Abstract
2-Hydroxyoleic acid (2OHOA) is a synthetic fatty acid with antihypertensive properties that is able to alter structural membranes properties. The main purpose of this study was to analyze the effect of 2OHOA on the membrane architecture in cholesterol (Cho)-rich domains. For this purpose, model membranes mimicking the composition of lipid rafts and PC- or PE-Cho-rich domains were examined in the absence and presence of 2OHOA by synchrotron X-ray diffraction, atomic force microscopy (AFM) and microcalorimetry (DSC) techniques. Our results demonstrate that 2OHOA phase separates from lipid raft domains and affects the lateral organization of lipids in the membrane. In model raft membranes, 2OHOA interacted with the sphingomyelin (SM) gel phase increasing the thickness of the water layer, which should lead to increased bilayer fluidity. The hydrogen binding competition between 2OHOA and Cho could favour the enrichment of 2OHOA in SM domains separated from the SM-Cho domains, resulting in an enhanced phase separation into SM-2OHOA-rich liquid-disordered (non-raft) and SM-Cho-rich liquid-ordered (raft) domains. The segregation into 2OHOA-rich/Cho-poor and 2OHOA-poor/Cho-rich domains was also observed in PC bilayers.
Introduction
The heterogeneous lateral organization of lipids and proteins is a crucial feature in cell membrane functions affecting a variety of processes, including signal transduction (Simons and Toomre Citation2000). Its relevance has been the subject of intense scrutiny (Lingwood and Simons Citation2010). Cholesterol (Cho)-rich domains constitute one example of lateral heterogeneity in the cell surface. Lipid rafts represent a type of Cho-rich domains characterized by a high concentration of Cho and sphingomyelin (SM) organized as a liquid-ordered phase (Edidin Citation2003). However, there may also be other Cho-rich domains in cell membranes composed by specific lipids and proteins that do not fit the concept of lipid raft (Epand et al. Citation2003).
In the context of lipid lateral heterogeneities, an important question is related to the organizing principles governing the structural and functional properties of lipid assemblies at the surface of living cells. To understand lipid-dependent membrane organization, lipid research has been focused on the characterization of lipid-lipid and lipid-protein interactions that promote lipid segregation and phase separation. In model membranes, phase separation occurs in binary and ternary mixtures of lipids that have different phase transition temperatures. Studies conducted on ternary lipid mixtures, combining Cho with saturated and unsaturated lipids, have shown coexistence of a Cho-enriched liquid-ordered (lo) phase and a Cho-depleted liquid-disordered (ld) phase and both phases differ from the solid-ordered gel phase. Phase diagrams have been mapped to reveal under which conditions coexisting immiscible liquid phases can be observed (Simons and Vaz Citation2004, Veatch and Keller Citation2005). It has been argued that Cho shows a preferential interaction for sphingolipids over PC lipids with similar acyl chains (Ramstedt and Slotte Citation2006). Moreover, the lipid composition of membrane rafts isolated by conventional methods showed enrichment in sphingolipids and Cho (Simons and Vaz Citation2004). Cho seems to be responsible for driving lipid phase separation thereby creating coexisting lo and ld domains in bilayers (London and Brown Citation2000). For these reasons, the study of liquid immiscible phases and raft formation has been emphasized on sphingolipids/Cho-enriched domains. Most biophysical studies on binary and ternary lipid mixtures have been conducted using bulk techniques, such as synchrotron X-ray diffraction (Chachaty et al. Citation2005, Mills et al. Citation2008, Quinn and Wolf Citation2009, Quinn and Wolf Citation2010), fluorescence spectroscopy (de Almeida et al. Citation2003, Nicolini et al. Citation2006); fluorescence microscopy (Dietrich et al. Citation2001, Stockl et al. Citation2008) and atomic force microscopy (Rinia and de Kruijff Citation2001, Lawrence et al. Citation2003, van Duyl et al. Citation2003). The static structure and phase behaviour of several lipid mixtures have been well characterized. There is abundant evidence that the lateral distribution of lipids in the bilayer can be driven by specific lipid-lipid interactions. However, few studies have analyzed the effect of membrane intercalating compounds on lipid phase domains.
2-Hydroxyoleic acid (2OHOA) is a synthetic fatty acid with antihypertensive properties (Alemany et al. Citation2004). We demonstrated that short-term administration of 2OHOA decreased blood pressure in animal models by modulating G-protein-mediated signal transduction (Alemany et al. Citation2006). The molecular mechanism of 2OHOA that underlies these effects is still unknown. However, if the membrane structure is involved in signal transduction processes, then structural alterations of cell membranes affect membrane behaviour and, thus, may influence physiological processes and ameliorate pathological situations. This hypothesis is consistent with the fact that 2OHOA affected the structural properties of biological and model membranes (Barceló et al. Citation2004, Prades et al. Citation2008). Therefore, it is important to analyze the effect of 2OHOA on the membrane lateral heterogeneity and lipid organization. The present study was undertaken to determine whether partitioning of Cho into lipid domains may be modulated by the effect of a 2-hydroxlated fatty acid. The specific question posed was: Can 2OHOA affect membrane architecture in Cho rich domains? For this purpose, simple model systems containing Cho and different lipid compositions with gel/fluid and liquid/liquid phases were studied by X-ray diffraction, differential scanning microcalorimetry (DSC) and atomic force microscopy (AFM) analysis.
Materials and methods
Materials
1,2-dioleoyl-sn-glycero-3-phosphatidylcholine (DOPC), 1-palmitoyl,2-oleoyl-sn-glycero-3-phosphatidylcholine (POPC), 1-palmitoyl,2-oleoyl-sn-glycero-3-phosphatidylethanolamine (POPE), egg sphingomyelin (SM) and 2-hydroxyoleic acid were purchased from Avanti Polar Lipids, Inc. (Alabaster, AL, USA) and stored under argon at −80°C. Cholesterol (Cho) and N-(2-Hydroxy ethyl) piperazine-N’-(2-ethanesulfonic acid) sodium salt (Hepes) were obtained from Sigma Chem. Co. (Poole, Dorset, UK).
