (which was often featureless) was verified by pushing the AFM tip through the layer using the breakthrough technique described in reference (Connell and Smith Citation2006). Although we were unable to control the temperature with our AFM set-up, we measured the temperature in the liquid drop above the sample during a typical experiment and found it varied from 26–27°C.
Results and discussion
Steady-state fluorescence anisotropy is a broadly used technique to study changes in lipid environments, using fluorescent probes that partition preferentially to the lipid phase and with negligible fluorescence in water (Lakowicz Citation1999, Trevors Citation2003, Marczak Citation2009). TMA-DPH and DPH were the fluorescent probes chosen since their biophysical characteristics are well known and they have been extensively used to detect lipid phase transitions, independently of which phase is under study (van Langen et al. Citation1989, Basanez et al. Citation1996, Repáková et al. Citation2005, M'Baye et al. Citation2008). On the other hand, DLS is a non-invasive optical technique that can detect lipid phase transitions and accurate transition temperatures (Michel et al. Citation2006).
and summarize the results obtained in this work and it is clear that similar results, within experimental error, are obtained by the two fluorescent probes used in the steady-state fluorescence measurements, which are in agreement with the results obtained by DLS.
Table I. Mean lipid transition temperatures (Tm/°C) obtained for the different lipid systems studied, using TMA-DPH and DPH steady-state fluorescence anisotropy, and by dynamic light scattering (DLS) methodology described by Michel et al. (Citation2006). All lipid mixtures were studied in 10 mM HEPES buffer, pH 7.4, NaCl 0.1 M. The values represent the mean value of the five independent measures.
Although mixtures of PE and PG have been the most commonly used model system for bacterial membranes, they lack CL, one important bacterial membrane compound. Cardiolipins constitute a class of lipids that is, in many ways, exceptional, not only for its peculiar structure (they are divalent anionic lipids with four acyl chains) but also due to its variability, in percentage and organism, of its acyl chains. Therefore, different lipid molar ratios of POPE:POPG:CL can significantly affect not only the obvious lipid water interface properties and the overall membrane charge, but also the thermotropic properties of these model systems of bacterial membranes. Moreover different species of the same lipid group are expected to also influence these properties. The CL we used, from a bacterial natural extract commercially available, is in fact a mixture of different CL molecular species, as stated before in the Materials and methods section. This mixture presents CL molecular species with different nominal fatty acid composition with different transition temperatures which are described in (also see references therein).
Table II. Different molecular species present in the cardiolipin mixture from E. coli extract.
For the POPE:POPG:CL (0.80:0.15:0.05) system only one single transition temperature was obtained, in the range 19–21°C. Nevertheless, two transition temperatures were found for the POPE:POPG:CL (0.60:0.20:0.20) system: The first transition temperature is observed in the range 12–15°C, and the second one in the range 40–44°C.
The transition temperature value obtained for the POPE:POPG:CL (0.80:0.15:0.05) system was lower than the transition temperature reported for the binary POPE:POPG system in a 0.82:0.18 molar proportion (Pozo Navas et al. Citation2005), 22.7°C, but higher than the transition temperature obtained for POPE:CL in a 0.80:0.20 molar proportion, Tm = 15.2°C (Domènech et al. 2007b).
