Abstract
Protein transport via the Sec translocon represents an evolutionary conserved mechanism for delivering cytosolically-synthesized proteins to extra-cytosolic compartments. The Sec translocon has a three-subunit core, termed Sec61 in Eukaryotes and SecYEG in Bacteria. It is located in the endoplasmic reticulum of Eukaryotes and in the cytoplasmic membrane of Bacteria where it constitutes a channel that can be activated by multiple partner proteins. These partner proteins determine the mechanism of polypeptide movement across the channel. During SRP-dependent co-translational targeting, the ribosome threads the nascent protein directly into the Sec channel. This pathway is in Bacteria mainly dedicated for membrane proteins but in Eukaryotes also employed by secretory proteins. The alternative pathway, leading to post-translational translocation across the Sec translocon engages an ATP-dependent pushing mechanism by the motor protein SecA in Bacteria and a ratcheting mechanism by the lumenal chaperone BiP in Eukaryotes. Protein transport and biogenesis is also assisted by additional proteins at the lateral gate of SecY/Sec61α and in the lumen of the endoplasmic reticulum or in the periplasm of bacterial cells. The modular assembly enables the Sec complex to transport a vast array of substrates. In this review we summarize recent biochemical and structural information on the prokaryotic and eukaryotic Sec translocons and we describe the remarkably complex interaction network of the Sec complexes.
Introduction
The sorting of proteins between different cellular compartments is mediated by a large diversity of protein transport systems (Lithgow & Waksman, Citation2013; Papanikou et al., Citation2007). In prokaryotes, the cytoplasmic membrane is responsible for asymmetric protein distribution between the cytosol and periplasmic space or the outer membrane in Gram-negative Bacteria. Targeting, sorting and transport systems in Eukaryotes are more complex, owing to the presence of organelles. The protein transport gateway to most organelles (secretory pathway) is the endoplasmic reticulum (ER) and the Sec translocon constitutes the major protein transport site in the ER membrane. The Sec translocon is also universally conserved in the cytoplasmic membrane of Bacteria and Archaea (Kudva et al., Citation2013; Zimmermann et al., Citation2011). In addition, the Sec translocon is present in the thylakoid membrane in chloroplasts, but absent in most mitochondria with the exception of certain protozoans (Albiniak et al., Citation2012; Tong et al., Citation2011).
Although the Sec translocon primarily functions as an aqueous conduit for proteins, it differs from most other pores as its channel forming subunit (SecY/Sec61α) is able to open both transversally into the periplasm/ER lumen and also laterally to the lipid membrane (). Transverse opening facilitates protein translocation across the membrane, whereas lateral opening of the translocon allows lipid insertion of membrane proteins. The lateral gate of the translocon is composed of four flexible α-helices of the SecY/Sec61α subunit (Van den Berg et al., Citation2004). The translocation of secretory proteins and large hydrophilic domains of membrane proteins through the SecY/Sec61 pore requires ATP hydrolysis by the ATPase SecA on the cis side of the bacterial membrane or by chaperones like BiP on the trans side of the ER membrane (Kudva et al., Citation2013; Zimmermann et al., Citation2011). The proton motive force (pmf) also contributes to the energetics of protein translocation across the bacterial membrane (van der Laan et al., Citation2004) although it is not essential for protein transport in vitro (Koch & Müller, Citation2000).
Figure 1. Protein targeting pathways in bacterial and mammalian cells (A) Bacteria engage two targeting pathways for delivering proteins to the SecYEG translocon. The SecA-dependent pathway is used by periplasmic and outer membrane proteins, which contain a cleavable signal sequence. Cytosolic chaperones, including trigger factor and tetrameric SecB keep the nascent polypeptide in a translocation-competent state during its journey to the membrane. The pre-protein is then transferred to SecA, which drives translocation through the SecYEG channel by ATP hydrolysis. Although it is generally assumed that SecA acts post-translationally, some data indicate that SecA can bind to ribosome-nascent chains (RNCs), i.e. that it can also act co-translationally. The SecYEG translocon interacts at least transiently with the SecDFYajC complex, which might support the proton-motif force (pmf)-dependent steps during protein transport. The co-translational SRP-pathway is mainly used for inner membrane proteins and initiated by the ribosome-bound SRP. SRP-RNCs bind to the SecYEG-bound SRP receptor FtsY, RNCs dock onto the SecYEG translocon and the SRP-FtsY complex dissociates in a GTP-dependent manner. During the lateral exit from the SecYEG channel, the nascent membrane protein contactsYidC. YidC is shown to support SecYEG during membrane protein insertion, and it also acts as a SecYEG independent insertase for small or closely spaced membrane proteins. Targeting of membrane proteins to YidC also appears to be SRP-dependent. The translocon associates transiently with several additional proteins, that are required for cleaving the signal sequences of secretory proteins (signal peptidase, SPase), for protein folding (the periplasmic chaperones Skp and PpiD) or for quality control (the membrane-bound protease FtsH). (B) In eukaryotes, the Sec61-mediated insertion and the translocation occurs both co-translationally and post-translationally. During post-translational targeting, fully synthesized pre-proteins are kept in a transport-competent state by members of the Hsp90, Hsp70 and Hsp40 chaperone families. Translocation is mediated by the Sec61 complex in association with Sec62/Sec63 and the chaperone BiP at the lumenal side of the ER membrane. BiP binds to the translocating substrates in the ER lumen and prevents their back-sliding by an ATP-dependent ratcheting mechanism. The eukaryotic SRP pathway delivers both membrane proteins and secretory proteins co-translationally to the Sec61 complex. The eukaryotic SRP receptor consists of two unrelated GTPases, SRα (homologous to FtsY) and SRβ. The Sec61 translocon associates in a substrate-dependent manner with additional proteins that either bind to RNCs (Ramp4) or are suggested to assist membrane protein folding (TRAM, Sec63). BiP is also required for co-translational transport. Like in bacteria, additional proteins are involved in processing and quality control (SPase; TRAP [translocon associated protein], oligosacharyl transferase [OST], the Hsp40-homologue Erj1 or AAA-proteases). This Figure is reproduced in color in the online version of Molecular Membrane Biology.
![Figure 1. Protein targeting pathways in bacterial and mammalian cells (A) Bacteria engage two targeting pathways for delivering proteins to the SecYEG translocon. The SecA-dependent pathway is used by periplasmic and outer membrane proteins, which contain a cleavable signal sequence. Cytosolic chaperones, including trigger factor and tetrameric SecB keep the nascent polypeptide in a translocation-competent state during its journey to the membrane. The pre-protein is then transferred to SecA, which drives translocation through the SecYEG channel by ATP hydrolysis. Although it is generally assumed that SecA acts post-translationally, some data indicate that SecA can bind to ribosome-nascent chains (RNCs), i.e. that it can also act co-translationally. The SecYEG translocon interacts at least transiently with the SecDFYajC complex, which might support the proton-motif force (pmf)-dependent steps during protein transport. The co-translational SRP-pathway is mainly used for inner membrane proteins and initiated by the ribosome-bound SRP. SRP-RNCs bind to the SecYEG-bound SRP receptor FtsY, RNCs dock onto the SecYEG translocon and the SRP-FtsY complex dissociates in a GTP-dependent manner. During the lateral exit from the SecYEG channel, the nascent membrane protein contactsYidC. YidC is shown to support SecYEG during membrane protein insertion, and it also acts as a SecYEG independent insertase for small or closely spaced membrane proteins. Targeting of membrane proteins to YidC also appears to be SRP-dependent. The translocon associates transiently with several additional proteins, that are required for cleaving the signal sequences of secretory proteins (signal peptidase, SPase), for protein folding (the periplasmic chaperones Skp and PpiD) or for quality control (the membrane-bound protease FtsH). (B) In eukaryotes, the Sec61-mediated insertion and the translocation occurs both co-translationally and post-translationally. During post-translational targeting, fully synthesized pre-proteins are kept in a transport-competent state by members of the Hsp90, Hsp70 and Hsp40 chaperone families. Translocation is mediated by the Sec61 complex in association with Sec62/Sec63 and the chaperone BiP at the lumenal side of the ER membrane. BiP binds to the translocating substrates in the ER lumen and prevents their back-sliding by an ATP-dependent ratcheting mechanism. The eukaryotic SRP pathway delivers both membrane proteins and secretory proteins co-translationally to the Sec61 complex. The eukaryotic SRP receptor consists of two unrelated GTPases, SRα (homologous to FtsY) and SRβ. The Sec61 translocon associates in a substrate-dependent manner with additional proteins that either bind to RNCs (Ramp4) or are suggested to assist membrane protein folding (TRAM, Sec63). BiP is also required for co-translational transport. Like in bacteria, additional proteins are involved in processing and quality control (SPase; TRAP [translocon associated protein], oligosacharyl transferase [OST], the Hsp40-homologue Erj1 or AAA-proteases). This Figure is reproduced in color in the online version of Molecular Membrane Biology.](/cms/asset/71c0cfd0-da83-4248-b264-29242716567a/imbc_a_907455_f0001_b.jpg)
A major challenge during protein transport is preventing premature and unproductive folding of proteins before they reach the Sec translocon. This is especially the case for hydrophobic membrane proteins which are aggregation-prone. Targeting of many proteins therefore begins at the ribosome during translation. Co-translational targeting is initiated by the signal recognition particle (SRP), which binds to the tunnel exit of the ribosome to recognize its substrate early during synthesis (Berndt et al., Citation2009; Bornemann et al., Citation2008). SRP-ribosome-associated nascent chains (SRP-RNCs) are delivered to the membrane-bound SRP receptor (SR), and the ribosome thereafter aligns with the channel of the Sec translocon to facilitate co-translational transport of the nascent polypeptide (Gilmore et al., Citation1982b; Koch et al., Citation1999; Valent et al., Citation1998). In bacteria, the SRP pathway is mainly dedicated to the targeting of inner membrane proteins, while the eukaryotic SRP delivers both ER membrane proteins and secretory proteins to the Sec61 complex.
The Sec translocon also transports proteins post-translationally, i.e. upon termination of protein synthesis. In bacteria, the post-translational mode is preferentially employed by periplasmic and outer membrane proteins and involves the cytosolic ATPase SecA (Koch et al., Citation1999; Koch & Müller, Citation2000). SecA also cooperates with the SRP pathway for the insertion of membrane proteins with periplasmic domains longer than approx. 30 amino acids (Deitermann et al., Citation2005; Neumann-Haefelin et al., Citation2000; Sääf et al., Citation2009). In eukaryotes, post-translational transport requires the association of Sec61 with the Sec62/Sec63 complex (Meyer et al., Citation2000; Panzner et al., Citation1995).
The folding and processing of transported proteins is facilitated by periplasmic and lumenal chaperones (Gordon & Kindt, Citation1976; Missiakas et al., Citation1996), signal peptidases (Chang et al., Citation1978; Zwizinski & Wickner, Citation1980) and oligosaccharyl transferases (Lau et al., Citation1983). The final topology of membrane proteins is further affected by the lipid composition of the membrane (Dowhan & Bogdanov, Citation2009). The dynamic interplay of the core translocon with many additional factors is common to both prokaryotes and eukaryotes, and this probably ensures efficient transport and biogenesis of a vast array of substrates.
This review provides an insight to the current knowledge on the Sec translocon. Most of the data on the prokaryotic Sec translocon is based on the Gram-negative model organism E. coli. For reviews covering protein translocation in Archaea and Gram positive bacteria please see Pohlschröder et al. (Citation2005), Calo & Eichler (Citation2011) and Yuan et al. (Citation2010).
Composition and architecture of the core Sec complex
Most components of the Sec pathway were identified from genetic screens conducted in E. coli and Saccharomyces cerevisiae (Deshaies & Schekman, Citation1987; Emr et al., Citation1981; Oliver & Beckwith, Citation1981). Mutations that caused protein secretion defects were referred to as sec alleles and mapped to secA, secD, secE, secF and secY in E.coli; prl mutations (suppressors of signal sequence mutations) allowed the secretion of proteins with defective signal sequences and were mapped to secA (prlD), secE (prlG), secG (prlH) and secY (prlA) (Bieker & Silhavy, Citation1990; Emr et al., Citation1981, Ito et al., Citation1983; Oliver & Beckwith, Citation1981; Schatz & Beckwith, Citation1990). The three-dimensional crystal structure of the Sec translocon from the archaeon Methanocaldococcus janaschii confirmed many structural and functional predictions that were based on these early genetic screens (Smith et al., Citation2005; Van den Berg et al., Citation2004). Subsequent studies found that the general architecture observed for the Methanocaldococcus jananschii Sec complex appears to be universally conserved in both prokaryotes and eukaryotes (Becker et al., Citation2009; Clemons et al., Citation2004; Egea & Stroud, Citation2010; Frauenfeld et al., Citation2011; Gogala et al., Citation2014; Gumbart et al., Citation2009; Li et al., Citation2007; Ménétret et al., Citation2005; Citation2007; Citation2008; Mitra et al., Citation2005; Park et al., Citation2014; Tsukazaki et al., Citation2008; Zimmer et al., Citation2008).
The core Sec translocon consists of three protein subunits – SecY, SecE and SecG in bacteria, and Sec61α, Sec61γ and Sec61β in eukaryotes (Zimmermann et al., Citation2011). SecYE and Sec61αγ exhibit significant sequence conservation and are essential (Kudva et al., Citation2013; Park & Rapoport, Citation2012). The Sec61β subunit found in Eukaryotes and Archaea is not homologous to the eubacterial SecG subunit, and neither Sec61β nor SecG are essential for protein transport (Finke et al., Citation1996; Nishiyama et al., Citation1994).