Sample preparation
For X-ray diffraction experiments, multilamellar lipid vesicles (MLV) 15% (w/w) were prepared in 10 mM Hepes, 100 mM NaCl, 1 mM EDTA, pH 7.4 (Hepes buffer) according to established procedures (Funari et al. Citation2003). Lipid powder was hydrated in the presence or absence of 2OHOA at the desired molar ratio, and the sample was thoroughly homogenized with a pestle-type minihomogenizer (Sigma) and vortexed until a homogeneous mixture was obtained. The suspensions were then submitted to seven temperature cycles (heating to 70°C and cooling to −20°C) to ensure complete homogenization. Samples were allowed to equilibrate at 4°C during 24 h before X-ray diffraction examination. The reversibility of phase behaviour was verified as a control of a possible dehydration of the lipid mixtures. For AFM and DSC analysis, lipids dissolved in chloroform/methanol (2:1, v/v) were mixed at the molar ratio specified. Lipid mixtures were evaporated under argon and vacuum-dried. The lipid film was resuspended in Hepes buffer by vortex. The lipid suspension (2 mM) was submitted to seven freeze/thaw cycles (70°C and −20°C) to guarantee complete hydration of the lipid vesicles. For AFM experiments, MLV were sonicated in a bath sonicator (Branson) for 40 min, to obtain small unilamellar vesicles (SUV) for the preparation of the supported bilayers.
Synchrotron X-ray diffraction analysis
Measurements were conducted at the Soft Condensed Matter beamline A2 of HASYLAB at the Deutsches Elektronen Synchrotron (DESY). The X-ray wavelength was 0.15 nm. The sample to detector distance was 1.5 m. Small and Wide-Angle (SAXS and WAXS) Synchrotron radiation X-ray scattering data were collected simultaneously, using standard procedures. Silver behenate and polyethylene theraphtalate were employed to calibrate the SAXS and WAXS regions, respectively. Positions of the observed peaks were converted into distances, d (nm), after calibration using the standards indicated. Data from each sample were acquired continuously for 15 s at each temperature, followed by a waiting time of 45 s with a local shutter closed.
Lipid mixtures (20 μl) were dispersed in the measurement cell and mounted on a programmable temperature stage. The data collection analyses were the same as those described previously (Funari et al. Citation2003). For measurement of lipid mixtures in quasi-equilibrium conditions, samples were allowed to equilibrate for 15 min at each temperature before data acquisition. To work in dynamic conditions, the system was heated at a scan rate of 1°C/min to the higher temperature, kept at this temperature for 5 min and then cooled to the lower temperature to check the reversibility of the transitions. At the end of the experiment, the samples were maintained at the lowest temperature for 5 min to confirm the phase structure. SM samples, binary and ternary mixtures of POPC, DOPC, SM and Cho were heated from 20–50°C and then cooled-down to 20°C, working in quasi-equilibrium and dynamic conditions. DOPC samples were run in the range temperature 20–40°C. POPE and POPE:Cho mixtures were heated from 10–85°C and cooled down to 10°C working in dynamic conditions. Data analysis was performed using ORIGIN software (Origin Lab Corp., Northampton, MA, USA). Peak decomposition analysis of the SAXS intensity peaks was undertaken using PEAKFIT (v4.12) software. Peaks could be fitted by Lorentz symmetrical functions with fitting coefficients R2 ≥ 0.95.
Differential scanning calorimetry (DSC)
Experiments were performed on a high-resolution differential scanning microcalorimeter (MC-2, Microcal). MLV (2 mM lipids) were heated at a scan rate of 1°C/min. The transition temperature and enthalpy was calculated by fitting the transitions to a single Van't Hoff component. The gel-to-liquid lamellar phase transition temperature (Tm) corresponded to the maximum excess heat capacity.
Atomic force microscopy (AFM)
The analysis was carried out on a Nanoscope IV (Digital instruments, Santa Barbara, CA, USA). Supported membrane bilayers were prepared with SUV suspensions (2 mM in PL). 10 μl of SUV suspension were applied onto freshly cleaved mica (diameter 1 cm), allowed to absorb and fuse on the mica during 60 min at room temperature. The sample was rinsed with Hepes buffer and used for scanning with AFM. They were scanned in constant-force mode using silicon nitride tips on integral cantilevers with a spring constant of 0.06 N/m, as estimated by the manufacturers (Digital Instruments). Scans were recorded at a minimal force where the image was stable (usually smaller than 500 pN). All samples were imaged at room temperature. The Nanoscope software was used to obtain the cross-section of the domains. Two-three independently prepared samples were imaged for each lipid composition, and several areas were scanned for each sample.
Results
X-ray diffraction analysis
We analyzed the effect of 2OHOA on the thermotropic phase behaviour and structure of lipid mixtures-Cho rich, working in quasi-equilibrium (equilibrating the samples 15 min at each temperature before data acquisition) and dynamic conditions (scan rate at 1°C/min). The study was focused on the ternary mixture DOPC:SM equimolar and Cho 30 mol%, representative of a model lipid raft, and the binary mixtures POPC:Cho 30 mol% and POPE:Cho 30 mol%.