For the POPE:POPG:CL system in 0.60:0.20:0.20 molar proportions, the first observed transition temperature was ≈ 14°C. This transition temperature is lower than that reported for the system POPE:POPG (0.60:0.40), which was ≈ 20°C (Pozo Navas et al. Citation2005, Lopes et al. Citation2010). However, results reported for POPE/CL mixtures (Domènech et al. 2007b, Epand et al. Citation2007) suggest that cardiolipin's presence in the POPE environment leads to a decrease in transition temperature compared with the pure POPE, reported as 24.7–27.1°C. In fact, Domènech et al. (2007b) found a Tm of 15.2°C for a POPE:CL system with a 0.8:0.2, while Epand et al. (Citation2007) found a Tm of 12°C for the same system with 0.75:0.25 molar proportion, showing that CL does indeed decrease the Tm considerably. Epand et al. (Citation2007) and Domènech et al. (2007) used 1,1′2,2′-tetraoleoyl cardiolipin (TOCL) and therefore the observed decrease of the POPE transition temperature was due to the 25% TOCL incorporated. Since we worked with a mixture of CL species in which TOCL was present at 14.4% (see ) and we also observed a decrease in the first transition temperature recovered, we think it is fair to discuss that this might also be due to cardiolipin's presence. Moreover, it seems likely that the lowest transition temperature obtained for the POPE:POPG:CL (60:20:20) (≈ 14°C) is mainly governed by POPE–CL interactions. The higher transition temperature observed (≈ 42°C), for this system, most probably derives from some of the molecular cardiolipin's species presence at this high concentration, the ones with higher temperature transitions (see ), since according to the literature (Yokota et al. Citation1980, Lewis and McElhaney Citation2009, Avanti Polar Lipids 2010), the transition temperatures of the main cardiolipin derivatives presented in our mixture can vary in the range 35–62°C depending on their structure. Therefore, this higher transition should be mainly influenced by cardiolipin's presence. Moreover this higher transition temperature was already observed for a ternary POPE:POPG:CL system in a similar molar ratio to the one present in the E. coli membrane (Lopes et al. Citation2010).
Mixtures of PEs and PGs, whether saturated or unsaturated, and their overall characteristics as well as their thermodynamic mixing properties have been extensively studied (Garidel and Blume Citation2000, Lohner et al. Citation2001, Pozo Navas et al. Citation2005, Arouri et al. Citation2009). The POPE:POPG phase diagram, under physiologically relevant conditions, showed that the thermodynamic mixing properties are dominated by electrostatic forces at the headgroup and are largely independent of the hydrocarbon's chain composition (Pozo Navas et al. Citation2005). Pure POPE or POPG have a transition temperature of 24.7°C and −5.3°C, respectively (Pozo Navas et al. Citation2005).
PEs are zwitterionic amphiphiles and, in the fluid phase, their polar headgroup has a smaller diameter than the hydrocarbon chain region which results in the molecular shape of a truncated cone and an intrinsic propensity of PE bilayers to form surfaces with a negative curvature, leading to the formation of non-lamellar phases at elevated temperatures (Gunstone et al. Citation1994). PGs, in contrast, are negatively charged at pH > 5 and because of their cylindrical molecular shape, they prefer to form flat bilayers even at high temperatures (Huang and Li Citation1999, Degovics et al. Citation2000, Riske et al. Citation2004).
CL, the third main component of bacterial lipid membranes, is a doubly negatively charged four-tailed phospholipid with a polar headgroup, which is relatively rigid (i.e., it is a mobility-restricted entity) with a relative small cross-sectional area per lipid and, unlike zwitterionic lipids, it cannot shield its charges by rearranging itself (Dahlberg and Maliniak Citation2008). These different structural characteristics undoubtedly change the surface properties of model system membranes compared to those composed only of POPE:POPG and the results obtained in this work confirm this behavior. All our results show that the lowest transition temperature obtained for both systems are mainly governed by CL interactions.
Further characterization of these systems was made by AFM at room temperature (26–27°C approximately). This technique allows the study of the dimension, heterogeneity and distribution of supported lipid bilayers. Planar structures were obtained and the existence of a membrane layer was verified as described in the experimental section. shows AFM images obtained for POPE:POPG:CL (0.80:0.15:0.05). The mean height of the bilayer obtained is 4.0 ± 0.1 nm. This value is much smaller than the reported values obtained for POPE:POPG binary systems, which are ≈ 4.7 nm and ≈ 4.6 nm for POPE:POPG (0.6:0.4) (Lopes et al. Citation2010) and POPE:POPG (0.75:0.25) (Doménech et al. 2006), respectively. Moreover this result is also very different from the reported value for POPE:CL (0.8:0.2) which exhibit domains in a height range of 4.7–6.6 nm (Doménech et al. 2007a, 2007b). Nevertheless, the obtained value is similar to the one reported for the ternary system POPE:POPG:CL in E. coli lipid membrane observed proportions (0.67:0.23:0.10) ≈ 3.9 nm (Lopes et al. Citation2010). This result shows, therefore, that even a small percentage of CL produces important changes not only the membrane thermotropic properties, but also in the bilayer structure.