SecY and Sec61α are comprised of 10 transmembrane α-helical domains (TMs) each and have similar molecular masses, i.e. 48 kDa for E. coli SecY and 52 kDa for Homo sapiens Sec61α (Rensing & Maier, Citation1994). When visualized from the top, the 10 TMs are divided into two halves that resemble a clamshell surrounding a central pore (; Van den Berg et al., Citation2004). The two halves (TMs 1–5 and 6–10) are connected by a periplasmic loop, which is referred to as the hinge region or the back of the translocon. A side section through the SecYEβ complex reveals an hourglass-shaped structure with two funnels, one opening to the cytoplasmic face and the other one to the periplasmic face of the membrane (). The two funnels are separated by a central constriction, which is called the pore ring. It is comprised of amino acid residues with bulky hydrophobic side chains, e.g. by six isoleucine residues in E. coli SecY (Van den Berg et al., Citation2004). The translocon assumes a closed conformation in the resting state: the cytoplasmic funnel is empty and its periplasmic counterpart is plugged by a short α-helix (Helix 2a or plug) (Tsukazaki et al., Citation2008; Van den Berg et al., Citation2004). Mutagenesis studies and structural data suggest that the central constriction and the plug may play a role in the controlled opening and closing of the Sec pore (Egea & Stroud, Citation2010; Harris & Silhavy, Citation1999; Van den Berg et al., Citation2004). Molecular dynamics simulations support the view that the plug participates in sealing the pore and maintaining substrate selectivity of the translocon (Gumbart & Schulten, Citation2008). However, deleting the plug domain of the Sec channel does not affect growth of either E. coli or S. cerevisiae (Junne et al., Citation2006; Li et al., Citation2007; Maillard et al., Citation2007) although it decreases the selectivity of the translocon for its substrates (Li et al., Citation2007; Maillard et al., Citation2007). The X-ray structure of the plug-less SecYE channel shows that neighbouring residues can replace the function of the plug (Li et al., Citation2007).
Figure 2. The Sec translocon. Schematic representation of the Sec translocon in the closed (A) and the open (B) conformation viewed from the front in the membrane plane (left), as a transverse section through the middle of the pore in the membrane plane (middle) and from the cytoplasmic side (top, right). The open and the closed conformation refer to the lateral gate being closed or open as shown in the front and top representation, respectively. The transverse section and the top view show the pore ring and the plug being displaced for the accommodation of a substrate. (C) Surface representation of the Archaeal SecYEβ translocon in the plane of the membrane (adapted from Van den Berg et al. [Citation2004]; pdb: 1RHZ). The lateral gate helices (TM2b, TM3, TM7 and TM8) of SecY and a short helix (helix 2a), called the plug, are highlighted. The plug is suggested to be involved in sealing the channel. The cytoplasmic loops C4, C5 and C6 of SecY are the major cytoplasmic contact sites for FtsY, SecA and the ribosome. (D) The top view of Sec61YEβ from the cytoplasmic site shows the plug (dark green) sealing the channel and SecE embracing SecY at the back. This Figure is reproduced in color in the online version of Molecular Membrane Biology.
![Figure 2. The Sec translocon. Schematic representation of the Sec translocon in the closed (A) and the open (B) conformation viewed from the front in the membrane plane (left), as a transverse section through the middle of the pore in the membrane plane (middle) and from the cytoplasmic side (top, right). The open and the closed conformation refer to the lateral gate being closed or open as shown in the front and top representation, respectively. The transverse section and the top view show the pore ring and the plug being displaced for the accommodation of a substrate. (C) Surface representation of the Archaeal SecYEβ translocon in the plane of the membrane (adapted from Van den Berg et al. [Citation2004]; pdb: 1RHZ). The lateral gate helices (TM2b, TM3, TM7 and TM8) of SecY and a short helix (helix 2a), called the plug, are highlighted. The plug is suggested to be involved in sealing the channel. The cytoplasmic loops C4, C5 and C6 of SecY are the major cytoplasmic contact sites for FtsY, SecA and the ribosome. (D) The top view of Sec61YEβ from the cytoplasmic site shows the plug (dark green) sealing the channel and SecE embracing SecY at the back. This Figure is reproduced in color in the online version of Molecular Membrane Biology.](/cms/asset/54f60bcf-5cb1-4131-a4c2-373d40d5271a/imbc_a_907455_f0002_b.jpg)
The exit of TMs into the lipid phase is facilitated by structural rearrangements in the lateral gate of the SecY/Sec61α channel and involves TMs 2b and 3 on one side and helices 7 and 8 on the other side of SecY (Hizlan et al. (Citation2012), ). Signal sequences might be maintained at the lateral gate since cross-linking data have shown that signal sequences contact lipids during insertion (Higy et al., Citation2005; Martoglio et al., Citation1995) and that they are intercalated between transmembrane helices 2 and 7 of SecY/Sec61α (Plath et al., Citation1998).
Most bacteria have a SecE molecule with only one TM. In contrast, E. coli SecE consists of three TMs and has a molecular mass of 14 kDa. However, only the third TM of SecE is essential for protein transport and cell viability (Schatz et al., Citation1991). In eukaryotes, including H. sapiens, Sec61γ is a single-spanning membrane protein of approx. 8 kDa (Hartmann et al., Citation1994). SecE is located at the back of SecY () stabilizing the two halves of SecY (Van den Berg et al., Citation2004). Indeed, SecY molecules that have been proteolytically cleaved at the hinge region remain active if SecE is present (Lycklama a Nijeholt et al., Citation2013). SecY in E. coli is rapidly degraded by the membrane protease FtsH in the absence of SecE (Kihara et al., Citation1995).
SecG in E. coli is a 12 kDa protein consisting of two TMs connected by a cytoplasmic loop. Cross-linking studies have located SecG next to the cytosolic loops C2 and C3 of SecY (Satoh et al., Citation2003; van der Sluis et al., Citation2002), although SecG/Sec61β are thought to have only limited contacts to SecY and SecE (Van den Berg et al., Citation2004; Zimmer et al., Citation2008). SecG is not essential for protein transport in vitro (Brundage et al., Citation1990) but SecG deletion strains exhibit protein transport defects in vivo (Flower, Citation2001; Flower et al., Citation2000). In E. coli, the function of SecG has been linked to the SecA-dependent post-translational transport across the Sec channel (Duong & Wickner, Citation1997b; Morita et al., Citation2012; Nishiyama et al., Citation1996). As SecA is absent in Eukaryotes with the exception of chloroplasts and since it is also not found in Archaea, a functional connection between SecA and SecG would explain its presence in Bacteria only. SecG has been proposed to undergo reversible topology inversions for facilitating SecA-SecY interaction (Morita et al., Citation2012; Nishiyama et al., Citation2012; Sugai et al., Citation2007). Although several dual topology proteins have been identified in E. coli (Daley et al., Citation2005; Rapp et al., Citation2006), a topologically fixed SecG derivative does not prevent SecA-dependent protein translocation (van der Sluis et al., Citation2006). Thus, the physiological significance of the topology switch needs to be further analysed. Recent data suggest that the orientation of SecG depends on a non-proteinaceous glycolipozyme that was shown to influence membrane protein insertion and translocation (Moser et al., Citation2013).
The non-homologous Sec61β in Eukaryotes and Archaea is slightly smaller than SecG and contains only one TM (Hartmann et al., Citation1994; Kalies et al., Citation1998). Sec61β was shown to interact with the SRP receptor (Helmers et al., Citation2003) and with the signal peptidase in yeast (Kalies et al., Citation1998). Furthermore, the role of Sec61β might not be limited to translocation since it interacts with Rtn1p, a protein involved in ER tubule formation (Zhao & Jäntti, Citation2009), and it appears to be required for plasma membrane targeting of Gurken, the ligand of epidermal growth factor receptor in Drosophila (Kelkar & Dobberstein, Citation2009).
Additional subunits, partner proteins and the membrane environment of the bacterial and eukaryotic Sec complex
The Sec complex has a modular nature. Some of the interactions of the core Sec translocon have been observed in all domains of life, e.g. with ribosomes, the SRP receptor and signal peptidases; yet many interactions are characteristic to either Bacteria or Eukaryotes. A number of proteins have been shown to interact at least transiently with the Sec translocon (). Attempts to determine the structure of a holo-translocon (Duong & Wickner, Citation1997b) comprising several modules attached to the Sec core complex have been successful for SecA-SecYE complexes (Zimmer et al., Citation2008) and for ribosome-SecYEG/ribosome-Sec61 complexes (Frauenfeld et al., Citation2011; Gogala et al., Citation2014; Ménétret et al., Citation2005, Citation2007, Citation2008; Mitra et al., Citation2005; Park & Rapoport, Citation2012; Park et al., Citation2014). The Sec translocon interactions with additional partners depend on the nature of the substrate and therefore the exact composition of the holo-translocon is probably rather flexible in vivo.
Table 1. Sec-translocon associated proteins and their conservation. Dark grey represents the proteins present in all or most species; light grey represents the proteins found in some species; blank – no known homologue. The paralogues are not indicated.
The interaction of ribosomes with Sec complex
The ability of the Sec complex to bind to ribosomes is an essential feature and ribosome binding sites on the translocon are evolutionarily conserved (Becker et al., Citation2009; Frauenfeld et al., Citation2011; Houben et al., Citation2005; Prinz et al., Citation2000). The cytosolic loops of SecY/Sec61α between TM 6 and 7 (C4 loop) and TM 8 and 9 (C5 loop) mediate ribosome binding (Cheng et al., Citation2005; Frauenfeld et al., Citation2011; Kuhn et al., Citation2011; Citation2014; Park & Rapoport, Citation2012; Park et al., Citation2014; Raden et al., Citation2000). The universal ribosome adaptor site consisting of the proteins L23, L24 and L29 (E. coli nomenclature, ), and conserved rRNA helices, establish contacts to the Sec translocon both in prokaryotes and eukaryotes (Becker et al., Citation2009; Frauenfeld et al., Citation2011). Recent comparative cryo-EM reconstructions show that both translating and non-translating ribosomes provide the same binding sites for the translocon although rather large conformational changes take place within the translocon upon substrate binding (Gogala et al., Citation2014; Park et al., Citation2014).
Figure 3. The ribosomal tunnel exit as a binding platform for targeting factors, chaperones, nascent chain processing enzymes and the translocon. L23, L24 and L29 constitute a universal ribosomal adaptor site. Data are collected from: TF and peptidyl formylase (PDF): (Kramer et al., Citation2002; Bingel-Erlenmeyer et al., Citation2008); SecY: (Frauenfeld et al., Citation2011); SecA: (Huber et al., Citation2011); SRP: (Gu et al., Citation2003; Halic et al., Citation2006; Schaffitzel et al., Citation2006); methionine amino peptidase (MAP): (Sandikci et al., Citation2013); YidC: (Köhler et al., Citation2009; Seitl et al., Citation2013; Welte et al., Citation2012). This Figure is reproduced in color in the online version of Molecular Membrane Biology.
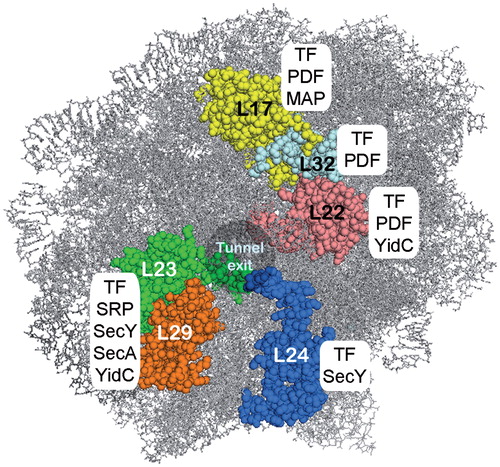
The tunnel exit area of the ribosome contacts not only the Sec translocon, but acts as the binding platform for SRP, SecA, trigger factor, and nascent chain modifying enzymes (), (Baram et al., Citation2005; Ferbitz et al., Citation2004; Frauenfeld et al., Citation2011; Gu et al., Citation2003; Huber et al., Citation2011; Kramer et al., Citation2002; Kramer et al., Citation2009; Kuhn et al., Citation2011; Schlünzen et al., Citation2005; Ullers et al., Citation2003). Similarly, the corresponding area of the eukaryotic ribosome also controls the binding of SRP, methionine aminopeptidase 1 and the chaperones NAC (nascent chain associated complex) and Ssb1/2 in a substrate-specific manner (Raue et al., Citation2007). It is not clear how binding of that many ribosomal tunnel exit ligands is coordinated in space and time.
The interaction of other ribosomal regions with the membrane might also facilitate the contact to the Sec translocon. One such region is the eukaryotic ribosome expansion segment 27 (ES27L) which has been shown to contact the ER membrane by in situ cryo-EM tomography in canine pancreas microsomes (Pfeffer et al., Citation2012). Conformational rearrangements of ES27L might play a role in ribosome release from the ER membrane (Pfeffer et al., Citation2012). Whether ES27L interacts with the membrane lipids or proteins is not yet clear but its flexibility suggests that it might respond to events on the ribosomal tunnel exit.
The interaction of the SRP receptor with Sec complex
The transfer of RNCs from the SRP to the Sec complex is the final and most crucial step during co-translational targeting. Biochemical and genetic evidence suggest that membrane-bound SR interacts directly with the Sec61 complex (Jiang et al., Citation2008; Song et al., Citation2000). The bacterial SR is termed FtsY and it is homologous to the eukaryotic SRα subunit (Luirink et al., Citation1994). FtsY and SRα belong to the SIMIBI family of GTPases harboring a characteristic NG-domain (). However, both proteins use different strategies for membrane binding. The X-domain of SRα dimerizes with SRβ, an integral 30 kDa ER membrane protein present only in Eukaryotes () (Schwartz & Blobel, Citation2003; Schlenker et al., Citation2006). SRβ belongs to the Ras superfamily of small GTPases and it requires GTP-activation to allow stable SRα binding (Fulga et al., Citation2001; Schwartz & Blobel, Citation2003). The observation that Sec61 regulates the nucleotide occupancy of SRβ has led to the idea that Sec61β functions as nucleotide exchange factor for SRβ (Helmers et al., Citation2003). The interaction with Sec61β could keep SRβ in its GTP-bound state, which would prime it for the subsequent interaction with SRα.