Lipid mixture DOPC:SM equimolar and Cho 30 mol%
The X-ray diffraction pattern of the ternary mixture running in quasi-equilibrium conditions is depicted in , panel A. In the range temperature 20–37°C, three broad diffraction peaks overlapping (d-spacing ∼7.2, 6.8 and 5.8 nm) were observed in the SAXS. In addition, a diffraction peak with d-spacing 3.5 nm was seen at all temperatures and was assigned to immiscible Cho domain. The presence of a WAXS peak with d-spacing 0.43 nm indicated the occurrence of a gel phase in the mixture. At temperatures above 40°C, the SAXS patterns showed sharp diffraction peaks (d-spacing ∼6.8 nm) accompanied by the corresponding second order diffraction peak and a broad peak with d-spacing ∼5.8 nm. The absence of a WAXS peak and the presence of second-order reflections were indicative of the coexistence of liquid lamellar structures. The SAXS and WAXS patterns recorded in a subsequent cooling scan were reversible with no significant temperature hysteresis. The thermotropic behaviour of the ternary lipid mixture was also analyzed in dynamic conditions to follow the melting profile of the lipid domains that were defined while working in quasi-equilibrium conditions (, panel D). To assign the SAXS diffraction peaks in the ternary mixture to lipid populations, further studies were carried out with control lipid mixtures subjected to a similar heating/cooling scan conditions. The thermotropic phase behaviour of pure SM samples (, panel C) had a Lβ-to-Lα phase transition at 39°C, in agreement with DSC and XRD data previously reported (Chachaty et al. Citation2005). The Lβ phase was characterized by a lamellar repeat distance of 7.2 nm and a wide-angle d-spacing of 0.43 nm. The Lα phase identified by two-orders of diffraction peaks in the SAXS had a d-spacing of 6.8 nm. The binary mixture SM:Cho 25 mol% (, panel B) showed two coexisting phases defined by the first two orders of diffraction peaks in the SAXS region (d-values ∼7.6 and 6.8 nm) below the melting temperature. Indeed, a diffraction peak with d-spacing = 3.5 nm, assigned to immiscible Cho domains, was also observed. By increasing the temperature, a phase transition related to the SM occurred in the binary mixture. Above the SM melting temperature, a unique Lα phase with d = 6.8 nm appeared as in the system of pure SM. A similar pattern was observed working in dynamic conditions (, panel E).
Figure 1. Linear plots of the X-ray scattering pattern. Small-angle (SAXS) and wide-angle X-ray scattering (WAXS) patterns recorded from the lipid mixtures indicated in the absence (A, B, C, D and E) and presence (F, G, H, I and J) of 2OHOA 40:1 (lipid:fatty acid molar ratio). The sequence of the patterns (A, B, F and G) was acquired in quasi-equilibrium conditions after equilibrating the sample during 15 min at each temperature. The other patterns (C, D, E, H, I and J) were recorded in dynamic conditions at a scan rate of 1°C/min. Successive diffraction patterns were collected for 15 s every minute. The Lβ phase was characterized by the first order reflection peak on the SAXS and the presence of a peak on the WAXS region. The Lα phase was identified by the first and second order reflection peaks on the SAXS and the absence of peak in the WAXS region. The phase transition Lβ -to-Lα was defined by the vanishing peak in the WAXS region.
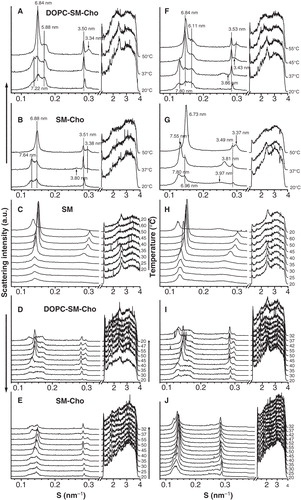
The composition of the coexisting lamellar phases in the binary and the ternary mixtures was assigned on the basis of the relationship between the d-spacing parameter and the temperature (). For this purpose, the first-order Bragg reflections were examined by peak fitting analysis using Lorentz symmetrical functions (). In SM:Cho 30 mol% samples, the scattering was split into two peaks representing ∼35 (peak 1) and 65% (peak 2) of the total. From the analysis of the d-spacing vs. temperature obtained in quasi-equilibrium and dynamic conditions (), peak 1 in the binary mixture (d-spacing ∼7.6 nm) could match with a Lβ phase SM rich from considerations of the thermotropic behaviour of pure SM samples. Therefore, peak 2 in SM:Cho 30 mol% samples (d-spacing ∼6.9 nm; see ) might correspond to a liquid phase SM-Cho-rich domain (Pandit et al. Citation2004). On the other hand, in DOPC:SM equimolar and Cho30 mol% mixtures, three peaks contributed to ∼21 (peak 1), 55 (peak 2) and 24% (peak 3) to the total intensity scattering below the SM phase transition. They were consistent with the presence of a gel phase (peak 1 with d-value ∼7.2 nm), in coexistence with two liquid phases, most likely a liquid-ordered (lo, peak 2 with d-values ∼6.8 nm) and a liquid-disordered (ld, peak 3 with d-value ∼5.8 nm) phase. The d-spacing of peak 1 was close to the value recorded from SM in the gel phase and was allocated to a SM gel rich domain. The two liquid phases could be associated to SM-Cho enriched and DOPC-rich domains, respectively. Peak 2 was allocated to the SM-Cho enriched phase looking at the lamellar d-spacing (Quinn and Wolf Citation2009). The SM-Cho rich domain would be ∼1 nm thicker than the DOPC-rich domain, in agreement with data reported with model raft mixtures analyzed by AFM experiments and molecular dynamic simulations (Rinia et al. Citation2001, Pandit et al. Citation2004). The assignment of peak 3 to a DOPC-rich domain was considered from the d-spacing value of pure DOPC membranes (). The decrease in the d-spacing of the DOPC domain might be due to the occurrence of Cho that could organize as a DOPC-Cho domain (Parker et al. Citation2004). It has been estimated by H-NMR that a DOPC-enriched phase in an equimolar DOPC/SM/Cho mixture could contain around 10–15% Cho (van Duyl et al. Citation2003). The data of our analysis would be in line with the phase diagram reported for DOPC:SM:Cho samples by fluorescence microscopy (Veatch and Keller Citation2005). Finally, the ternary mixture underwent phase separation into two liquid phase domains (d ∼6.8 nm and 5.8 nm, see ), due to the SM melting by increasing the temperature. They might be associated to SM- and DOPC-rich liquid phases in the presence of Cho, although the analysis was limited to conclude about the Cho distribution in both phases.