Figure 2. Atomic Force Microscopy image of bilayer produced from POPE:POPG:CL (0.80:0.15:0.05) liposomes, in HEPES buffer 10 mM, pH 7.4, 0.1 M NaCl at 26°C. The dashed line in the image (left) indicates the location of the height profile (right).
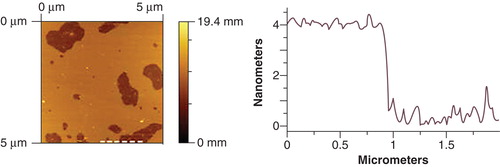
Unlike the POPE:POPG:CL (0.80:0.15:0.05) system, we found that it was not possible to obtain reproducible AFM data for the POPE:POPG:CL (0.60:0.20:0.20) system. We repeated these experiments several times, but consistently found that while the system with the lower proportion of CL gave reproducible results, the results found with 20% CL varied wildly from one experiment to another. Complex mixtures of lipids like this one, where there is a high CL concentration together with a high overall negative surface charge can support multiple co-existing phases, thus increasing the system heterogeneity. This can result in a system with highly heterogeneous molecular ordering which we consider to be the most probable cause of the difficulties we found in obtaining reproducible results. To our best knowledge although there are several AFM published results for POPE:POPG and POPE:CL systems (Domènech et al. 2007a, 2007b, Citation2009, Citation2010, Picas et al. Citation2009), there remains only one previously published paper describing AFM imaging of a ternary system that combines POPE, POPG and CL, in which similar results to ours from the POPE:POPG:CL (0.80:0.15:0.05) system were found (Lopes et al. Citation2010). Overall, these AFM results clearly show that the presence of CL has a crucial effect on the properties of model membrane system of bacterial membranes.
Conclusions
The two techniques, namely DLS and fluorescence anisotropy, are simple to implement and suitable to study lipid systems. The results were reproducible and the values obtained from the two techniques were the same, within experimental errors, showing that either technique can be used to obtain accurate values to characterize the thermotropic behavior of such systems. With this study we have shown by three different techniques, which are not usually combined in these kinds of studies, how CL introduction in bacterial membrane model systems impacts on thermotropic lipid behavior even if a CL small percentage is added. A 22.7°C transition temperature was reported by Pozo Navas et al. (Citation2005) for a POPE:POPG (0.82:0.18) in contrast with a 19.2°C transition temperature for our POPE:POPG:CL (0.80:0.15:0.05). Moreover the AFM experiment also showed that even a small percentage of CL greatly reduces reduced the mean height of the bilayer, which for our POPE:POPG:CL (0.80:0.15:0.05) was 4.0 ± 0.1 nm and for the published studies for POPE:POPG mixtures (in proportions so different as 0.6:0.4 and 0.75:0.25) were all very similar and about 4.7 nm. Furthermore, using a higher percentage of CL showed that we have observed an additional transition temperature at higher temperatures.
Lipid phase transitions are known to influence protein redistribution (Seeger et al. Citation2009), and CL has been shown to have a strong influence on membrane organization, and therefore affects protein-lipid interactions and membrane active molecules (Domènech et al. 2007a, 2007c, Epand and Epand 2009a, 2009b). Thus it is fair to say that CL is a crucial element when trying to approach a more accurate model system for bacteria membranes. This work further supports this finding, clearly showing that even in small proportion, CL affects lipids thermotropic behavior. The comparison of the data obtained in this work with values reported in literature show that the main transition temperatures obtained are undoubtedly CL-dependent ().
Table III. Comparison of the first transition temperature (Tm/°C) obtained for systems containing different POPE/POPG/CL proportions.
Due to the small sampling size, AFM may not be suitable to study naturally derived mixtures of lipids. However, reproducible results were obtained in the case of the synthetic model POPE:POPG:CL (0.80:0.15:0.05), which further reinforced the considerable difference that addition of CL makes to the systems.
Taken all together, we were able to compare how low and high CL concentration affect bacterial membrane model system properties; moreover, these results show that since membrane properties are a key feature for the understanding of drug mechanisms of action, better model membranes should be used, in order to avoid the risk of obtaining conclusions not relevant to real systems.