Figure 4. Structure of the signal recognition particle (SRP) and its receptor (SR) (A) Cryo-EM reconstitution of the eukaryotic SRP with the conserved SRP54 subunit, the additional eukaryotic SRP subunits and the 7.5 S RNA (adapted from: (Halic et al., Citation2004); pdb: 1RY1). (B) Crystal structure of the prokaryotic SRP (adapted from (Ataide et al., Citation2011); pdb: 2XXA). The conserved protein subunit Ffh (fifty-four homologue) and the 4.5 S RNA. (C) Crystal structure of the NG-subunit of the bacterial SRP receptor FtsY (adapted from (Ataide et al., Citation2011); pdb: 2XXA) (D) Complex of the prokaryotic SRP and the NG-domain of FtsY (adapted from (Ataide et al., Citation2011); pdb: 2XXA) (E) Crystal structure of the eukaryotic X-domain of SRα in complex with the cytoplasmic domain of SRβ (adapted from Schwartz & Blobel [Citation2003]; pdb: 1NRJ). This Figure is reproduced in color in the online version of Molecular Membrane Biology.
![Figure 4. Structure of the signal recognition particle (SRP) and its receptor (SR) (A) Cryo-EM reconstitution of the eukaryotic SRP with the conserved SRP54 subunit, the additional eukaryotic SRP subunits and the 7.5 S RNA (adapted from: (Halic et al., Citation2004); pdb: 1RY1). (B) Crystal structure of the prokaryotic SRP (adapted from (Ataide et al., Citation2011); pdb: 2XXA). The conserved protein subunit Ffh (fifty-four homologue) and the 4.5 S RNA. (C) Crystal structure of the NG-subunit of the bacterial SRP receptor FtsY (adapted from (Ataide et al., Citation2011); pdb: 2XXA) (D) Complex of the prokaryotic SRP and the NG-domain of FtsY (adapted from (Ataide et al., Citation2011); pdb: 2XXA) (E) Crystal structure of the eukaryotic X-domain of SRα in complex with the cytoplasmic domain of SRβ (adapted from Schwartz & Blobel [Citation2003]; pdb: 1NRJ). This Figure is reproduced in color in the online version of Molecular Membrane Biology.](/cms/asset/9ffad4e3-4739-4391-a9a3-70a24f14a7b1/imbc_a_907455_f0004_b.jpg)
There is no SRβ-homologue present in bacterial membranes and as FtsY does not have an X-domain, it uses both its NG domain and an enterobacteria-specific A-domain for membrane attachment. FtsY binds to negatively charged phospholipids and to the cytosolic loops of SecY (Angelini et al., Citation2005; Citation2006; Braig et al., Citation2009; Kuhn et al., Citation2011; Parlitz et al., Citation2007). In E. coli, FtsY is present in large excess over SecYEG and it is likely that a large portion of the SecYEG translocons are in contact with FtsY (Drew et al., Citation2003; Kudva et al., Citation2013). Importantly, the same conserved residues of SecY that are in contact with FtsY also bind to the ribosome (Kuhn et al., Citation2011). Therefore, it appears likely that FtsY occupies the ribosome binding site of SecY until it is displaced by SRP-RNCs. FtsY also competes with SecA for SecYEG binding (Kuhn P, Koch HG, unpublished work), but it is unknown how access of FtsY or SecA to SecYEG is regulated in vivo. Although the A-domain of FtsY has been shown to interact with SecY, deleting the A-domain reduces the efficiency of co-translational targeting only moderately (Eitan & Bibi, Citation2004; Weiche et al., Citation2008). In contrast, deleting the two lipid-binding helices in the N-terminus and at the interface of A and N domains of FtsY completely inhibits co-translational targeting (Parlitz et al., Citation2007; Weiche et al., Citation2008). This could indicate that membrane attachment of FtsY and not its ability to bind to SecY is crucial for its function (Mircheva et al., Citation2009). However, a second SecY binding site is proposed to exist within the NG-domain of FtsY, which could facilitate its binding to SecY independently of the A-domain (Kuhn et al., Citation2011). In addition, the membrane around the bacterial Sec complex is likely enriched with phosphatidylglycerol and cardiolipin, which are required for SecYEG activity (Gold et al., Citation2010). As FtsY also binds preferentially to negative phospholipids (Braig et al., Citation2009), sufficient amounts of FtsY are probably located in close proximity to the SecYEG complex even in the absence of the A-domain.
The interaction of signal peptidase with Sec complex
The majority of non-cytosolic proteins originally bear a signal sequence which is recognized by targeting factors. After the pre-protein is targeted to the translocase, the signal sequence is eventually removed by membrane-embedded signal peptidases (SPases). The signal peptide is subsequently degraded by the membrane bound signal peptide peptidases (SPPase) (Nam & Paetzel, Citation2013; Voss et al., Citation2013; Wang et al., Citation2008) and the amino acids are recycled.
Many different SPases are found in all domains of life. Prokaryotic SPases are classified as Spase I, II and IV (Auclair et al., Citation2012). The Spase I (LepB in E. coli) is an essential and conserved serine-protease, specific to the non-lipoprotein substrates of the Sec and Tat (twin-arginine-dependent translocation) translocons (Auclair et al., Citation2012; Nyathi et al., Citation2013). Its catalytic domain is located in the periplasm and its TMs probably assist in signal peptide processing (Paetzel et al., Citation1998). The Spase I of E. coli has two transmembrane domains while Bacillus subtilis has one (Tjalsma et al., Citation1998). Eukaryotic signal peptidases are organized in multi-subunit complexes termed SPC. However, the catalytic activity of SPC is located at LepB homologue which is Sec11 in yeast () (VanValkenburgh et al., Citation1999) and Spc18/Spc21 in mammals (Liang et al., Citation2003).
Figure 5. The topology of yeast Sec62, Sec63, Sec71, Sec72 and Sec11. The lumenal J-domain of Sec63 is important for the recruitment of BiP, a chaperone which is essential for Sec61-mediated translocation. The negatively charged C-terminus of Sec63 binds to the N-terminus of Sec62 to collectively support post-translational translocation. Sec71 and Sec72 form the complex with Sec62/Sec63. Sec11 is the catalytic subunit of the yeast signal peptidase complex. This Figure is reproduced in color in the online version of Molecular Membrane Biology.
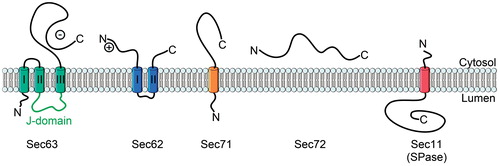
Although signal sequences of Sec substrates are cleaved off during the translocation (Josefsson & Randall, Citation1981a; Citation1981b), evidence for a direct interaction between SPase and the translocon is limited. So far, only the yeast Sec61β was shown to interact with signal peptidase during translocation (Antonin et al., Citation2000; Kalies et al., Citation1998).
The effect of the lipid environment on protein transport
The lipid bilayer constitutes the permeability barrier of the cell and influences directly the activity of multiple membrane protein complexes, including the Sec translocon. Phospholipids also affect the stability and final topology of newly synthesized membrane proteins (Dowhan & Bogdanov, Citation2009). This is achieved by the charged phospholipid head groups, their asymmetric distribution and the nature of the acyl chains.
The ER membrane and the cytoplasmic membrane of Gram-negative bacteria consist largely of zwitterionic phospholipids while Gram-positive bacteria have more anionic membrane lipids (Epand & Epand, Citation2011). A comparison of the lipid composition of E. coli membrane and the ER membrane is given in .
Table 2. The phospholipid composition of the E. coli inner membrane and the ER membrane.
The zwitterionic phospholipid phosphotidylethanolamine (PE) is important for membrane elasticity and curvature (Raetz, Citation1978). PE probably supports conformational flexibility of the Sec complex during protein transport (Rietveld et al., Citation1995) and its depletion has been shown to reduce translocation efficiency (Mikhaleva et al., Citation2001; van der Does et al., Citation2000). PE has also been suggested to affect membrane binding of FtsY (Millman et al., Citation2001).
Phospholipids with negatively charged head groups like phosphatidylglycerol (PG), phosphatidylserine (PS) and phosphatidylinositol (PI) have the most pronounced effect on membrane protein biogenesis (). The activities of both SecA and FtsY are stimulated by negatively charged phospholipids (Bahari et al., Citation2007; Braig et al., Citation2009; Lam et al., Citation2010; Lill et al., Citation1990; Parlitz et al., Citation2007) and the absence of PG severely impairs protein transport in E. coli (de Vrije et al., Citation1988; van der Does et al., Citation2000). Cardiolipin (CL) also stabilizes the SecYEG dimer and creates a high affinity binding surface for the motor protein SecA (Gold et al., Citation2010). Acidic phospholipids induce the dissociation of dimeric SecA exposing its binding interface to SecYEG (Alami et al., Citation2007). The amount of CL bound to SecYEG is proportional to the ATPase activity of SecA (Gold et al., Citation2010).
Table 3. Influence of structural lipids of the E. coli inner membrane and the ER membrane on targeting and function of Sec translocon.
Sterols seem to inhibit protein translocation initiation, most likely hindering RNC binding to Sec61 (Nilsson et al., Citation2001; Yamamoto et al., Citation2012). This is probably the reason why sterols are scarce in the ER membrane (van Meer et al., Citation2008).
The interaction network of bacterial Sec complex
Some Sec complex-associated proteins like SecA are present only in bacteria while others like periplasmic chaperones also have functional homologues in eukaryotic cells ().
SecA
SecA is (Figure 6) probably best studied partner protein of the bacterial Sec complex. It was identified in the genetic screens during the discovery of the sec and prl alleles (Emr et al., Citation1981; Oliver & Beckwith, Citation1981). It has a dual role as it acts as an ATP-fueled motor supporting protein transport across the inner membrane and as a targeting factor for the post-translational pathway.
SecA binds to SecYEG with an affinity of 20–40 nM (Douville et al., Citation1995) and is considered to function as a soluble subunit of the Sec translocon. SecA and FtsY have an overlapping binding sites on SecY, however SecA binds to additional residues on SecY that are distributed across cytosolic loops C2–C6 (Mori & Ito, Citation2006). The crystal structure of SecA in complex with the bacterial translocon (Zimmer et al., Citation2008) shows SecA:SecYEG in a 1:1 stoichometry but biochemical data also support a 1:2 or 2:2 stoichometry (Deville et al., Citation2011; Osborne & Rapoport, Citation2007). The PBD domain (peptide binding domain); also called PPXD; (pre-protein cross-linking domain) of SecA, provides the major contact site with SecY (). The PBD domain of free SecA is closely packed against the helical wing domain (HWD) (Hunt et al., Citation2002; Vassylyev et al., Citation2006) but in the SecY-bound SecA structure the PBD is rotated towards the nucleotide-binding domain 2 (NBD-2) (Zimmer et al., Citation2008). It is thought that the PBD domain functions as a flexible trap that captures the translocating substrate in a groove formed by PBD and HWD (Park & Rapoport, Citation2012). In the SecA-SecYE structure, the groove is aligned with the SecY channel, allowing the substrate to move through the groove into the channel (Zimmer et al., Citation2008).
Figure 6. Structure of SecA, the motor protein of the post-translational transport in bacteria. (A) Schematic domain organisation of SecA (NBD, Nucleotide binding domains; PBD, peptide-cross-linking domain; HSD, helical scaffold domain; HWD, helical wing domain; CTD, C-terminal domain). (B) Crystal structure of SecA from Thermotoga maritima (adapted from Zimmer et al. (Citation2008); pdb: 3DIN). The colour code is the same as in (A). (C) Crystal structure of SecA in complex with the SecYEG translocon (adapted from Zimmer et al. (Citation2008); pdb: 3DIN). The helices of the lateral gate of SecY are highlighted. This Figure is reproduced in color in the online version of Molecular Membrane Biology.
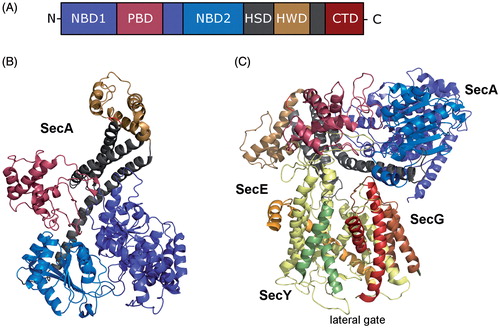
SecDFYajC
The trimeric SecDFYajC complex is a low-abundant integral membrane protein complex that was shown to interact with the SecYEG (Duong & Wickner, Citation1997b). The deletion of SecD/SecF negatively affects bacterial growth and their presence stimulates protein export (Pogliano & Beckwith, Citation1994). SecDFYajC might support the pmf-and SecA-dependent steps of protein transport (Duong & Wickner, Citation1997a; Tsukazaki et al., Citation2011). However, Archaea lack SecA but have SecDF, thus their SecA-associated role is not clear (Hand et al., Citation2006). As SecDFYajC binds to the YidC insertase, it was proposed to tether YidC to the SecYEG channel (Nouwen & Driessen, Citation2002). However, a recent study showed that SecY and YidC interact even in the absence of SecDF (Sachelaru et al., Citation2013).
YidC
YidC is an essential membrane protein, present in Bacteria, some Archaea, mitochondria (Oxa1) and chloroplasts (Alb3, Alb4) (for review see Dalbey et al., Citation2011; Kudva et al., Citation2013). It acts as a co-insertase/chaperone supporting the integration of membrane proteins via the Sec complex (Beck et al., Citation2001; Nagamori et al., Citation2004). YidC was recently shown to establish extensive contacts to all four TMs of the lateral gate of SecY (Sachelaru et al., Citation2013). Apart from that, YidC can also serve as a Sec-independent insertase for a broad range of inner membrane proteins (Chen et al., Citation2002; Samuelson et al., Citation2001; Welte et al., Citation2012). YidC substrates are mainly hydrophobic without long periplasmic stretches (Welte et al., Citation2012). While targeting of substrates to YidC has been shown to require the SRP pathway (Facey et al., Citation2007; Welte et al., Citation2012), it remains to be investigated whether this is a general rule for targeting. It also remains to be studied how YidC-mediated insertion of membrane proteins occurs in vivo.