Figure 2. Analysis of the X-ray scattering patterns. (A) Peak fitting analysis from the first-order lamellar reflections recorded at 37°C: experimental data (- - -) and individual components (—). (B) Lamellar d-spacing as a function of the temperature from the lipid mixtures indicated in the absence and presence of 2OHOA (40:1, lipid:fatty acid molar ratio).
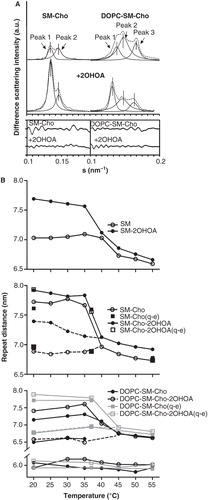
DOPC:SM equimolar and Cho 30 mol% mixtures showed the presence of phase separation domains, in agreement with recent XRD data reported on ternary mixtures with a membrane raft lipid composition (Quinn and Wolf Citation2010). However, they differ from previous data that found no evidence for any phase separation working with mixtures of SM and Cho in the range 33–50 mol% and SM:PC:Cho 33:33:33 mol% (Chachaty et al. Citation2005). There are controversial results about the phase separation of lipid-raft model mixtures (Gandhavadi et al. Citation2002, Veatch et al. Citation2004, Chachaty et al. Citation2005, Quinn and Wolf Citation2010). It has been argued that sample preparation procedures could influence the apparent phase behaviour of such model membranes (Silvius Citation2003, Buboltz et al. Citation2007). It is worth noting that when the same analyzed samples were measured 14 months later, they showed a very clear sequence of X-ray diffraction patterns corresponding to a complete homogeneous mixture with a well organized Lα phase (data not shown). The differences in the experimental data depending on the sample history could indicate that the formation of ordered domains in model membranes freshly prepared is associated to the occurrence of metastable phase separations. In Cho-rich lipid bilayers prepared by the conventional film-deposition procedure, the lipid segregation in Cho-enriched and Cho-depleted metastable domains could determine, in part, the differences observed in the phase behaviour of raft model membranes and related to the sample history (Chachaty et al. Citation2005, Quinn and Wolf Citation2010). On the other hand, an apparent low Cho solubility was observed in our experiments, compared to the Cho limit solubility in PC and raft model membranes reported by X-ray diffraction studies (Huang et al. Citation1999, Gandhavadi et al. Citation2002, Chachaty et al. Citation2005, Quinn and Wolf Citation2010). These differences could also be related to the method used for sample preparation and analysis (Knoll et al. Citation1985, Epand et al. Citation2000).
Table I. Structural properties of lipid mixtures.
Lipid mixture DOPC:SM equimolar, Cho 30 mol% and 2OHOA (40:1, lipid:fatty acid molar ratio)
The XRD patterns of the ternary mixture were altered by the presence of 2OHOA, varying the d-spacing of lipid populations, without changing the thermotropic phase behaviour significantly (, panels A, F). Working in quasi-equilibrium conditions, 2OHOA the relative area of peak 1 attributed to a SM gel phase and increased the d-value (d = 7.8 at 37°C) (see ). In addition, 2OHOA decreased the Cho peak intensity (d = 3.5 nm) and augmented the lamellar d-spacing of peak 3 (d = 6.1 nm) assigned to DOPC rich domains (see , panels A and F). The 2OHOA effect on DOPC domains was probably due to the incorporation of the 2-hydroxylated fatty acid within the bilayer, as it was also observed in the control DOPC:2OHOA samples (). Running the binary mixture SM:Cho 30 mol% in the presence of 2OHOA in quasi-equilibrium conditions (, panel G), 2OHOA affected the SM gel phase in a similar way as has been described above in the ternary mixture (, panels B and G). In turn, control SM samples in the absence and presence of 2OHOA corroborated the specific 2OHOA effect on the SM gel phase (, panels C and H). 2OHOA broadened the peak assigned to the SM gel phase, increased the d-spacing (d ∼ 7.6 nm at 30°C) and keep the gel phase ca. 5°C under the Lβ-to-Lα phase transition temperature of the pure SM dispersions (, upper panel). The presence of homogeneous lipid populations in SM:2OHOA mixtures was checked by DSC (). SM dispersions showed a Tm = 39°C and a calorimetric enthalpy = 6.4 Kcal/mol. 2OHOA induced a modest concentration-dependent decrease in both Tm and enthalpy associated with the Lβ-to-Lα phase transition (∼2°C and 0.3 Kcal/mol at SM:2OHOA, 20:1 molar ratio). From the X-ray diffraction data, the 2OHOA effect on the SM gel phase could be interpreted in the sense that the 2-hydroxylated fatty acid would increase the repulsion between SM and DOPC rich bilayers causing a thicker water layer, most likely related to the capacity of the hydroxyl group to form hydrogen bonds with the SM polar head groups. This should inevitably increase the bilayers fluidity.
Figure 3. DSC heating thermograms of SM:2OHOA mixtures. SM membranes in the absence and presence of 2OHOA at the molar ratio indicated in each plot. Endothermic heat flow was normalized to the phospholipid concentration. DSC scans were performed at a scan rate of 1°C/min.