Acknowledgements
Partial financial support for this work was provided by FCT PTDC/SAU-FAR/111414/2009. This work has also been supported by Fundaçãopara a Ciência e a Tecnologia through grant no. PEst-C/EQB/LA0006/2011. S. C. Lopes and C. Neves thank FCT for a SFRH/BPD/34262/2006 and SFRH/BD/61137/2009 fellowship, respectively.
Declaration of interest: The authors report no conflicts of interest. The authors alone are responsible for the content and writing of the paper.
References
- Adam HJ, Laing NM, King CR, Lulashnyk B, Hoban DJ, Zhanel GG. 2009. In vitro activity of Nemonoxacin, a novel nonfluorinated quinolone, against 2,440 clinical isolates. Antimicrob Agents Chemother 53:4915–4920.
- Arouri A, Dathe M, Blume A. 2009. Peptide induced demixing in PG/PE lipid mixtures: A mechanism for the specificity of antimicrobial peptides towards bacterial membranes?Biochim Biophys Acta 1788:650–659.
- Basanez G, Nieva JL, Rivas E, Alonso A, Goni FM. 1996. Diacylglycerol and the promotion of lamellar-hexagonal and lamellar-isotropic phase transitions in lipids: implications for membrane fusion. Biophys J 70:2299–2306.
- Bechinger B. 2010. Membrane association and pore formation by alpha-helical peptides. Adv Exp Med Biol 677:24–30.
- Bechinger B, Lohner K. 2006. Detergent-like actions of linear amphipathic cationic antimicrobial peptides. Biochim Biophys Acta 1758:1529–1539.
- Cheng JTJ, Hale JD, Elliott M, Hancock REW, Straus SK. 2011. The importance of bacterial membrane composition in the structure and function of aurein 2.2 and selected variants. Biochim Biophys Acta 1808:622–633.
- Chongsiriwatana NP, Patch JA, Czyzewski AM, Dohm MT, Ivankin A, Gidalevitz D, 2008. Peptoids that mimic the structure, function, and mechanism of helical antimicrobial peptides. Proc Natl Acad Sci USA 105:2794–2799.
- Connell SD, Smith DA. 2006. The atomic force microscope as a tool for studying phase separation in lipid membranes (Review). Mol Membr Biol 23:17–28.
- Dahlberg M, Maliniak A. 2008. Molecular dynamics simulations of cardiolipin bilayers. J Phys Chem B 112:11655–11663.
- Degovics G, Latal A, Lohner K. 2000. X-ray studies on aqueous dispersions of dipalmitoyl phosphotidylglycerol in the presence of salt. J Appl Cryst 33:544–547.
- Dennison SR, Morton LHG, Harris F, Phoenix DA. 2008. The impact of membrane lipid composition on antimicrobial function of an [alpha]-helical peptide. Chem Phys Lipids 151:92–102.
- Ding J, Li P, Ho B. 2008. The Sushi peptides: Structural characterization and mode of action against Gram-negative bacteria. Cell Mol Life Sci 65:1202–1219.
- Domènech O, Dufrêne YF, Van Bambeke F, Tukens PM, Mingeot-Leclercq M-P. 2010. Interactions of oritavancin, a new semi-synthetic lipoglycopeptide, with lipids extracted from Staphylococcus aureus. Biochim Biophys Acta 1798:1876–1885.
- Domènech O, Francius G, Tulkens PM, Van Bambeke F, Dufrêne Y, Mingeot-Leclercq M-P. 2009. Interactions of oritavancin, a new lipoglycopeptide derived from vancomycin, with phospholipid bilayers: Effect on membrane permeability and nanoscale lipid membrane organization. Biochim Biophys Acta 1788:1832–1840.
- Domènech Ò, Merino-Montero S, Montero MT, Hernández-BorrellJ. 2006. Surface planar bilayers of phospholipids used in protein membrane reconstitution: An atomic force microscopy study. Colloid Surface B 47:102–106.