PpiD and Skp
The periplasm of Gram-negative bacteria hosts a myriad of chaperones engaged in protein folding and quality control (for review see Merdanovic et al., Citation2011). Two of these periplasmic chaperones, PpiD and Skp (seventeen-kilodalton-protein) are known to act in the immediate vicinity of the SecYEG translocon. PpiD and Skp are periplasmic chaperones that influence the assembly of numerous outer membrane and periplasmic proteins (Chen & Henning, Citation1996; Dartigalongue & Raina, Citation1998; Jarchow et al., Citation2008). Skp was shown to interact with its substrate in the vicinity of the plasma membrane (Schäfer et al., Citation1999) and before the preprotein is fully translocated by the Sec complex (Harms et al., Citation2001). Although this suggests that Skp is in close proximity to the Sec complex, direct evidence for an interaction between the two is lacking. This is different for PpiD, another non-essential and membrane-anchored periplasmic chaperone. Cross-linking data show that PpiD establishes extensive contacts with the lateral gate of SecY (Sachelaru et al., Citation2013). PpiD is thought to mediate the release of the nascent chain from the translocon and it could play a role in the early folding of translocated proteins (Antonoaea et al., Citation2008; Matern et al., Citation2010).
FtsH and Syd
FtsH is an essential zinc-metalloprotease which plays a role in membrane protein quality control in bacteria, mitochondria and chloroplasts. It is proposed to degrade misfolded substrates in an ATP-dependent fashion (Dalbey et al., Citation2012; Ito & Akiyama, Citation2005). FtsH has been shown to degrade the SecY subunit of the translocon when SecE is not present in stoichiometric amounts (Kihara et al., Citation1995). This could be mediated by the small SecY-binding cytosolic protein Syd which might recognize the compromised status of the translocon (Dalal et al., Citation2009). FtsH has also been found in the complex with YidC indicating that the latter might participate in the quality control of transport processes (van Bloois et al., Citation2008).
MPiase
MPiase is a glycolipid composed of diacylglycerol and a glycan chain of three acetylated aminosugars linked through pyrophosphate. MPiase was shown to exhibit chaperone-like activity driving subsequent membrane integration of substrates (Nishiyama et al., Citation2012). A role during protein translocation and a direct interaction with the Sec complex has also been suggested based on the observation that the topology inversion of SecG occurs only when MPiase associates with SecYEG (Moser et al., Citation2013).
The interaction network of eukaryotic Sec complex
The core Sec complexes in eukaryotes have additional subunits like Sec62 and Sec63, which are involved in post-translational protein transport. The intricate interaction network of the Sec61 translocon also includes proteins required for energizing protein transport (BiP) and substrate folding and modification (TRAP, TRAM, OST). A recent study identified also O-mannosyltransferase in complex with the Sec translocon and found that mannosylation can take place during translocation of the substrate protein (Loibl et al., Citation2014). The eukaryotic translocon also establishes transient contacts to protein kinases and protein acetylases since Sec61 is a subject of co-translational and post-translational regulation. Sec61β, ERj1 and Sec63 have been shown to be phosphorylated by protein kinase C and casein kinase 2 (Ampofo et al., Citation2013; Götz et al., Citation2009; Gruss et al., Citation1999). Yeast Sec62 and Sec61β (Sbh1) appear to be co-translationally acetylated by NatA (Soromani et al., Citation2012).
Sec62/Sec63
Sec62 and Sec63 are integral ER membrane proteins facilitating Sec61-dependent translocation. Sec62 has two membrane-spanning helices and a positively charged N-terminal cytoplasmic domain (Wittke et al., Citation2000). Sec63 belongs to the Hsp40 family of heat shock proteins and contains a characteristic J-domain between its second and third TM () (Skowronek et al., Citation1999). The J-domain is located in the ER lumen where it interacts with the Hsp70-family protein BiP (Brodsky et al., Citation1995). The negatively charged C-terminus of Sec63 contacts the N-terminus of Sec62 (Lang et al., Citation2012).
Sec62 is an essential protein involved in posttranslational translocation (Deshaies & Schekman, Citation1989; Lang et al., Citation2012; Ng et al., Citation1996). Sec63, on the other hand, influences both, post-translational and co-translational transport (Brodsky et al., Citation1995; Young, Citation2001). For the latter, Sec63 acts independently of Sec62 (Jermy et al., Citation2006; Mades et al., Citation2012). However, Sec63 is not essential in mammalian cells (Lang et al., Citation2012; Meyer et al., Citation2000; Tyedmers et al., Citation2000), since it could be functionally replaced by a similar protein Erj1 (Kroczynska et al., Citation2004). The mammalian homologue of Sec62 has gained a ribosome-binding site alluding to a possible contribution to co-translational transport (Müller et al., Citation2010).
Sec62/Sec63 complex assembles in a 1:1 ratio with the core translocon (Meyer et al., Citation2000). Yeast has two additional subunits, Sec71 and Sec72 () in complex with Sec62/Sec63 (Deshaies et al., Citation1991; Feldheim et al., Citation1993; Plath et al., Citation2004). Although mutations in sec71 or sec72 impair protein transport, they are not essential and their role is not understood (Fang & Green, Citation1994).
BiP (binding immunoglobulin protein)
BiP, also known as 78 kDa glucose-regulated protein (GRP-78), heat shock 70 kDa protein 5 (HSPA5) or Kar2p in yeast, is an essential lumenal Hsp70-family chaperone. BiP has multiple functions during ER transport; it assists the insertion of pre-proteins into the Sec complex (Dierks et al., Citation1996), helps in gating the Sec complex (Alder et al., Citation2005; Hamman et al., Citation1998) and serves as a molecular ratchet during translocation (Nicchitta & Blobel, Citation1993; Tyedmers et al., Citation2003). Binding of BiP to the Sec complex occurs via its co-chaperone Sec63 (Lyman & Schekman, Citation1995, Citation1997) and Erj1 (Dudek et al., Citation2002). In addition, BiP has multiple lipid binding sites (Keller, Citation2011). Recently, the lumenal loop 7 of Sec61α was shown to contact BiP (Schäuble et al., Citation2012).
Calmodulin
The Sec61 pore is responsible for passive Ca2+ efflux from the ER into the cytoplasm (Erdmann et al., Citation2011; Flourakis et al., Citation2006) and preventing Ca2+ leakage requires channel gating. The chaperone BiP has been shown to seal the lumenal opening of the Sec61α during early translocation events (Alder et al., Citation2005; Hamman et al., Citation1998). In higher eukaryotes, Ca2+ leakage is further reduced by the cytoplasmic protein calmodulin. Calmodulin is a universal mediator of Ca2+-controlled activity of numerous enzymes, ion channels, aquaporins and other proteins (Zhou et al., Citation2013). Recently, a high affinity binding site for calmodulin (IQ motif) was identified on the cytosolic N-terminus of Sec61α (Erdmann et al., Citation2011). Ca2+-bearing calmodulin binds to the translocon and limits ion flux (Erdmann et al., Citation2011) but the efficiency of calmodulin in restricting Ca2+ leakage largely depends on the presence of BiP (Schäuble et al., Citation2012). Calmodulin might also have an additional role in the post-translational targeting pathway (Shao & Hegde, Citation2011).
TRAM (translocating chain associated membrane protein)
TRAM is a 37-kDa glycoprotein that spans the ER membrane eight times with both N- and C-termini facing the cytoplasm (Tamborero et al., Citation2011). TRAM was identified as a major cross-linking partner of several secretory proteins in mammalian cells (Görlich et al., Citation1992; Krieg et al., Citation1989) and was shown to be required for protein transport in reconstituted proteoliposomes (Gorlich & Rapoport, Citation1993). Crosslinking data demonstrate that TRAM remains in contact with nascent chains even after their release from Sec61α (Liao et al., Citation1997; Sadlish et al., Citation2005). TRAM is suggested to act as a chaperone during the integration of less hydrophobic TM segments into the bilayer (Cross & High, Citation2009; Heinrich et al., Citation2000; Shao & Hegde, Citation2011). It is likely that TRAM cooperates with the Sec complex to assemble multiple TMs, a function similar to the proposed role of YidC during bacterial membrane insertion.
TRAP (translocon associated protein complex)
TRAP is a hetero-tetrameric protein complex that binds stoichiometrically to Sec61 (Hartmann et al., Citation1993; Ménétret et al., Citation2008). TRAP associates with Sec61 and oligosaccharyl transferase (OST, see below) to form the most abundant protein complexes associated with membrane-bound ribosomes (Potter & Nicchitta, Citation2002). In situ cryo electron tomography studies have mapped TRAP in complex with monomeric Sec61 (Pfeffer et al., Citation2012). TRAP accelerates transport of various substrates, but its precise function is unknown (Fons et al., Citation2003). Recent studies have suggested that TRAP is involved in the topogenesis of membrane proteins, affecting the translocation of charged residues (Sommer et al., Citation2013).
OST (oligosaccharyl transferase)
Approximately 70% of the eukaryotic secretome is potentially glycosylated (Zafar et al., Citation2011). Errors in glycosylation lead to misfolded proteins, giving rise to many congenital diseases (Schachter & Freeze, Citation2009). Asparagine-linked (N-linked) glycosylation takes place in the lumen of the ER and is catalyzed by OST, which transfers the dolichol-linked sugar unit to the corresponding sequon in the substrate protein (Tai & Imperiali, Citation2001). OST is a membrane-embedded hetero-oligomeric complex with at least eight different subunits in yeast (Karaoglu et al., Citation1997). The catalytic subunit STT3 is highly conserved across the species (Burda & Aebi, Citation1999). N-glycosylation can occur co-translationally in a supramolecular complex of OST, the translating ribosome and Sec61 (Harada et al., Citation2009). OST binds to the ribosome near the tunnel exit (Harada et al., Citation2009) and to the Sec61 complex (Chavan et al., Citation2005; Pfeffer et al., 2014; Wang & Dobberstein, Citation1999). Shibatani et al. (Citation2005) showed that mammalian OST complexes also have a high affinity to TRAM.
N-linked glycosylation is one of the most common post-translational modifications in eukaryotes, but is also conserved in several prokaryotes (Aebi et al., Citation2013; Baker et al., Citation2013). The catalytic subunit of the bacterial OST is called PglB and exhibits significant sequence similarity to the eukaryotic STT3 (Schwarz & Aebi, Citation2011), however a direct interaction of PglB with the bacterial SecYEG complex remains to be demonstrated.
Calnexin
Calnexin is a type I ER membrane protein that serves as a constituent of the ER chaperone machinery for glycoproteins (Aebi et al., Citation2010). Calnexin can bind to its substrates both post-translationally and co-translationally, suggesting its proximity to the Sec61 translocon (Chen et al., Citation1995). A direct interaction between calnexin and the Sec61 complex was confirmed by two-hybrid analyses and co-immuneprecipitation (Boisramé et al., Citation2002). Recently, it was shown that palmitoylated calnexin is part of the ribosome-translocon complex and makes contact to the Sec61 associated TRAPα subunit (Lakkaraju et al., Citation2012a). Interestingly, the calnexin-ribosome-translocon complex appears to require the actin cytoskeleton for stabilization, which adds to the emerging concept that the cytoskeleton serves as an organizer and regulator of multiple cellular processes (Jaqaman & Grinstein, Citation2012; Kim & Coulombe, Citation2010).
RAMP4 (ribosome-associated membrane protein)
RAMP4 is a 7-kDa single-spanning membrane protein associating with the active ribosome-Sec61 complex (Görlich et al., Citation1992). In cells with high secretory activity like hepatocytes, the unfolded protein response is induced in the absence of RAMP4 (Hori et al., Citation2006). Overexpression of RAMP4 in ER-stressed HEK293 cells supresses aggregation and degradation of newly synthesized membrane proteins (Yamaguchi et al., Citation1999). This indicates that RAMP4 is involved in membrane protein folding. RAMP4 has also been shown to regulate N-linked glycosylation of nascent secretory proteins (Lee et al., Citation2003; Schröder et al., Citation1999). Moreover, RAMP4 could be involved in the early sensing of a nascent chain since the eukaryotic ribosomal protein Rpl17 (E. coli L22 homologue) crosslinks to RAMP4 only if the nascent TM segment is buried inside the ribosomal tunnel (Pool, Citation2009).
Erj1
ERj1 is a Sec63-related mammalian ER-membrane resident protein belonging to the Hsp40 family (Dudek et al., Citation2002). Erj1 contacts the ribosomal tunnel exit and BiP in the periplasm (Blau et al., Citation2005; Dudek et al., Citation2005), regulating protein translation in a BiP-dependent manner (Benedix et al., Citation2010; Dudek et al., Citation2005).
Protein targeting to the Sec complex
Signal sequences
Signal peptides determine the cellular localization of proteins (Hegde & Bernstein, Citation2006). Proteins that are translocated via the Sec complex usually possess an N-terminal signal sequence, which has three parts: a positively charged N-terminal region, followed by a central hydrophobic H-region and a polar C-terminal region (, (von Heijne, Citation1985). In secretory proteins, the C-region contains a cleavage site for signal peptidases (von Heijne, Citation1984). The general architecture of signal sequences is conserved, but they are highly variable in their primary sequence and length (von Heijne, Citation1986). Eukaryotic and prokaryotic signal sequences are interchangeable (von Heijne, Citation1985). Several studies, however, have shown that the signal peptide is not the sole determinant for efficient targeting to the Sec translocon. Mature alkaline phosphatase C-terminal portion contributes to the SecA and translocon binding independently of the signal peptide (Gouridis et al., Citation2009). Furthermore, the superoxide dismutase in proteobacteria is transported to the periplasm despite of the lack of a canonical signal peptide (Krehenbrink et al., Citation2011).
Figure 7. The signal sequence. The signal peptides of the Sec translocon susbtrates in eukaryotes and prokaryotes share a common architecture, with a short, positively charged N-terminal region (N-region), a central, hydrophobic region (H-region) and a polar C-terminal region (C-region). The best conserved part of the signal peptide is the C-region, which can also contain a signal peptidase (SPase) cleavage site. This Figure is reproduced in color in the online version of Molecular Membrane Biology.