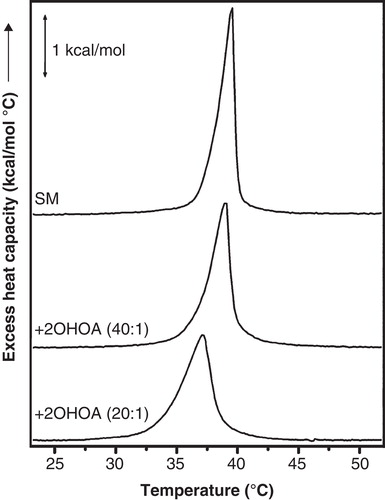
The binary and ternary mixtures in the presence of 2OHOA were also run in dynamic conditions (, panels I and J). The plots obtained illustrated some significant differences. A much poorer separation of the coexisting phases was observed and the Cho peak intensity increased (see , panels F and I; G and J). The dissimilarities between the data obtained in quasi-equilibrium and dynamic conditions may be due to several factors, being the most important the fact that the system did not have the necessary time to reach equilibrium and therefore it was constantly in a transition state. The heating scan will facilitate the molecules to diffuse leading to the formation of clusters or aggregates that became better separated upon cooling.
AFM analysis
Lipid mixtures DOPC:SM equimolar and Cho 25 mol% in the absence and presence of 2OHOA (lipid:fatty acid, 40:1 molar ratio) were imaged with AFM at room temperature. A representative image is shown in (panels A, B). The ternary mixture depicted a large number of islands ∼5 nm above the underlying mica and occupied an average amount of ∼40–50% of the total area. The cross-section of the line drawn in panel (A) is illustrated in panel (C). The size of the bright domains was in the order of ∼300–900 nm in horizontal distance and protrude ∼0.6–0.8 nm from the surrounding bilayer. AFM images showed a mixture of two coexisting phases in agreement with data previously reported with model lipid raft membranes (Rinia et al. Citation2001). Bright domains could be assigned to a condensed SM-rich phase that would be surrounded by lipids in a fluid phase, most probably a DOPC-rich phase. Addition of 2OHOA (lipid:2OHOA, 40:1, molar ratio) resulted in a decrease of the size and the number of islands (∼24–30% of the total area) (panels C and D). The islands emerged ∼5 nm from the matrix and the domains were in the range ∼200–400 nm (horizontal distance). The height level emerging from the lipid bilayer did not change significantly in the presence of 2OHOA. AFM data showed that 2OHOA did not apparently affect the phase separation present in the lipid raft model membranes and point to a 2OHOA fluidifying effect on the lipid bilayer.
Figure 4. AFM images of DOPC:SM equimolar and Cho 25 mol% mixtures. Lipid mixture in the absence (A) and presence (C) of 2OHOA (lipid:fatty acid, 40:1 molar ratio). The horizontal black line marks the location of the section analysis shown in (B) and (D). The arrows denote the two different height levels of the domains. Vertical distances between the arrows were 4.96 and 0.60 nm (panel B) and 5.0 and 0.61 nm (panel D).
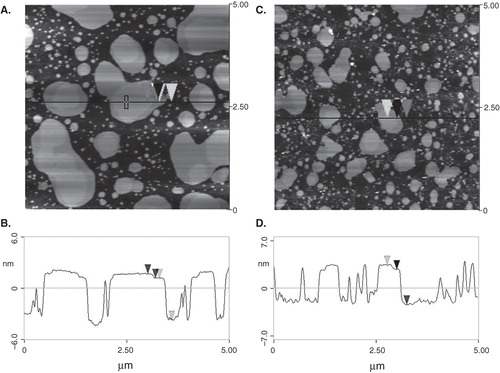
Lipid mixture POPC:Cho (30 mol%) and 2OHOA (20:1, lipid:fatty acid molar ratio)
The X-ray diffraction patterns in the absence and presence of 2OHOA are illustrated in . POPC:Cho (30 mol%) samples showed a sharp peak assigned to a Lα phase (d = 6.6 nm at 30°C) overlapped with a broader peak centered at d ∼6.1 nm and a peak at 3.5 nm due to the presence of an immiscible CHO domain. The Lα phase was identified on the basis of the absence of WAXS diffraction peaks and considerations of the diffraction pattern of pure POPC membranes (Lα phase with d-spacing = 6.5 nm) identified by two orders of diffraction peaks (). Lipid mixtures in the presence of 2OHOA had two overlapped diffraction peaks matching to lamellar liquid phases with d-values of 7.1 and 6.7 nm at 30°C and a broad shoulder (d ∼6.4 nm). The thermal compressibility factor of the phases was increased in the presence of 2OHOA. The sharp peak corresponding to Cho domains became broader in the presence of 2OHOA and it was overlapped by the higher order diffraction peaks of the lamellar phases. Control experiments with POPC:2OHOA (20:1, molar ratio) illustrated a similar diffraction pattern as POPC membranes, with no change in the thermotropic behaviour and the structural properties, indicating a 2OHOA miscibility in POPC membranes. Considering the experimental data of 2OHOA lipid mixtures and the corresponding controls, the 2OHOA effect was to enhance the segregation of the components in the mixture (probably POPC:2OHOA and POPC:Cho domains) and to disorder the Cho-rich domain partially.
Figure 5. Analysis of the X-ray scattering pattern of POPC:Cho (30 mol%) membranes. Small-angle (SAXS) and wide-angle (WAXS) scattering patterns recorded in the absence (A) and presence (B) of 2OHOA 40:1 (lipid:fatty acid molar ratio). The sequence of patterns was acquired in dynamic conditions with a scan rate of 1°C/min. Successive diffraction patterns were collected for 15 s every minute. The Lα phase was identified by the first and second order reflection peaks on the SAXS and the absence of peaks on the WAXS region. Right panels: Dependence of the repeat distance with temperature for the XRD pattern in the absence (C) and presence (D) of 2OHOA. Phases represented are Lα1 (▪) and Lα2 (•) and d-spacing of immiscible Cho peak (▴).