- Domènech Ò, Redondo L, Montero MT, Hernández-Borrell J. 2007a. Specific adsorption of Cytochromec on cardiolipin-glycerophospholipid monolayers and bilayers. Langmuir 23:5651–5656.
- Domènech Ò, Morros A, Cabañas ME, Montero MT, Hernández-Borrell J. 2007b. Thermal response of domains in cardiolipin content bilayers. Ultramicroscopy 107:943–947.
- Domènech Ò, Redondo L, Picas L, Morros A, Montero MT, Hernández-Borrell J. 2007c. Atomic force microscopy characterization of supported planar bilayers that mimic the mitochondrial inner membrane. J Mol Recognit 20:546–553.
- Epand RF, Maloy L, Ramamoorthy A, Epand RM. 2010a. Amphipathic helical cationic antimicrobial peptides promote rapid formation of crystalline states in the presence of phosphatidylglycerol: Lipid clustering in anionic membranes. Biophys J 98:2564–2573.
- Epand RF, Maloy WL, Ramamoorthy A, Epand RM. 2010b. Probing the “charge cluster mechanism” in amphipathic helical cationic antimicrobial peptides. Biochemistry 49:4076–4084.
- Epand RF, Tokarska-Schlattner M, Schlattner U, Wallimann T, Epand RM. 2007. Cardiolipin clusters and membrane domain formation induced by mitochondrial proteins. J Mol Biol 365:968–980.
- Epand RF, Pollard JE, Wright JO, Savage PB, Epand RM. 2010c. Depolarization, bacterial membrane composition, and the antimicrobial action of ceragenins. Antimicrob Agents Chemother 54:3708–3713.
- Epand RM, Epand RF. 2009a. Domains in bacterial membranes and the action of antimicrobial agents. Mol BioSyst 5:580–587.
- Epand RM, Epand RF. 2009b. Lipid domains in bacterial membranes and the action of antimicrobial agents. Biochim Biophys Acta 1788:289–294.
- Epand RM, Epand RF. 2011. Bacterial membrane lipids in the action of antimicrobial agents. J Pept Sci 17:298–305.
- Epand RM, Rotem S, Mor A, Berno B, Epand RF. 2008. Bacterial membranes as predictors of antimicrobial potency. J Am Chem Soc 130:14346–14352.
- Epand RF, Wang G, Berno B, Epand RM. 2009. Lipid segregation explains selective toxicity of a series of fragments derived from the human cathelicidin LL-37. Antimicrob Agents Chemother 53:3705–3714.
- Esquembre R, Ferrer ML, Gutiérrez MC, Mallavia R, Mateo CR. 2007. Fluorescence study of the fluidity and cooperativity of the phase transitions of zwitterionic and anionic liposomes confined in sol-gel glasses. J Phys Chem B 111:3665–3673.
- Findlay B, Zhanel GG, Schweizer F. 2010. Cationic amphiphiles, a new generation of antimicrobials inspired by the natural antimicrobial peptide scaffold. Antimicrob Agents Chemother 54:4049–4058.
- Findlay JBC, Evans WH. 1990. Biological membranes a practical approach. Oxford: Oxford University Press.
- Frantz J-F, Elezgaray J, Berson P, Vacher P, Dufourc EJ. 2008. Pore formation induced by an antimicrobial peptide: Electrostatic effects. Biophys J 95:5748–5756.
- Frederix PLTM, Bosshart PD, Engel A. 2009. Atomic force microscopy of biological membranes. Biophys J 96:329–338.
- Frias M, Benesch MGK, Lewis RNAH, McElhaney RN. 2011. On the miscibility of cardiolipin with 1,2-diacyl phosphoglycerides: Binary mixtures of dimyristoylphosphatidylethanolamine and tetramyristoylcardiolipin. Biochim Biophys Acta 1808:774–783.
- Garidel P, Blume A. 2000. Miscibility of phosphatidylethanolamine-phosphatidylglycerol mixtures as a function of pH and acyl chain length. Eur Biophys J 28:629–638.
- Gong Y, Ma M, Luo Y, Bong D. 2008. Functional determinants of a synthetic vesicle fusion system. J Am Chem Soc 130:6196–6205.