Most integral membrane proteins lack cleavable signal sequences and their highly hydrophobic N-terminal TM serves as a signal for recognition instead (von Heijne, Citation1990). This TM is a stretch of about 20 mostly non-polar amino acids and is referred to as a signal anchor sequence.
Co-translational targeting via the SRP pathway
In 1970, Blobel and Sabatini proposed that the cellular localization of a protein is dictated by a region encoded in its N-terminus. SRP was discovered nine years later as a cytosolic agent restoring the translocation activity of high-salt washed canine pancreatic ER microsomes (Walter & Blobel, Citation1980; Walter et al., Citation1981). SRP was also shown to selectively arrest the translation of a secretory pre-protein in vitro (Walter & Blobel, Citation1981). This translation elongation arrest was rescued by an ER membrane-embedded factor (Walter & Blobel, Citation1981), which was identified to be the SRP receptor (SR) (Gilmore et al., Citation1982a, Citation1982b). Soon after, SRP was found to bind to the N-terminus of its substrate (Kurzchalia et al., Citation1986). The SRP pathway is highly conserved and essential in all organisms (Bernstein et al., Citation1993; Larsen & Zwieb, Citation1993; Poritz et al., Citation1988a, Citation1988b; Römisch et al., Citation1990), with the known exception of the yeast S. cerevisiae (Hann & Walter, Citation1991) and some Streptococcus species (Gutierrez et al., Citation1999; Hasona et al., Citation2005).
Initial attempts to link prokaryotic SRP to protein targeting events were unsuccessful and it was assumed that SRP in bacteria might function as a chaperone. This assumption was supported by the fact that the SRP components were not identified in the initial genetic screens for mutants impaired in protein secretion. It became clear only later, that the E. coli SRP pathway is predominantly engaged in targeting of membrane proteins (de Gier et al., Citation1996; Luirink et al., Citation1994; Macfarlane & Muller, Citation1995) which explains why the SRP pathway was not identified in the initial genetic screens using secretory proteins as substrates. Subsequent biochemical studies revealed that the E. coli SecA/SecB pathway and the SRP pathway constitute two largely non-overlapping targeting pathways for secretory and membrane proteins, respectively (Koch et al., Citation1999; Valent et al., Citation1998). Re-defined genetic screens then also confirmed the requirement of the SRP pathway for membrane protein targeting (Tian & Beckwith, Citation2002).
Structure of the SRP
The structure of SRP varies largely across species. Eukaryotic SRP is composed of six proteins (SRP9/SRP14/SRP19/SRP54/SRP68/SRP72) and the 7S RNA (Figure 4A; Walter & Blobel, Citation1982). Bacteria have a minimal version of SRP, consisting of the protein Ffh, (Fifty-Four Homologue, due to its homology to the eukaryotic SRP54 subunit), and 4.5S RNA (Figure 4B; Poritz et al., Citation1990) both being essential for targeting. Ffh can functionally replace mammalian and yeast SRP54 (Bernstein et al., Citation1993; Powers & Walter, Citation1997). Hence, the bacterial SRP represents the minimal functional SRP.
Ffh can be divided into two domains: The methionine-rich M-domain is responsible for signal sequence binding (Lütcke et al., Citation1992; Zheng & Gierasch, Citation1997; Zopf et al., Citation1990), while the N-terminal NG-domain contains the GTPase centre and provides the binding surface for the SRP receptor (Egea et al., Citation2004; Focia et al., Citation2004; Zopf et al., Citation1993). The high methionine content in the M-domain is thought to provide structural flexibility for accommodating signal sequences of different sizes and compositions (Bernstein et al., Citation1993; Keenan et al., Citation1998). The 4.5S RNA interacts with both domains of Ffh. It constitutes together with the M-domain a binding groove for signal sequences, and it is required for stable complex formation between Ffh and SR by modulating their GTP hydrolysis activity during the targeting cycle (Ataide et al., Citation2011; Jagath et al., Citation2001; Miller et al., Citation1994; Zheng & Gierasch, Citation1997). The presence of RNA in SRP probably alludes to its evolutionary age (Hartman & Smith, Citation2010). Interestingly, SRP RNA is lacking in the plastids of spermatophytes, where it has been functionally replaced by the protein cpSRP43 (Träger et al., Citation2012). In other plants, cpSRP43 and RNA subunits are both required for functionality of the SRP complex (Träger et al., Citation2012).
Our knowledge on prokaryotic SRP is mainly based on studies using E. coli as a model organism. However, additional SRP subunits or SRP-like GTPases have been found in other bacteria and Archaea (Bange & Sinning, Citation2013; Zwieb & Bhuiyan, Citation2010).
The most significant difference between the bacterial and the eukaryotic SRP is the presence of the Alu domain in the latter. The Alu domain consists of the SRP subunits 9 and 14 and domain I of 7.5S RNA (Siegel & Walter, Citation1985; Siegel & Walter, Citation1986). It has been shown to arrest translation elongation immediately after the signal sequence emerges from the ribosome (Ogg & Walter, Citation1995). SRP9 and SRP14 interact with the ribosome at the interface of the small and large ribosomal subunits (Terzi et al., Citation2004). Cryo-EM studies indicate that the Alu domain reaches into the elongation factor binding site to compete with EF-Tu binding (Halic et al., Citation2004). Elongation arrest likely increases the time window for efficient targeting to avoid that substrates exceeding a critical length lose their translocation competence (Flanagan et al., Citation2003). Elongation arrest may depend on the concentration of free SR on the ER membrane (Lakkaraju et al., Citation2008). E. coli SRP does not contain the Alu domain and therefore lacks the ability to arrest translation (Powers & Walter, Citation1997).
The SRP19 subunit of eukaryotic SRP mediates 7S RNA binding to SRP54 (Egea et al., Citation2008; Hainzl et al., Citation2002; Citation2005; Wild et al., Citation2001). The SRP68/72 subunits probably cooperate with SRP19 to support the last step, SRP54 binding, of SRP complex assembly (Leung & Brown, Citation2010).
The SRP cycle
The SRP cycle () starts with substrate recognition at the tunnel exit of the ribosome. SRP and ribosomes establish a high-affinity contact before the substantial part of the nascent chain has emerged from the tunnel (Bornemann et al., Citation2008; Flanagan et al., Citation2003). E. coli SRP rapidly scans ribosomes and its binding to the ribosomal tunnel exit is only stabilized in the presence of a signal anchor sequence (Holtkamp et al., Citation2012). After the M-domain of SRP binds the signal sequence, the complex is targeted to the membrane where contacts between SRP and SR are established. The NG-domains of SRα/FtsY and SRP build a pseudo-homodimer () (Egea et al., Citation2004; Focia et al., Citation2004) forming a composite GTPase site. GTP binding stablilizes the interaction between SRP and SR (Bacher et al., Citation1996) and initiates the transfer of signal sequence from SRP to the Sec complex (Rapiejko & Gilmore, Citation1997). The Sec translocon regulates conformational changes in the compound SRP-SR complex and activates GTP hydrolysis (Akopian et al., Citation2013a). SRP and FtsY stimulate GTP hydrolysis reciprocally (Powers & Walter, Citation1997). Released SRP then cycles back to the cytosol to start a new targeting reaction. For recent reviews on the SRP cycle see Leung & Brown (Citation2010), Akopian et al. (Citation2013b), Kudva et al. (Citation2013), Nyathi et al. (Citation2013), and Kuhn et al. (Citation2014).
Figure 8. Schematic view of the SRP-SR cycle. The model was adapted from (Akopian et al., Citation2013b). (1) SRP rapidly scans ribosomes and binds stably only to those translating a SRP substrate (2) The presence of the correct substrate increases the affinity of SRP to its receptor (SR), resulting in targeting of the ribosome nascent chain (RNC) to the membrane. This conformation of the SRP-SR complex is termed the early complex. In bacteria, the SRP-SR affinity is further increased if SR is in contact with negatively charged phospholipids. (3) In the presence of the correct substrate, the GTPase domains of SRP and SR align to form a composite GTPase centre (closed complex). To prevent premature dissociation of the SRP-SR complex, GTP hydrolysis is delayed until contacts to the Sec translocon are established. (4) GTP hydrolysis induces the dissociation of SRP-SR complex. SRP-SR GTPases do not require GTP-activating proteins or nucleotide-exchange factors. For SRP, the contact with the ribosome might be sufficient to exchange GDP against GTP. This Figure is reproduced in color in the online version of Molecular Membrane Biology.
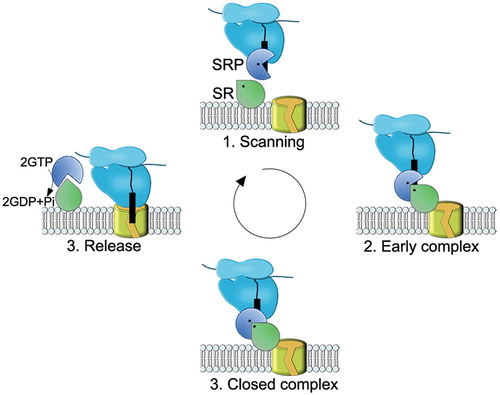
Eukaryotic SRP recognizes both secretory pre-proteins and membrane proteins, while E. coli SRP has a higher affinity for hydrophobic signal anchor sequences (Beck et al., Citation2000; Lee & Bernstein, Citation2001; Valent et al., Citation1995). Yet E. coli SRP is also required for the targeting of a few periplasmic proteins, with cleavable but unusually hydrophobic signal sequences (Huber et al., Citation2005). This probably explains why E. coli SRP was also found to bind to the signal sequence of the eukaryotic secretory protein preprolactin (Luirink et al., Citation1992) and why E. coli Ffh can replace SRP54 in eukaryotes (Bernstein et al., Citation1993; Powers & Walter, Citation1997). How SRP selects its substrates is still not entirely clear, but it is thought to be mainly influenced by the hydrophobicity of the substrate protein (Beck et al., Citation2000; Neumann-Haefelin et al., Citation2000; Ng et al., Citation1996; Valent et al., Citation1998) and by the absence of helix breaking amino acids (Beha et al., Citation2003). Additionally, translational speed and specific proofreading steps which control the GTPase cycle of the SRP-SR targeting complex probably contribute to substrate selection (Zhang et al., Citation2009a, Citation2010).
SRP-independent targeting to the Sec complex
In vitro studies performed with E. coli inverted membrane vesicles have determined that SecA, SecB and the pmf are necessary and sufficient for the translocation of Sec-dependent secretory proteins, i.e. proteins of the periplasmic space or the outer membrane (Brundage et al., Citation1990; Cunningham et al., Citation1989; Koch et al., Citation1999). SecA is only found in Bacteria and SecB in Proteobacteria (Müller et al., Citation2001). How Archaea have compensated for the loss of SecA is not known, but in some species the Sec-independent post-translational Tat (twin-arginine translocation) pathway might be responsible for the export of many secretory proteins (Palmer & Berks, Citation2012).
Also eukaryotic cells employ SRP-independent pathways for secretory and membrane proteins. These alternative pathways initially seemed to be required in S. cerevisiae where the SRP-pathway is inessential (Hann & Walter, Citation1991). However, it is becoming increasingly apparent that post-translational transport across the ER membrane is utilized by a significant number of proteins in all eukaryotes. One SRP-independent pathway in eukaryotes is mediated by the ER-embedded Sec62/Sec63 proteins, which cooperate with Sec61. Another known pathway is the Get3/TRC40 pathway, which transports membrane proteins with C-terminal transmembrane domains (tail-anchored [TA] proteins). Although, SRP was found to interact with model TA proteins (Abell et al., Citation2004; Leznicki et al., Citation2010), it is generally assumed that the GET3/TRC40 pathway does not involve the Sec complex (Denic et al., Citation2013; Hegde & Keenan, Citation2011).
Bacterial SecA/SecB pathway
Secretory pre-proteins in E. coli are targeted to the translocon by the 100 kDa-ATPase SecA, which in some cases is assisted by the cytosolic chaperone SecB () (Driessen & Nouwen, Citation2008; Kudva et al., Citation2013; Park & Rapoport, Citation2012). SecA binds to signal sequences (Akita et al., Citation1990; Karamyshev & Johnson, Citation2005), and is therefore required for the targeting of pre-proteins to the membrane (Gelis et al., Citation2007; Lill et al., Citation1990; Randall & Hardy, Citation1977). However, it is not clear where the signal sequence binding occurs. The classical model suggests that it takes place after the pre-protein is targeted to the membrane by SecB and other chaperones (Fekkes et al., Citation1998; Randall et al., Citation1998). Recent evidence for SecA-ribosome binding indicates however that SecA may also recognize its substrates co-translationally (Huber et al., Citation2011).
SecB is a 17 kDa cytosolic protein that forms a homotetramer (Xu et al., Citation2000) and is thought to hold the secretory pre-protein in an unfolded state (Randall et al., Citation1998; Watanabe & Blobel, Citation1989). Its role in pre-protein targeting is disputed since SecB does not bind the signal sequence specifically, but rather has a general affinity to hydrophobic stretches (Knoblauch et al., Citation1999). Furthermore, SecB is not essential suggesting that protein transport is not strictly dependent on it (de Cook & Tommassen, Citation1991; van der Sluis & Driessen, Citation2006). The number of E. coli proteins for which a clear SecB dependency during transport has been shown is rather low (<20), suggesting that it is dispensable during transport of most E. coli proteins.
In vitro studies have identified another chaperone, Trigger Factor (TF), as one of earliest and major contact partners of a nascent secretory protein (Beck et al., Citation2000; Deuerling et al., Citation2003; Valent et al., Citation1995). However, recent in vivo evidence from ribosome profiling studies suggests that TF mainly binds to substrates after they have reached a length of more than 100 residues (Oh et al., Citation2011). TF preferentially associates with ribosomes engaged in translation of secretory proteins (Oh et al., Citation2011). Ribosome-binding is required for the activity of TF which is to keep the nascent polypeptide in a translocation-competent loosely folded shape (Hoffmann et al., Citation2012). We suggest a recent review by Castanié-Cornet et al. (Citation2013) for more details on the chaperone network facilitating protein targeting in E. coli.