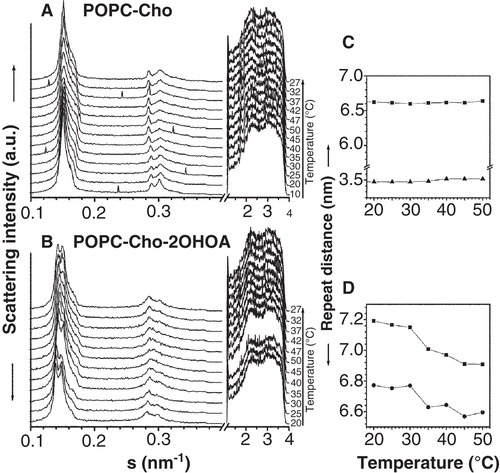
Lipid mixture POPE:Cho (30 mol%) and 2OHOA (20:1, lipid:fatty acid molar ratio)
POPE:Cho samples in the absence and presence of 2OHOA had similar diffraction patterns (). POPE:Cho mixtures showed a lamellar Lβ phase (d-spacing 6.3 nm), indexed by one order of reflection and a sharp wide-angle reflection in the WAXS (d-spacing 0.43 nm), that coexisted with a liquid crystalline Lα phase (d-spacing 5.8 nm) (panels A, D). The thermotropic behaviour illustrated a Lβ-to-Lα phase transition at ∼25°C characterized by the disappearance of the peak in the WAXS and a Lα-to-HII phase transition at ∼56°C. The HII phase, defined by three orders of reflexions in the SAXS, had a d-value of 6.0 nm at 80°C. Cho (30 mol%) shifted the Lα-to-HII phase transition of POPE membranes toward lower temperature in agreement with data published (Takahashi et al. Citation1996, Pare and Lafleur Citation1998). The presence of 2OHOA in POPE Cho-rich mixtures did not abolish the Lα phase below 25°C or alter the onset of the Lα-to-HII phase transition (panels B, E). In turn, POPE:2OHOA (20:1, molar ratio) mixtures (panels C, F) showed a phase sequence Lβ-to-Lα and Lα-to-HII similar to POPE membranes. The effect of 2OHOA was to induce a HII phase propensity (Barceló et al. Citation2004), reducing the onset of the Lα-to-HII phase transition temperature from 71–65°C ().
Figure 6. Analysis of the X-ray scattering pattern of POPE:Cho (30 mol%) membranes. Small-angle (SAXS) and wide-angle (WAXS) scattering patterns recorded from POPE:Cho (30 mol%) (A, B) and POPE (C) mixtures in the absence (A) and presence of 2OHOA (40:1, lipid:fatty acid molar ratio) (B, C). The sequence of patterns was acquired in dynamic conditions with a scan rate of 1°C/min. The Lβ phase was characterized by the first order reflection peak on the SAXS and the presence of a peak on the WAXS region. The Lα phase was identified by the first and second order reflection peaks on the SAXS and the absence of peaks on the WAXS region. The phase transition Lβ-to-Lα was defined by the vanishing peak on the WAXS region. The HII phase was recognized by three higher-order reflection peaks indicated. Right panels: Dependence of the repeat distance with temperature for the lipid mixtures indicated (B, E, F). Phases represented are Lβ (▪), Lα (•) and HII (▴,▾).
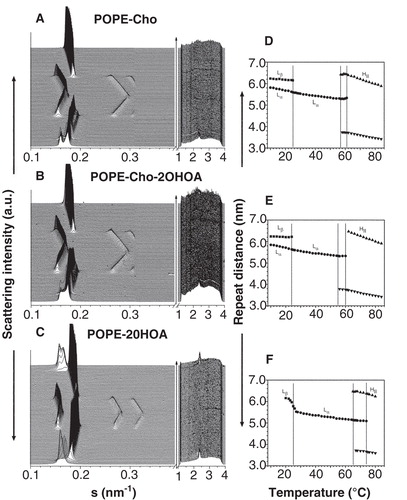
Discussion
The results presented demonstrate that 2OHOA phase separates from Cho-rich domains and affects the lateral organization of membrane lipids. In DOPC:SM:Cho model raft membranes, 2OHOA interacts with the SM gel phase increasing the thickness of the water layer, which should cause increased bilayer fluidity. The α-hydroxyl group of 2OHOA could act as a hydrogen-bond donor with the carbonyl oxygen of the phospholipid acyl chains (Barceló et al. Citation2004) and possibly with the SM amide group, like Cho (Ramstedt and Slotte Citation2006). Therefore, a hydrogen binding competition between 2OHOA and Cho at the polar/non-polar interface of the bilayer, in addition to the steric incompatibility of the rigid steroid moiety and the highly disordered fatty acyl chain with a cis double bond, could favour a 2OHOA enrichment in SM domains separated from the SM-Cho-rich domains, resulting in an enhanced phase separation into SM-2OHOA-rich liquid-disordered (non-raft) and SM-Cho-rich liquid-ordered domains (lipid rafts). 2OHOA phase separation might also occur in PC-Cho-rich membranes. On the other hand, the interaction of SM with 2OHOA seems to solubilise Cho in model membranes mimicking the composition of lipid rafts. The increase in membrane fluidity, due to the presence of 2OHOA, could favour the solubility of Cho in the bilayer and as a consequence a lipid reorganization in the membrane would take place (Kahya et al. Citation2003). The lipid restructuring effect of 2OHOA in DOPC:SM:Cho membranes (raft-like lipid domains) may occur through the recruitment of the Cho into DOPC and SM-Cho domains and the SM into SM-2OHOA-rich and SM-Cho domains. Interestingly, the 2OHOA effect of increasing the Cho solubility in DOPC:SM:Cho and SM:Cho samples was not strong enough to prevent the phase separation of the previously observed coexisting domains in raft model membranes. According to the X-ray diffraction and the AFM studies, we are limited to conclude about how the different domains, and particularly the SM-Cho-rich domain, are affected by the 2OHOA-membrane interaction. We hypothesize that 2OHOA might act as a nucleation centre for the formation of SM-rich and Cho-poor fluid domains altering the membrane lateral organization, which should consequently have implications in the lateral organization of lipids in the cell membrane.