- Guentzel M. 1996. Escherichia, Klebsiella, Enterobacter, Serratia, Citrobacter, and Proteus. Texas: University of Texas Medical Branch.
- Gunstone FD, Harwood JL, Padley FB. 1994. The Lipid Handbook. London: Chapman and Hall/CRC.
- Hadjicharalambous C, Sheynis T, Jelinek R, Shanahan MT, Ouellette AJ, Gizeli E. 2008. Mechanisms of alpha-defensin bactericidal action: Comparative membrane disruption by Cryptdin-4 and its disulfide-null analogue. Biochemistry 47:12626–12634.
- Haines TH. 2009. A new look at cardiolipin. Biochim Biophys Acta 1788:1997–2002.
- Hale JD, Hancock RE. 2007. Alternative mechanisms of action of cationic antimicrobial peptides on bacteria. Expert Rev Anti Infect Ther 5:951–959.
- Halling KK, Ramstedt B, Nyström JH, Slotte JP, Nyholm TKM. 2008. Cholesterol interactions with fluid-phase phospholipids: Effect on the lateral organization of the bilayer. Biophys J 95:3861–3871.
- Hawser S, Hoban D, Bouchillon S, Badal R, Carmeli Y, HawkeyP. 2011. Antimicrobial susceptibility of intra-abdominal Gram-negative bacilli from Europe: SMART Europe 2008. Eur J Clin Microbiol Infect Dis 30:173–179.
- Hoch FL. 1992. Cardiolipins and biomembrane function. Biochim Biophys Acta 1113:71–133.
- Huang C-H, Li S. 1999. Calorimetric and molecular mechanics studies of the thermotropic phase behavior of membrane phospholipids. Biochim Biophys Acta 1422:273–307.
- Huang Y, Huang J, Chen Y. 2010. Alpha-helical cationic antimicrobial peptides: Relationships of structure and function. Protein Cell 1:143–152.
- Hubner W, Mantsch HH, Kates M. 1991. Intramolecular hydrogen-bonding in cardiolipin. Biochim Biophys Acta 1066:166–174.
- Hurdle JG, O'Neill AJ, Chopra I, Lee RE. 2011. Targeting bacterial membrane function: An underexploited mechanism for treating persistent infections. Nat Rev Microbiol 9:62–75.
- Lakowicz J. 1999. Principles of fluorescence spectroscopy. New York: Kluwer.
- Leontiadou H, Mark AE, Marrink SJ. 2006. Antimicrobial peptides in action. J Am Chem Soc 128:12156–12161.
- Lewis RNAH, McElhaney RN. 2009. The physicochemical properties of cardiolipin bilayers and cardiolipin-containing lipid membranes. Biochim Biophys Acta 1788:2069–2079.
- Lewis RNAH, Zweytick D, Pabst G, Lohner K, McElhaney RN. 2007. Calorimetric, X-ray diffraction, and spectroscopic studies of the thermotropic phase behavior and organization of tetramyristoyl cardiolipin membranes.Biophys J. 92:3166–3177.
- Lohner K. 2001. The role of membrane lipid composition in cell targeting of antimicrobial peptides. Norfolk: Horizon Scientific Press.
- Lohner K. 2009. New strategies for novel antibiotics: Peptides targeting bacterial cell membranes. Gen Physiol Biophys 28:105–116.
- Lohner K, Blondelle SE. 2005. Molecular mechanisms of membrane perturbation by antimicrobial peptides and the use of biophysical studies in the design of novel peptide antibiotics. Comb Chem High Throughput Screen 8:241–256.
- Lohner K, Latal A, Degovics G, Garidel P. 2001. Packing characteristics of a model system mimicking cytoplasmic bacterial membranes. Chem Phys Lipids 111:177–192.
- Lohner K, Sevcsik E, Pabst G, Liu AL. 2008. Liposome-based biomembrane mimetic systems: Implications for lipid-peptide interactions. Adv Planar Lipid Bilayers Liposomes 6:103–137.
- Lopes S, Neves C, Eaton P, Gameiro P. 2010. Cardiolipin, a key component to mimic the E. coli bacterial membrane in model systems revealed by dynamic light scattering and steady-state fluorescence anisotropy. Anal Bioanal Chem 398:1357–1366.