Eukaryotic Sec62/Sec63 pathway
Yeast cells lacking SRP suffer from severe growth defects (Hann & Walter, Citation1991), but their translocation ability recovers over time (Ogg et al., Citation1992). This indicates the existence of alternative SRP-independent protein targeting pathways. Attempts to identify these salvage pathways soon led to the discovery of SEC62/SEC63 alleles since their mutations caused secretion defects of SRP-independent pre-proteins (Deshaies & Schekman, Citation1989; Ng et al., Citation1996). In fact, a large-scale analysis of yeast S. cerevisiae signal sequences predicts that approx. 43% of its secretome would utilize an SRP-independent pathway (Ast et al., Citation2013). It is important to emphasize that post-translational transport still requires the Sec61 channel and BiP (Matlack et al., Citation1999; Panzner et al., Citation1995).
Although Sec62 has been mainly associated with post-translational translocation, Sec63 might also contribute to co-translational transport (Deshaies & Schekman, Citation1989; Ng et al., Citation1996). Nevertheless, the precise function of both proteins remains elusive. Sec63 is thought to affect the gating of the Sec61 translocon in a precursor dependent fashion (Lang et al., Citation2012). Furthermore, overexpression of Sec63 leads to a considerable decrease in the steady-state levels of multi-spanning membrane proteins (Mades et al., Citation2012), indicating that Sec63 might also have a regulatory function.
The C-terminus of yeast Sec62 was found in close proximity to the signal sequence of prepro-alpha-factor in vivo (Dünnwald et al., Citation1999). This was confirmed by cross-linking experiments, which indicate that the signal sequence establishes contacts with both Sec61 and Sec62 (Plath et al., Citation2004). Further systematic studies of substrates with different signal sequences indicate that Sec62 is indeed involved in recognition of moderately hydrophobic signal sequences (Reithinger et al., Citation2013).
The transport of two discrete sets of proteins has been shown to be dependent on Sec62: the translocation of small proteins (100–160 residues) (Lakkaraju et al., Citation2012b) and the transport of GPI-anchored cell surface proteins (Ast et al., Citation2013). Small proteins, including hormones, chemokines, and antimicrobial peptides are abundant in metazoa (Ingolia et al., Citation2009). Why small proteins escape SRP pathway is not clear but they may simply be too short for efficient co-translational recognition. GPI-anchored proteins carry an N-terminal signal sequence and a characteristic C-terminal GPI signature sequence, which is potentially recognized by the Get3/TRC40 pathway (Ast et al., Citation2013). Both motifs are required for efficient translocation, revealing the interplay between two different targeting systems (Ast et al., Citation2013).
It is not entirely clear how substrates are routed to the post-translational targeting pathway in eukaryotes. Some, like prepro-alpha factor appear to engage both SRP-dependent and -independent targeting pathways in yeast, but are exclusively co-translationally targeted by SRP when analyzed with mammalian ER membranes (Garcia & Walter, Citation1988; Ng et al., Citation1996). Pathway selection is not only determined by the signal sequence or the length of a preprotein, but additional features within the mature domain are also important (Johnson et al., Citation2013; Shao & Hegde, Citation2011). A recent study also suggests that SRP and Sec62-mediated targeting might not be mutually exclusive (Reithinger et al., Citation2013).
What are the cytosolic targeting factors for Sec62/Sec63 pathway remains to be elucidated. The ATP-dependent Hsp70/Hsp90 chaperone network has been shown to bind post-translationally transported proteins (Ast et al., Citation2013; Chirico et al., Citation1988; Deshaies et al., Citation1988). Surprisingly, the Ca2+-binding protein calmodulin binds selectively to the hydrophobic signal sequences of short precursors in a Ca2+-dependent fashion (Shao & Hegde, Citation2011). Like SRP, calmodulin contains a methionine-rich domain responsible for recognizing a diverse set of hydrophobic targets (O’Neil & DeGrado, Citation1990). Calmodulin maintains the translocation competence of the small proteins protecting them from ubiquitination (Shao & Hegde, Citation2011). How calmodulin releases its substrate is not known, but it was shown to interact with Sec61 (Erdmann et al., Citation2011). The frequency and significance of calmodulin-mediated protein targeting needs to be further studied.
Translation-independent targeting
Asymmetric mRNA localization is a tool to determine the location of protein synthesis in eukaryotes. Developmental abnormalities and neuronal disorders are caused by mRNA trafficking defects (Xing & Bassell, Citation2013). mRNA transport is a common paradigm in polarized cells but recent evidence indicates that ER localization of proteins might also be determined by ribosome-free mRNA targeting (Hermesh & Jansen, Citation2013). The ER acts as a major site for protein synthesis in eukaryotes (Reid & Nicchitta, Citation2012; Stephens et al., Citation2005). The translation of cytosolic and nuclear mRNAs can be initiated by ER-bound ribosomes but the lack of a signal sequence induces ribosome detachment from the ER membrane (Potter & Nicchitta, Citation2000). It has also been shown that the large ribosomal subunit remains at the membrane after synthesis of a secretory or a membrane protein is terminated (Potter & Nicchitta, Citation2000). This probably reduces the need for SRP-dependent targeting of RNCs. Targeting of mRNAs to the ER membrane in S. cerevisiae occurs independently of translation and SRP (Kraut-Cohen et al., Citation2013). Recent studies have revealed that prokaryotic mRNAs also tend to localize at the place where their corresponding protein products execute their function (Broude, Citation2011; Montero Llopis et al., Citation2010; Nevo-Dinur et al., Citation2011; Valencia-Burton et al., Citation2007).
What promotes ribosome-independent mRNA localization to the ER or the bacterial inner membrane is not known. Both the involvement of the cytoskeleton (Czaplinski & Singer, Citation2006; Palacios, Citation2007) and mRNA-binding proteins (to facilitate diffusion) have been suggested (Hermesh & Jansen, Citation2013). It is also not clear on what are the targeting decisions based. It is proposed that cis-acting sequences within mRNAs may serve as targeting signals. Membrane-spanning domains are encoded by the codons showing a strong uracil bias (Prilusky & Bibi, Citation2009). Indeed, the U-enriched stretches of the transcript are required to localize mRNA properly (Bibi, Citation2012; Kraut-Cohen & Gerst, Citation2010).
The Sec61 complex is probably the main ribosome docking site in eukaryotes, because after Sec61 depletion 60% less ER-bound polysomes have been observed (Lang et al., Citation2012). The relative amount of membrane-bound ribosomes is thought to be much lower in bacteria (Herskovits & Bibi, Citation2000; Randall & Hardy, Citation1977) and the classical model suggests that ribosomes detach from the membrane after synthesis and insertion of membrane proteins is completed. However, even non-translating ribosomes exhibit a significant affinity for the Sec translocon in E. coli (Prinz et al., Citation2000; Schaletzky & Rapoport, Citation2006). Membrane-bound ribosomes could re-initiate the translation of SRP substrates and thus would not depend on cytosolic SRP for targeting. In support of this, a recent study has shown that membrane-anchored SRP maintains its targeting activity (Braig et al., Citation2011), indicating that recognition of signal sequences does not necessarily need to occur in the cytosol. Furthermore, it was suggested that FtsY, the bacterial SRP receptor, is co-translationally targeted to the bacterial membrane by an SRP-independent mechanism (Herskovits et al., Citation2002). This would constantly replenish the membrane-bound ribosome pool (Bibi, Citation2011). However, this model requires further experimental verification.
Mechanisms of SecY/Sec61 mediated co-translational and post-translational protein transport
The Sec complex constitutes a passive pore that needs to be activated by an interaction with its partner proteins (). Although the function of some of these proteins is known, in most cases it is still unclear how the biological function of these proteins is coordinated with the transport activity of the Sec complex.
A single copy of SecY/Sec61 is sufficient for protein transport
The oligomeric state of the Sec complex in its resting and active state has been a matter of intense debate. Depending on experimental procedures and the expression level of the Sec complex, monomeric, dimeric, tetrameric and higher oligomers of SecY and Sec61 complexes have been found (see Park & Rapoport, Citation2012; Kudva et al., Citation2013 for recent reviews). Although there appears to be now a general agreement that a single copy of the Sec complex is sufficient for protein translocation and insertion, pore sizes not compatible with a single SecY copy were detected by fluorescence quenching experiments (Hamman et al., Citation1997) and electrophysiology measurements (Wirth et al., Citation2003). Thus, issues about the oligomeric state of the Sec complex and in particular the influence of substrates or Sec accessory proteins need to be resolved.
The active Sec complex during post-translational protein translocation
Translocation of a secretory protein through the Sec complex commences with loop-like insertion of the substrate into the channel. The signal sequence remains in contact with the channel wall, while the downstream segment resides in the pore (Plath et al., Citation1998; Shaw et al., Citation1988). Substrate insertion requires an open channel, and in the bacterial Sec complex, this channel opening might be induced upon binding of SecA (Zimmer et al., Citation2008). The crystal structure of the SecA-bound Sec complex shows a partially opened lateral gate and a displaced plug domain, although the latter appears to still seal the channel (Zimmer et al., Citation2008). Signal sequences have been cross-linked to both TMs 2b and 7 of Sec61 channel (Plath et al., Citation1998) and to phospholipids (Martoglio et al., Citation1995). This probably indicates that after initial SecA-dependent weakening of the lateral gate it further opens by the intercalation of the signal sequence. This is supported by the data with 2D crystals of the SecYEG complex in contact with a preprotein mimic (Hizlan et al., Citation2012). These data show that TM2b is tilted away from the lateral gate and that TM7 is relocated closer to TM5 and TM10 at the back of the channel. This movement displaces the plug even further and brings it closer to TM3 of SecE. The movement of TM7 as observed in the pre-protein activated 2D (Hizlan et al., Citation2012) structure has not been observed in the SecA-activated SecYE structure (Zimmer et al., Citation2008), and therefore both structures probably represent consecutive steps of the activation of the Sec complex prior to translocation. Recent cryo-EM reconstitution indicates that the plug does not shift prominently in the translocating Sec61-ribosome complex; rather the TM10 moves outward, disrupting the central constriction pore (Gogala et al., Citation2014). The observed conformational changes explain the phenotype of several prl mutations, which allow the translocation of substrates with defective or missing signal sequences. Many of the prl mutations were mapped to TM2a (plug), TM7 and TM10 (Osborne & Silhavy, Citation1993; Smith et al., Citation2005). In these mutants, lateral gate opening and plug movement is probably largely signal sequence independent.
Substrates are translocated through the channel in discrete steps of approx. 30–40 amino acids (Schiebel et al., Citation1991; Tomkiewicz et al., Citation2006) and each step is linked to ATP hydrolysis by SecA. How ATP binding and hydrolysis drive translocation of the substrate across the channel, is still unknown. One model predicts that two short α-helices of SecA, the two-helix finger, inserts into the channel upon ATP binding. After ATP hydrolysis, the two helix finger would reset and insert again upon binding the next ATP, thereby providing the mechanical force for the step-wise translocation (Erlandson et al., Citation2008). Although highly attractive, this model is questioned by the observation that translocation proceeds even when the two-helix finger is trapped in place by chemical cross-linking (Whitehouse et al., Citation2012). Alternative models have been proposed (Tomkiewicz et al., Citation2007), but none of them is currently fully supported by the experimental data.
Post-translational transport across the bacterial SecYEG complex is also stimulated by the proton motive force (pmf) as first described by (Driessen & Wickner, Citation1991). Their study also showed that both components of the pmf, i.e. the electrochemical gradient (Δψ) and proton gradient (ΔpH) are required for stimulation. Pmf reduces the amount of ATP required for the in vitro translocation of secretory proteins (Shiozuka et al., Citation1990) and is thought to act at a late stage of translocation (Schiebel et al., Citation1991). On the other hand, pmf was also proposed to help orienting the signal sequence within the Sec channel, which occurs at an early phase of translocation (Nouwen et al., Citation1996). Conformational changes triggered by the proton transfer could allow SecDFYajC to pull out substrates from the periplasmic side of the Sec channel (Tsukazaki et al., Citation2011). A similar function was also proposed for periplasmic chaperones like Skp (Schäfer et al., Citation1999) or PpiD (Antonoaea et al., Citation2008). However, it is unknown whether this function of Skp or PpiD is linked to the pmf.
In eukaryotes, the pre-proteins are transported to the translocon with the help of cytosolic chaperones of the Hsp90, Hsp70 and Hsp40 families (Chirico et al., Citation1988; Zimmermann et al., Citation1988). These chaperones keep pre-proteins competent for interaction with the Sec complex. During translocation, the Sec61 core associates with the Sec62/Sec63 complex, which in yeast involves also Sec71 and Sec72 (reviewed in Zimmermann et al., Citation2011). The cytosolic domains of these membrane proteins might interact with the chaperones, but this needs to be experimentally verified. Post-translational translocation in eukaryotes also requires energy, but unlike bacterial SecA, the Hsp70-type chaperone BiP (called Kar2p in yeast) binds to the incoming substrates in the ER lumen and prevents their back-sliding by ATP hydrolysis. This mechanism is referred to as Brownian ratchet because it confers directionality to the otherwise undirected Brownian motion. The Brownian ratchet model is also proposed as a possible mode of action for for SecA (Tomkiewicz et al., Citation2007).
The binding of the J-domain of Sec63 or ERj1 stimulates the ATP hydrolysis by BiP and induces the closure of its substrate binding pocket thereby trapping the translocated pre-protein (for review, see Park & Rapoport, Citation2012). Once the next segment of the translocated polypeptide emerges from the Sec channel, the next BiP molecule binds. Release of BiP from its substrate requires nucleotide exchange factors like Sil1p or Lhs1p (Tyson & Stirling, Citation2000).