Biological significance
Some membrane-intercalating compounds, like hexadecanol, ceramides and diglycerides, have been observed to displace Cho from domains of lipid membranes (Megha and London Citation2004, Alanko et al. Citation2005, Ratajczak et al. Citation2007). On the contrary, 2OHOA may in part function by enhancing the formation of Cho-poor fluid domains and the segregation of Cho in membrane domains, thereby altering the size and composition of the lipid micro-environments on the cell surface. Although, the mechanism of the 2OHOA effect in cells is likely multifaceted, one important function of 2OHOA could be related to an effect on the membrane lipid structure (Prades et al. Citation2008) and lateral organization of lipids in the cell membrane. These modified properties could have implications in the cellular signalling events related with physiological processes, such as hypertension. In other words, if 2OHOA affects membrane domain organization, then its physiological effect on diseases (e.g., hypertension) could be triggered by influencing cell membrane processes, for example, the function of associated membrane proteins whose localization and activity in cell signalling depend on the lipid domain structure of the cell membrane (Vogler et al. Citation2004, Alemany et al. Citation2006).
Conclusions
The findings of this study demonstrate that in model membranes mimicking the composition of lipid rafts or PC-Cho rich domains, 2OHOA phase separates from Cho-rich domains. In lipid raft membranes, 2OHOA interact with the SM gel phase. The hydrogen binding competition between 2OHOA and Cho could favour the enrichment of 2OHOA in SM domains separated from the SM-Cho domains, resulting in an enhanced phase separation into SM-2OHOA-rich liquid-disordered (non-raft) and SM-Cho-rich liquid-ordered (raft) domains. Although, the mechanism of the 2OHOA in cells is likely multifaceted, one important function of 2OHOA could be related to an effect on the lateral organization of lipids in the membrane and on the membrane lipid structure (Prades et al. Citation2008).
Acknowledgements
This work was funded by II-05-051EC from Deutsches Elektronen-Synchrotron DESY, HASYLAB (Hamburg, Germany); by the IHP-Contract HPRI-CT-2001-00140 of the European Commission. The authors want to thank the “Laboratorio Científico Técnico” (UIB) for the assistance in providing the infrastructure to conduct the study. We are grateful to Dr Joan Cifre for his technical assistance and Coral Prades-Tortosa for her contribution to the manuscript.
Declaration of interest: The authors report no conflicts of interest. The authors alone are responsible for the content and writing of the paper.
References
- Alanko SM, Halling KK, Maunula S, Slotte JP, Ramstedt B. 2005. Displacement of sterols from sterol/sphingomyelin domains in fluid bilayer membranes by competing molecules. Biochim Biophys Acta 1715:111–121.
- Alemany R, Teres S, Baamonde C, Benet M, Vogler O, Escriba PV. 2004. 2-hydroxyoleic acid: A new hypotensive molecule. Hypertension 43:249–254.
- Alemany R, Vogler O, Teres S, Egea C, Baamonde C, Barceló F, 2006. Antihypertensive action of 2-hydroxyoleic acid in SHRs via modulation of the protein kinase A pathway and Rho kinase. J Lipid Res 47:1762–1770.
- Barceló F, Prades J, Funari SS, Frau J, Alemany R, Escriba PV. 2004. The hypotensive drug 2-hydroxyoleic acid modifies the structural properties of model membranes. Mol Membr Biol 21:261–268.
- Buboltz JT, Bwalya C, Williams K, Schutzer M. 2007. High-resolution mapping of phase behavior in a ternary lipid mixture: Do lipid-raft phase boundaries depend on the sample preparation procedure? Langmuir 23:11968–11971.
- Chachaty C, Rainteau D, Tessier C, Quinn PJ, Wolf C. 2005. Building up of the liquid-ordered phase formed by sphingomyelin and cholesterol. Biophys J 88:4032–4044.
- de Almeida RF, Fedorov A, Prieto M. 2003. Sphingomyelin/phosphatidylcholine/cholesterol phase diagram: Boundaries and composition of lipid rafts. Biophys J 85:2406–2416.
- Dietrich C, Bagatolli LA, Volovyk ZN, Thompson NL, Levi M, Jacobson K, 2001. Lipid rafts reconstituted in model membranes. Biophys J 80:1417–1428.
- Edidin M. 2003. The state of lipid rafts: From model membranes to cells. Annu Rev Biophys Biomol Struct 32:257–283.
- Epand RM, Bach D, Borochov N, Wachtel E. 2000. Cholesterol crystalline polymorphism and the solubility of cholesterol in phosphatidylserine. Biophys J 78:866–873.
- Epand RM, Sayer BG, Epand RF. 2003. Peptide-induced formation of cholesterol-rich domains. Biochemistry 42:14677–14689.
- Funari SS, Barceló F, Escriba PV. 2003. Effects of oleic acid and its congeners, elaidic and stearic acids, on the structural properties of phosphatidylethanolamine membranes. J Lipid Res 44:567–575.
- Gandhavadi M, Allende D, Vidal A, Simon SA, Mcintosh TJ. 2002. Structure, composition, and peptide binding properties of detergent soluble bilayers and detergent resistant rafts. Biophys J 82:1469–1482.