- M'Baye G, Mély Y, Duportail G, Klymchenko AS. 2008. Liquid ordered and gel phases of lipid bilayers: Fluorescent probes reveal close fluidity but different hydration. Biophys J 95:1217–1225.
- Marczak A. 2009. Fluorescence anisotropy of membrane fluidity probes in human erythrocytes incubated with anthracyclines and glutaraldehyde. Bioelectrochemistry 74:236–239.
- Merino-Montero S, Montero MT, Hernández-Borrell J. 2006. Effects of lactose permease of Escherichia coli on the anisotropy and electrostatic surface potential of liposomes. Biophys Chem 119:101–105.
- Michel N, Fabiano A-S, Polidori A, Jack R, Pucci B. 2006. Determination of phase transition temperatures of lipids by light scattering. Chem Phys Lipids 139:11–19.
- Mileykovskaya E. 2007. Subcellular localization of Escherichia coli osmosensory transporter ProP: Focus on cardiolipin membrane domains. Mol Microbiol 64:1419–1422.
- Mileykovskaya E, Dowhan W. 2009. Cardiolipin membrane domains in prokaryotes and eukaryotes. Biochim Biophys Acta 1788:2084–2091.
- Naber KG, Hollauer K, Kirchbauer D, Witte W. 2000. In vitro activity of gatifloxacin compared with gemifloxacin, moxifloxacin, trovafloxacin, ciprofloxacin and ofloxacin against uropathogens cultured from patients with complicated urinary tract infections. Int J Antimicrob Agents 16:239–243.
- Nagamachi E, Kariyama R, Kanemasa Y. 1985. The effect of headgroup structure on phase transition of phospholipids membranes as determined by differential scanning calorimetry Physiol. Chem Phys Med NMR 17:255–260.
- Nicolas P. 2009. Multifunctional host defense peptides: Intracellular-targeting antimicrobial peptides. FEBS J 276:6483–6496.
- Oszlánczi Á, Bóta A, Berényi S, Klumpp E. 2010. Structural and morphological changes in bacteria-membrane mimetic DPPE/DPPG/water systems induced by sulfadiazine. Colloid Surface B 76:519–528.
- Pathan F, Venkata D, Panguluri S. 2010. Recent patents on antimicrobial peptides. Recent Pat DNA Gene Seq 4:10–16.
- Picas L, Montero MT, Morros A, Cabañas ME, Seantier B, Milhiet P-E, 2009. Calcium-induced formation of subdomains in phosphatidylethanolamine-phosphatidylglycerol bilayers: A combined DSC, 31P NMR, and AFM study. J Phys Chem B 113:4648–4655.
- Polyansky AA, Ramaswamy R, Volynsky PE, Sbalzarini IF, Marrink SJ, Efremov RG. 2010. Antimicrobial peptides induce growth of phosphatidylglycerol domains in a model bacterial membrane. J Phys Chem Lett 1:3108–3111.
- Pozo Navas B, Lohner K, Deutsch G, Sevcsik E, Riske KA, Dimova R, 2005. Composition dependence of vesicle morphology and mixing properties in a bacterial model membrane system. Biochim Biophys Acta 1716:40–48.
- Prossnigg F, Hickel A, Pabst G, Lohner K. 2010. Packing behaviour of two predominant anionic phospholipids of bacterial cytoplasmic membranes. Biophys Chem 150:129–135.
- Radzishevsky IS, Rotem S, Bourdetsky D, Navon-Venezia S, Carmeli Y, Mor A. 2007. Improved antimicrobial peptides based on acyl-lysine oligomers. Nat Biotechnol 25:657–659.
- Rainer S, Jain MK, Ramirez F, Ioannou PV, Marecek JF, Wagner R. 1979. Phase transition characteristics of diphosphatidylglycerol (cardiolipin) and stereoisomeric phosphatidyldiacylglycerol bilayers. mono-valent and divalent metal-ion effects. Biochim Biophys Acta 558:187–198.
- Raja M. 2010. The role of phosphatidic acid and cardiolipin in stability of the tetrameric assembly of potassium channel KcsA. J Membr Biol 234:235–240.