The active Sec complex during co-translational protein transport
After SRP-mediated membrane targeting the ribosome docks onto the cytosolic loops C4 and C5 of SecY/Sec61α and the cytoplasmic helix of SecE/Sec61γ (Becker et al., Citation2009; Cheng et al., Citation2005; Frauenfeld et al., Citation2011). This involves conserved binding sites within the rRNA and universal ribosome adaptor site (Behrens et al., Citation2013; Frauenfeld et al., Citation2011; Prinz et al., Citation2000). Cryo-EM structures do not reveal any significant conformational changes within Sec complex upon ribosome binding (Becker et al., Citation2009; Gogala et al., Citation2014; Park et al., Citation2014), although electrophysiology experiments show that ribosomes induce channel opening of the SecYEG translocon (Knyazev et al., Citation2013). The Cryo-EM structures of an RNC-bound Sec complex on the other hand, display a partially opened lateral gate (Frauenfeld et al., Citation2011; Gogala et al., Citation2014; Park et al., Citation2014), similarly to the partially opened SecA-SecY complex (Zimmer et al., Citation2008). The structure of a SecYE complex form Pyrococcus furiosus was solved with a part of a juxtaposed SecYE molecule inserted into the channel (Egea & Stroud, Citation2010). This second copy is suggested to mimic a polypeptide in transit and in agreement with this assumption; the structure shows a wider opening of the lateral gate. However, the plug was not displaced and was still occupying the central restriction (Egea & Stroud, Citation2010).
A recent study showed that upon binding of RNCs bearing a DsbA signal sequence to the Sec translocon (Park et al., Citation2014), the lateral gate opens and the conversion from the closed to the open structure appears to involve mainly rigid body movements of the N-terminal half of SecY. Additional changes are observed for TM7 and the cytoplasmic part of TM8, which is displaced towards the membrane surface. These movements of SecY are accompanied by movements of SecE and SecG (Park et al., Citation2014). The plug only slightly changes its position, but instead movements of the lateral gate helices generate a cavity adjacent to the plug domain that is confined by the signal sequence intercalated in the open lateral gate.
The portioning of TMs from the Sec channel into the lipid phase is mainly determined by the hydrophobicity of the TM (Hessa et al., Citation2005) and follows the “positive-inside rule” (von Heijne & Gavel, Citation1988). The Sec translocon can actively discriminate between TMs and less hydrophobic stretches (Hessa et al., Citation2005). The hydrophobicity threshold for integration is partly controlled by the central constriction of Sec61 pore (Demirci et al., Citation2013) and this threshold seems to be lower in prokaryotes (Öjemalm et al., Citation2013).
It has been recently shown that the Sec complex exerts a biphasic pulling force on a nascent chain (Ismail et al., Citation2012). It is not entirely clear where the TMs are exactly positioned during each phase, but the data are consistent with a sequential and ordered integration pathway as suggested also by (Houben et al., Citation2005; Sadlish et al., Citation2005). However, this linear membrane integration might not apply for every multi-spanning membrane protein. The translocon offers a flexible environment for membrane protein biogenesis as it can specifically delay the integration of selected TMs (Cross & High, Citation2009; Ismail et al., Citation2008) and reorient the others (Goder et al., Citation1999). The insertion of less hydrophobic TMs can be assisted by the neighboring more hydrophobic TM (Heinrich & Rapoport, Citation2003; Öjemalm et al., Citation2012). En bloc insertion has been shown by FRET analyses to occur pairwise (Hou et al., Citation2012) and several TMs can be maintained by the translocon simultaneously (Enquist et al., Citation2009; Sadlish et al., Citation2005).
It is not yet clear to which extent translocon-associated proteins affect the final topology of membrane proteins. Although SecYEG proteoliposomes together with SRP and FtsY are sufficient for membrane protein insertion in vitro (Braig et al., Citation2009; Welte et al., Citation2012), several studies have shown that TMs exiting the Sec complex contact YidC (Beck et al., Citation2001; Urbanus et al., Citation2001). The lateral gate of SecY itself also establishes contacts to the YidC (Sachelaru et al., Citation2013). Intriguingly, in vitro experiments demonstrated that the SecY-YidC interaction is influenced by the presence of RNCs (Sachelaru et al., Citation2013). It appears likely that that the substrate-induced movements of the lateral gate (Park et al., Citation2014) directly affect the SecY-YidC interface. In eukaryotes, TRAM and TRAP are shown to facilitate membrane protein insertion (Hegde et al., Citation1998; Saurí et al., Citation2007).
To support the biogenesis of membrane proteins with large periplasmic portions, the Sec-translocon must operate in a composite insertion-translocation mode. SecA is required to support the translocation of periplasmic domains longer than 30 amino acids (Deitermann et al., Citation2005). As the ribosome and SecA have overlapping binding sites on SecYEG (Kuhn et al, Citation2011) and as they compete with each other for SecYEG binding (Wu et al., Citation2012), it remains open how the simultaneous activity of ribosome and SecA is coordinated. The corresponding process in eukaryotes could be mediated by BiP, although this needs to be verified experimentally.
Conclusion and perspectives
The biochemical, biophysical and structural characterization of the Sec-dependent transport over the last decades has provided a detailed picture of this highly conserved and essential cellular process. A key conclusion is that protein transport machineries are modular and vastly dynamic in nature. This is exemplified by the observation that the Sec translocon cooperates with the seemingly independent twin-arginine translocation machinery during the insertion of a membrane protein (Keller et al., Citation2012). Revealing the coordinated interaction of the Sec translocon with its multiple partner proteins in response to substrate, will be an important next step in understanding the molecular mechanism of protein transport.
There are probably yet undiscovered cytosolic and membrane-bound partner proteins that support efficient transport via the Sec complexes in living cells. For instance, quality control is an important aspect in biological processes, but little is known on how the Sec translocon cooperates with proteases and chaperones for facilitating proper folding and eventually the removal of misfolded or mistargeted proteins.
SecA and SRP have been the subjects of extensive research. However, it needs to be elucidated how SecA links ATP-hydrolysis to polypeptide movement and how the proton motif force and the SecDFYajC complex contribute to translocation. Furthermore, the mechanism of SecA-dependent signal recognition and targeting is not entirely clear. The SecYEG independent insertion of membrane proteins via YidC raises the question of how SRP routes certain substrates into the YidC pathway instead of the SecYEG pathway.
Our knowledge on protein targeting is based on the detailed analyses of only a few substrates. A more comprehensive catalogue of validated substrates would reveal how they are specifically recognized and what determines their post-translational or co-translational targeting mode. In particular, the role of the translation speed in pathway selection requires further analysis. Likewise, the contribution of translation-independent targeting to the Sec complexes is a largely unexplored and exciting area of research.
Although a couple of high-resolution structures have illuminated intricate details of protein transport, additional structures are needed, i.e. structures of the Sec complex translocating a polypeptide, structures of the eukaryotic Sec complex and structures of the Sec translocon associated with FtsY, YidC, TRAM to name only a few. Cryo Electron tomography and other advanced microscopy techniques promise to provide in-depth analyses of protein transport at the cellular level.
The exciting developments during the last years have allowed a deeper insight into the molecular mechanisms that sustain protein transport via the Sec translocon. Nevertheless, the enormous complexity of this system requires further exploration.
Declaration of interest
The authors report no conflicts of interest. The authors alone are responsible for the content and writing of the paper.
This works was supported by grants of the Deutsche Forschungsgemeinschaft (DFG) (FOR929, FOR967 and GRK1478); and the Excellence Initiative grant of the German Federal and State Governments – GSC-4 Spemann Graduate School of Biology and Medicine to HGK. NAP was supported by a PhD fellowship of the German Academic Exchange Service (DAAD).
References
- Abell BM, Pool MR, Schlenker O, Sinning I, High S. 2004. Signal recognition particle mediates post-translational targeting in eukaryotes. EMBO J 23:2755–2764
- Aebi M, Bernasconi R, Clerc S, Molinari M. 2010. N-glycan structures: Recognition and processing in the ER. Trends Biochem Sci 35:74–82
- Aebi M, Schuldiner M, Schwappach B. 2013. N-linked protein glycosylation in the ER. Biochim Biophys Acta – Mol Cell Res 1833:2430–2437
- Akita M, Sasaki S, Matsuyama S, Mizushima S. 1990. SecA interacts with secretory proteins by recognizing the positive charge at the amino terminus of the signal peptide in Escherichia coli. J Biol Chem 265:8164–8169
- Akopian D, Dalal K, Shen K, Duong F, Shan S-o. 2013a. SecYEG activates GTPases to drive the completion of cotranslational protein targeting. J Cell Biol 200:397–405
- Akopian D, Shen K, Zhang X, Shan S-o. 2013b. Signal recognition particle: An essential protein-targeting machine. Annu Rev Biochem 82:693–721
- Alami M, Dalal K, Lelj-Garolla B, Sligar SG, Duong F. 2007. Nanodiscs unravel the interaction between the SecYEG channel and its cytosolic partner SecA. EMBO J 26:1995–2004
- Albiniak AM, Baglieri J, Robinson C. 2012. Targeting of lumenal proteins across the thylakoid membrane. J Experim Bot 63:1689–1698
- Alder NN, Shen Y, Brodsky JL, Hendershot LM, Johnson AE. 2005. The molecular mechanisms underlying BiP-mediated gating of the Sec61 translocon of the endoplasmic reticulum. J Cell Biol 168:389–399
- Ampofo E, Welker S, Jung M, Müller L, Greiner M, Zimmermann R, Montenarh M. 2013. CK2 phosphorylation of human Sec63 regulates its interaction with Sec62. Biochim Biophys Acta – Gen Subj 1830:2938–2945
- Angelini S, Boy D, Schiltz E, Koch HG. 2006. Membrane binding of the bacterial signal recognition particle receptor involves two distinct binding sites. J Cell Biol 174:715–724
- Angelini S, Deitermann S, Koch HG. 2005. FtsY, the bacterial signal-recognition particle receptor, interacts functionally and physically with the SecYEG translocon. EMBO Rep 6:476–481
- Antonin W, Meyer HA, Hartmann E. 2000. Interactions between Spc2p and other components of the endoplasmic reticulum translocation sites of the yeast Saccharomyces cerevisiae. J Biol Chem 275:34068–34072
- Antonoaea R, Fürst M, Nishiyama K-i, Müller M. 2008. The periplasmic chaperone PpiD interacts with secretory proteins exiting from the SecYEG translocon. Biochemistry 47:5649–5656
- Ast T, Cohen G, Schuldiner M. 2013. A network of cytosolic factors targets SRP-independent proteins to the endoplasmic reticulum. Cell 152:1134–1145
- Ataide SF, Schmitz N, Shen K, Ke A, Shan SO, Doudna JA, Ban N. 2011. The crystal structure of the signal recognition particle in complex with its receptor. Science 331:881–886
- Auclair SM, Bhanu MK, Kendall DA. 2012. Signal peptidase I: Cleaving the way to mature proteins. Protein Sci 21:13–25
- Bacher G, Lutcke H, Jungnickel B, Rapoport TA, Dobberstein B. 1996. Regulation by the ribosome of the GTPase of the signal-recognition particle during protein targeting. Nature 381:248–251
- Bahari L, Parlitz R, Eitan A, Stjepanovic G, Bochkareva ES, Sinning I, Bibi E. 2007. Membrane Targeting of ribosomes and their release require distinct and separable functions of FtsY. J Biol Chem 282:32168–32175
- Baker JL, Celik E, DeLisa MP. 2013. Expanding the glycoengineering toolbox: The rise of bacterial N-linked protein glycosylation. Trends Biotechnol 31:313–323
- Bange G, Sinning I. 2013. SIMIBI twins in protein targeting and localization. Nat Struct Mol Biol 20:776–780
- Baram D, Pyetan E, Sittner A, Auerbach-Nevo T, Bashan A, Yonath A. 2005. Structure of trigger factor binding domain in biologically homologous complex with eubacterial ribosome reveals its chaperone action. Proc Natl Acad Sci USA 102:12017–12022
- Beck K, Eisner G, Trescher D, Dalbey RE, Brunner J, Muller M, Müller M. 2001. YidC, an assembly site for polytopic Escherichia coli membrane proteins located in immediate proximity to the SecYE translocon and lipids. EMBO Rep 2:709–714
- Beck K, Wu L-F, Brunner J, Muller M. 2000. Discrimination between SRP- and SecA/SecB-dependent substrates involves selective recognition of nascent chains by SRP and trigger factor. EMBO J 19:134–143
- Becker T, Bhushan S, Jarasch A, Armache J-P, Funes S, Jossinet F, et al. 2009. Structure of monomeric yeast and mammalian Sec61 complexes interacting with the translating ribosome. Science 326:1369–1373