- Huang J, Buboltz JT, Feigenson GW. 1999. Maximum solubility of cholesterol in phosphatidylcholine and phosphatidylethanolamine bilayers. Biochim Biophys Acta 1417:89–100.
- Kahya N, Scherfeld D, Bacia K, Poolman B, Schwille P. 2003. Probing lipid mobility of raft-exhibiting model membranes by fluorescence correlation spectroscopy. J Biol Chem 278:28109–28115.
- Knoll W, Schmidt G, Ibel K, Sackmann E. 1985. Small-angle neutron scattering study of lateral phase separation in dimyristoylphosphatidylcholine-cholesterol mixed membranes. Biochemistry 24:5240–5246.
- Lawrence JC, Saslowsky DE, Edwardson JM, Henderson RM. 2003. Real-time analysis of the effects of cholesterol on lipid raft behavior using atomic force microscopy. Biophys J 84:1827–1832.
- Lingwood D, Simons K. 2010. Lipid rafts as a membrane-organizing principle. Science 327:46–50.
- London E, Brown DA. 2000. Insolubility of lipids in triton X-100: Physical origin and relationship to sphingolipid/cholesterol membrane domains (rafts). Biochim Biophys Acta 1508:182–195.
- Megha London E. 2004. Ceramide selectively displaces cholesterol from ordered lipid domains (rafts): Implications for lipid raft structure and function. J Biol Chem 279:9997–10004.
- Mills TT, Tristram-Nagle S, Heberle FA, Morales NF, Zhao J, Wu J, 2008. Liquid-liquid domains in bilayers detected by wide angle X-ray scattering. Biophys J 95:682–690.
- Nicolini C, Kraineva J, Khurana M, Periasamy N, Funari SS, Winter R. 2006. Temperature and pressure effects on structural and conformational properties of POPC/SM/cholesterol model raft mixtures – a FT-IR, SAXS, DSC, PPC and Laurdan fluorescence spectroscopy study. Biochim Biophys Acta 1758:248–258.
- Pandit SA, Vasudevan S, Chiu SW, Mashl RJ, Jakobsson E, Scott HL. 2004. Sphingomyelin-cholesterol domains in phospholipid membranes: Atomistic simulation. Biophys J 87:1092–1100.
- Pare C, Lafleur M. 1998. Polymorphism of POPE/cholesterol system: A 2H nuclear magnetic resonance and infrared spectroscopic investigation. Biophys J 74:899–909.
- Parker A, Miles K, Cheng KH, Huang J. 2004. Lateral distribution of cholesterol in dioleoylphosphatidylcholine lipid bilayers: Cholesterol-phospholipid interactions at high cholesterol limit. Biophys J 86:1532–1544.
- Prades J, Alemany R, Perona JS, Funari SS, Vogler O, Ruiz-Gutierrez V, 2008. Effects of 2-hydroxyoleic acid on the structural properties of biological and model plasma membranes. Mol Membr Biol 25:46–57.
- Quinn PJ, Wolf C. 2009. Thermotropic and structural evaluation of the interaction of natural sphingomyelins with cholesterol. Biochim Biophys Acta 1788:1877–1889.
- Quinn PJ, Wolf C. 2010. An X-ray diffraction study of model membrane raft structures. FEBS J 277:4685–4698.
- Ramstedt B, Slotte JP. 2006. Sphingolipids and the formation of sterol-enriched ordered membrane domains. Biochim Biophys Acta 1758:1945–1956.
- Ratajczak MK, Ko YT, Lange Y, Steck TL, Lee KY. 2007. Cholesterol displacement from membrane phospholipids by hexadecanol. Biophys J 93:2038–2047.
- Rinia HA, De Kruijff B. 2001. Imaging domains in model membranes with atomic force microscopy. FEBS Lett 504:194–199.
- Rinia HA, Snel MM, Van Der Eerden JP, De Kruijff B. 2001. Visualizing detergent resistant domains in model membranes with atomic force microscopy. FEBS Lett 501:92–96.
- Silvius JR. 2003. Role of cholesterol in lipid raft formation: Lessons from lipid model systems. Biochim Biophys Acta 1610:174–183.
- Simons K, Toomre D. 2000. Lipid rafts and signal transduction. Nat Rev Mol Cell Biol 1:31–39.
- Simons K, Vaz WL. 2004. Model systems, lipid rafts, and cell membranes. Annu Rev Biophys Biomol Struct 33:269–295.
- Stockl M, Plazzo AP, Korte T, Herrmann A. 2008. Detection of lipid domains in model and cell membranes by fluorescence lifetime imaging microscopy of fluorescent lipid analogues. J Biol Chem 283:30828–30837.
- Takahashi H, Sinoda K, Hatta I. 1996. Effects of cholesterol on the lamellar and the inverted hexagonal phases of dielaidoylphosphatidylethanolamine. Biochim Biophys Acta 1289:209–216.
- Van Duyl BY, Ganchev D, Chupin V, De Kruijff B, Killian JA. 2003. Sphingomyelin is much more effective than saturated phosphatidylcholine in excluding unsaturated phosphatidylcholine from domains formed with cholesterol. FEBS Lett 547:101–106.
- Veatch SL, Keller SL. 2005. Miscibility phase diagrams of giant vesicles containing sphingomyelin. Phys Rev Lett 94:148101.
- Veatch SL, Polozov IV, Gawrisch K, Keller SL. 2004. Liquid domains in vesicles investigated by NMR and fluorescence microscopy. Biophys J 86:2910–2922.
- Vogler O, Casas J, Capo D, Nagy T, Borchert G, Martorell G, 2004. The Gbetagamma dimer drives the interaction of heterotrimeric Gi proteins with nonlamellar membrane structures. J Biol Chem 279:36540–36545.