- Repáková J, Holopainen JM, Morrow MR, McDonald MC, Capková P, Vattulainen I. 2005. Influence of DPH on the structure and dynamics of a DPPC bilayer. Biophys J 88:3398–3410.
- Riske KA, Amaral LQ, Döbereiner HG, Lamy MT. 2004. Mesoscopic structure in the chain-melting regime of anionic phospholipid vesicles: DMPG. Biophys J 86:3722–3733.
- Robins-Browne RM, Cianciosi S, Bordun A-M, Wauters G. 1991. Pathogenicity of Yersinia kristensenii for mice. Infect Immun 59:162–167.
- Romantsov T, Guan Z, Wood JM. 2009. Cardiolipin and the osmotic stress responses of bacteria. Biochim Biophys Acta 1788:2092–2100.
- Rotem S, Radzishevsky IS, Bourdetsky D, Navon-Venezia S, Carmeli Y, Mor A. 2008. Analogous oligo-acyl-lysines with distinct antibacterial mechanisms. FASEB J 22:2652–2661.
- Sanderson JM. 2005. Peptide-lipid interactions: insights and perspectives. Org Biomol Chem 3:201–212.
- Sankaram MB, Powell GL, Marsh D. 1989. Effect of acyl chain composition on salt induced lamellar to inverted hexagonal phase transitions in cardiolipin. Biochim Biophys Acta 980:389–392.
- Schlame M, Ren M. 2009. The role of cardiolipin in the structural organization of mitochondrial membranes. Biochim Biophys Acta 1788:2080–2083.
- Seeger HM, Bortolotti CA, Alessandrini A, Facci P. 2009. Phase-transition-induced protein redistribution in lipid bilayers. J Phys Chem B 113:16654–16659.
- Sevcsik E, Pabst G, Jilek A, Lohner K. 2007. How lipids influence the mode of action of membrane-active peptides. Biochim Biophys Acta 1768:2586–2595.
- Sitterberg J, Gaspar MM, Ehrhardt C, Bakowsky U. 2010. Atomic force microscopy for the characterization of proteoliposomes. Methods Mol Biol 606:351–361.
- Spyratou E, Mourelatou EA, Makropoulou M, Demetzos C. 2009. Atomic force microscopy: A tool to study the structure, dynamics and stability of liposomal drug delivery systems. Expert Opin Drug Deliv 6:305–317.
- Stock I, Wiedemann B. 2003. Natural antimicrobial susceptibilities and biochemical profiles of Yersinia enterocolitica-like strains: Y. frederiksenii, Y. intermedia, Y. kristensenii and Y. rohdei. Immunol Med Mic 38:139–152.
- Sulakvelidze A. 2000. Yersiniae other than Y. enterocolitica, Y. pseudotuberculosis, and Y. pestis: The ignored species. Microbes Infect 2:497–513.
- Trevors JT. 2003. Fluorescent probes for bacterial cytoplasmic membrane research. J Biochem Biophys Methods 57:87–103.
- van Langen H, Schrama CA, van Ginkel G, Ranke G, Levine YK. 1989. Order and dynamics in the lamellar L alpha and in the hexagonal HII phase. Dioleoylphosphatidylethanolamine studied with angle-resolved fluorescence depolarization. Biophys J 55:937–947.
- Weber FJ, de Bont JAM. 1996. Adaptation mechanisms of microorganisms to the toxic effects of organic solvents on membranes. Biochim Biophys Acta 1286:225–245.
- Wydro P, Witkowska K. 2009. The interactions between phosphatidylglycerol and phosphatidylethanolamines in model bacterial membranes: The effect of the acyl chain length and saturation. Colloid Surface B 72:32–39.
- Yeagle P. 1987. The membranes of cells. New York: Academic Press, Inc.
- Yokota K, Kanamoto R, Kito M. 1980. Composition of cardiolipin molecular species in Escherichia coli. J Bacteriol 141:1047–1051.
- Zhao W, Róg T, Gurtovenko AA, Vattulainen I, Karttunen M. 2008. Role of phosphatidylglycerols in the stability of bacterial membranes. Biochimie 90:930–938.