- Beha D, Deitermann S, Muller M, Koch HG. 2003. Export of beta-lactamase is independent of the signal recognition particle. J Biol Chem 278:22161–22167
- Behrens C, Hartmann E, Kalies KU. 2013. Single rRNA helices bind independently to the protein-conducting channel SecYEG. Traffic 14:274–281
- Benedix J, Lajoie P, Jaiswal H, Burgard C, Greiner M, Zimmermann R, et al. 2010. BiP modulates the affinity of its co-chaperone ERj1 for ribosomes. J Biol Chem 285:36427–36433
- Berndt U, Oellerer S, Zhang Y, Johnson AE, Rospert S. 2009. A signal-anchor sequence stimulates signal recognition particle binding to ribosomes from inside the exit tunnel. Proc Natl Acad Sci USA 106:1398–1403
- Bernstein HD, Zopf D, Freymann DM, Walter P. 1993. Functional substitution of the signal recognition particle 54-kDa subunit by its Escherichia coli homolog. Proc Natl Acad Sci USA 90:5229–5233
- Bibi E. 2011. Early targeting events during membrane protein biogenesis in Escherichia coli. Biochim Biophys Acta – Biomembr 1808:841–850
- Bibi E. 2012. Is there a twist in the Escherichia coli signal recognition particle pathway? Trends Biochem Sci 37:1–6
- Bieker KL, Silhavy TJ. 1990. The genetics of protein secretion in E. coli. Trends Genet 6:329–334
- Bingel-Erlenmeyer R, Kohler R, Kramer G, Sandikci A, Antolic S, Maier T, et al. 2008. A peptide deformylase-ribosome complex reveals mechanism of nascent chain processing. Nature 452:108–111
- Blau M, Mullapudi S, Becker T, Dudek J, Zimmermann R, Penczek PA, Beckmann R. 2005. ERj1p uses a universal ribosomal adaptor site to coordinate the 80S ribosome at the membrane. Nat Struct Mol Biol 12:1015–1016
- Blobel G, Sabatini DD. 1970. Controlled proteolysis of nascent polypeptides in rat liver cell fractions. I. Location of the polypeptides within ribosomes. J Cell Biol 45:130–145
- Bogdanov M, Dowhan W. 1998. Phospholipid-assisted protein folding: Phosphatidylethanolamine is required at a late step of the conformational maturation of the polytopic membrane protein lactose permease. EMBO J 17:5255–5264
- Bogdanov M, Dowhan W. 2012. Lipid-dependent generation of dual topology for a membrane protein. J Biol Chem 287:37939–37948
- Bogdanov M, Sun J, Kaback HR, Dowhan W. 1996. A phospholipid acts as a chaperone in assembly of a membrane transport protein. J Biol Chem 271:11615–11618
- Boisramé A, Chasles M, Babour A, Beckerich J-M, Gaillardin C. 2002. Sbh1p, a subunit of the Sec61 translocon, interacts with the chaperone calnexin in the yeast Yarrowia lipolytica. J Cell Sci 115:4947–4956
- Bornemann T, Jöckel J, Rodnina MV, Wintermeyer W. 2008. Signal sequence-independent membrane targeting of ribosomes containing short nascent peptides within the exit tunnel. Nat Struct Mol Biol 15:494–499
- Braig D, Bär C, Thumfart J-O, Koch HG. 2009. Two cooperating helices constitute the lipid-binding domain of the bacterial SRP receptor. J Mol Biol 390:401–413
- Braig D, Mircheva M, Sachelaru I, van der Sluis EO, Sturm L, Beckmann R, Koch HG. 2011. Signal sequence-independent SRP-SR complex formation at the membrane suggests an alternative targeting pathway within the SRP cycle. Mol Cell Biol 22:2309–2323
- Brodsky JL, Goeckeler J, Schekman R. 1995. BiP and Sec63p are required for both co- and posttranslational protein translocation into the yeast endoplasmic reticulum. Proc Natl Acad Sci USA 92:9643–9646
- Broude NE. 2011. Analysis of RNA localization and metabolism in single live bacterial cells: Achievements and challenges. Mol Microbiol 80:1137–1147
- Brundage L, Hendrick JP, Schiebel E, Driessen AJM, Wickner W. 1990. The purified E. coli integral membrane protein SecYE is sufficient for reconstitution of SecA-dependent precursor protein translocation. Cell 62:649–657
- Burda P, Aebi M. 1999. The dolichol pathway of N-linked glycosylation. Biochim Biophys Acta – Gen Subj 1426:239–257
- Calo D, Eichler J. 2011. Crossing the membrane in Archaea, the third domain of life. Biochim Biophys Acta – Biomembr 1808:885–891
- Cao TB, Saier MH Jr. 2003. The general protein secretory pathway: phylogenetic analyses leading to evolutionary conclusions. Biochim Biophys Acta – Biomembr 1609:115–125
- Castanié-Cornet M-P, Bruel N, Genevaux P. 2013. Chaperone networking facilitates protein targeting to the bacterial cytoplasmic membrane. Biochim Biophys Acta – Mol Cell Res. doi: 10.1016/j.bbamcr.2013.11.007
- Chang CN, Blobel G, Model P. 1978. Detection of prokaryotic signal peptidase in an Escherichia coli membrane fraction: Endoproteolytic cleavage of nascent f1 pre-coat protein. Proc Natl Acad Sci USA 75:361–365
- Chavan M, Yan A, Lennarz WJ. 2005. Subunits of the translocon interact with components of the oligosaccharyl transferase complex. J Biol Chem 280:22917–22924
- Chen M, Xie K, Jiang F, Yi L, Dalbey REE. 2002. YidC, a newly defined evolutionarily conserved protein, mediates membrane protein assembly in bacteria. Biol Chem 383:1565–1572
- Chen R, Henning U. 1996. A periplasmic protein (Skp) of Escherichia coli selectively binds a class of outer membrane proteins. Mol Microbiol 19:1287–1294
- Chen W, Helenius J, Braakman I, Helenius A. 1995. Cotranslational folding and calnexin binding during glycoprotein synthesis. Proc Natl Acad Sci USA 92:6229–6233
- Cheng Z, Jiang Y, Mandon EC, Gilmore R. 2005. Identification of cytoplasmic residues of Sec61p involved in ribosome binding and cotranslational translocation. J Cell Biol 168:67–77
- Chirico WJ, Waters MG, Blobel G. 1988. 70K heat shock related proteins stimulate protein translocation into microsomes. Nature 332:805–810
- Clemons WM, Ménétret J-F, Akey CW, Rapoport TA. 2004. Structural insight into the protein translocation channel. Curr Opin Struct Biol 14:390–396
- Cline K, Dabney-Smith C. 2008. Plastid protein import and sorting: Different paths to the same compartments. Curr Opin Plant Biol 11:585–592
- Cross BC, High S. 2009. Dissecting the physiological role of selective transmembrane-segment retention at the ER translocon. J Cell Sci 122:1768–1777
- Cunningham K, Lill R, Crooke E, Rice M, Moore K, Wickner W, Oliver D. 1989. SecA protein, a peripheral protein of the Escherichia coli plasma membrane, is essential for the functional binding and translocation of proOmpA. EMBO J 8:955–959
- Czaplinski K, Singer RH. 2006. Pathways for mRNA localization in the cytoplasm. Trends Biochem Sci 31:687–693
- Dalal K, Nguyen N, Alami M, Tan J, Moraes TF, Lee WC, et al. 2009. Structure, binding, and activity of Syd, a SecY-interacting protein. J Biol Chem 284:7897–7902
- Dalbey RE, Lively MO, Bron S, Dijl JMV. 1997. The chemistry and enzymology of the type I signal peptidases. Protein Sci 6:1129–1138
- Dalbey RE, Wang P, Kuhn A. 2011. Assembly of bacterial inner membrane proteins. Annu Rev Biochem 80:161–187
- Dalbey RE, Wang P, van Dijl JM. 2012. Membrane proteases in the bacterial protein secretion and quality control pathway. Microbiol Mol Biol Rev 76:311–330
- Daley DO, Rapp M, Granseth E, Melén K, Drew D, von Heijne G. 2005. Global topology analysis of the Escherichia coli inner membrane proteome. Science 308:1321–1323
- Dartigalongue C, Raina S. 1998. A new heat-shock gene, ppiD, encodes a peptidyl-prolyl isomerase required for folding of outer membrane proteins in Escherichia coli. EMBO J 17:3968–3980
- de Cook H, Tommassen J. 1991. Conservation of components of the Escherichia coli export machinery in prokaryotes. FEMS Microbiol Lett 80:195–199
- de Gier J-WL, Mansournia P, Valent QA, Phillips GJ, Luirink J, von Heijne G. 1996. Assembly of a cytoplasmic membrane protein in Escherichia coli is dependent on the signal recognition particle. FEBS Lett 399:307–309
- de Vrije T, de Swart RL, Dowhan W, Tommassen J, de Kruijff B. 1988. Phosphatidylglycerol is involved in protein translocation across Escherichia coli inner membranes. Nature 334:173–175
- Deitermann S, Sprie GS, Koch HG. 2005. A dual function for SecA in the assembly of single spanning membrane proteins in Escherichia coli. J Biol Chem 280:39077–39085
- Demirci E, Junne T, Baday S, Bernèche S, Spiess M. 2013. Functional asymmetry within the Sec61p translocon. Proc Natl Acad Sci USA 110:18856–18861
- Denic V, Dotsch V, Sinning I. 2013. Endoplasmic reticulum targeting and insertion of tail-anchored membrane proteins by the GET pathway. Cold Spring Harb Perspect Biol 5:a013334
- Deshaies RJ, Koch BD, Werner-Washburne M, Craig EA, Schekman R. 1988. A subfamily of stress proteins facilitates translocation of secretory and mitochondrial precursor polypeptides. Nature 332:800–805
- Deshaies RJ, Sanders SL, Feldheim DA, Schekman R. 1991. Assembly of yeast Sec proteins involved in translocation into the endoplasmic reticulum into a membrane-bound multisubunit complex. Nature 349:806–808
- Deshaies RJ, Schekman R. 1987. A yeast mutant defective at an early stage in import of secretory protein precursors into the endoplasmic reticulum. J Cell Biol 105:633–645
- Deshaies RJ, Schekman R. 1989. SEC62 encodes a putative membrane protein required for protein translocation into the yeast endoplasmic reticulum. J Cell Biol 109:2653–2664
- Deuerling E, Patzelt H, Vorderwülbecke S, Rauch T, Kramer G, Schaffitzel E, et al. 2003. Trigger factor and DnaK possess overlapping substrate pools and binding specificities. Mol Microbiol 47:1317–1328
- Deville K, Gold VAM, Robson A, Whitehouse S, Sessions RB, Baldwin SA, et al. 2011. The oligomeric state and arrangement of the active bacterial translocon. J Biol Chem 286:4659–4669
- Dierks T, Volkmer J, Schlenstedt G, Jung C, Sandholzer U, Zachmann K, et al. 1996. A microsomal ATP-binding protein involved in efficient protein transport into the mammalian endoplasmic reticulum. EMBO J 15:6931–6942
- Douville K, Price A, Eichler J, Economou A, Wickner W. 1995. SecYEG and SecA are the stoichiometric components of preprotein translocase. J Biol Chem 270:20106–20111
- Dowhan W, Bogdanov M. 2009. Lipid-dependent membrane protein topogenesis. Annu Rev Biochem 78:515–540
- Drew D, Fröderberg L, Baars L, de Gier J-WL. 2003. Assembly and overexpression of membrane proteins in Escherichia coli. Biochim Biophys Acta – Biomembr 1610:3–10
- Driessen AJ, Wickner W. 1991. Proton transfer is rate-limiting for translocation of precursor proteins by the Escherichia coli translocase. Proc Natl Acad Sci USA 88:2471–2475
- Driessen AJM, Nouwen N. 2008. Protein translocation across the bacterial cytoplasmic membrane. Annu Rev Biochem 77:643–667
- Dudek J, Greiner M, Muller A, Hendershot LM, Kopsch K, Nastainczyk W, Zimmermann R. 2005. ERj1p has a basic role in protein biogenesis at the endoplasmic reticulum. Nat Struct Mol Biol 12:1008–1014
- Dudek J, Volkmer J, Bies C, Guth S, Muller A, Lerner M, et al. 2002. A novel type of co-chaperone mediates transmembrane recruitment of DnaK-like chaperones to ribosomes. EMBO J 21:2958–2967
- Dünnwald M, Varshavsky A, Johnsson N. 1999. Detection of transient in vivo interactions between substrate and transporter during protein translocation into the endoplasmic reticulum. Mol Cell Biol 10:329–344
- Duong F, Wickner W. 1997a. The SecDFyajC domain of preprotein translocase controls preprotein movement by regulating SecA membrane cycling. EMBO J 16:4871–4879
- Duong F, Wickner W. 1997b. Distinct catalytic roles of the SecYE, SecG and SecDFyajC subunits of preprotein translocase holoenzyme. EMBO J 16:2756–2768
- Egea PF, Napetschnig J, Walter P, Stroud RM. 2008. Structures of SRP54 and SRP19, the two proteins that organize the ribonucleic core of the signal recognition particle from Pyrococcus furiosus. PLoS One 3:e3528
- Egea PF, Shan SO, Napetschnig J, Savage DF, Walter P, Stroud RM. 2004. Substrate twinning activates the signal recognition particle and its receptor. Nature 427:215–221
- Egea PF, Stroud RM. 2010. Lateral opening of a translocon upon entry of protein suggests the mechanism of insertion into membranes. Proc Natl Acad Sci USA 107:17182–17187
- Eichler J. 2003. Evolution of the prokaryotic protein translocation complex: A comparison of archaeal and bacterial versions of SecDF. Mol Phylogen Evol 27:504–509
- Eitan A, Bibi E. 2004. The core Escherichia coli signal recognition particle receptor contains only the N and G domains of FtsY. J Bacteriol 186:2492–2494
- Emr SD, Hanley-Way S, Silhavy TJ. 1981. Suppressor mutations that restore export of a protein with a defective signal sequence. Cell 23:79–88
- Enquist K, Fransson M, Boekel C, Bengtsson I, Geiger K, Lang L, et al. 2009. Membrane-integration characteristics of two ABC transporters, CFTR and P-glycoprotein. J Mol Biol 387:1153–1164
- Epand RM, Epand RF. 2011. Functional consequences of the lateral organization of biological membranes. In: Yeagle PL, ed. The Structure of Biological Membranes, 3rd ed. Boca Raton, FL: CRC Press
- Erdmann F, Schäuble N, Lang S, Jung M, Honigmann A, Ahmad M, et al. 2011. Interaction of calmodulin with Sec61α limits Ca2+ leakage from the endoplasmic reticulum. EMBO J 30:17–31
- Erlandson KJ, Miller SBM, Nam Y, Osborne AR, Zimmer J, Rapoport TA. 2008. A role for the two-helix finger of the SecA ATPase in protein translocation. Nature 455:984–987
- Facey SJ, Neugebauer SA, Krauss S, Kuhn A. 2007. The mechanosensitive channel protein MscL is targeted by the SRP to the novel YidC membrane insertion pathway of Escherichia coli. J Mol Biol 365:995–1004
- Fang H, Green N. 1994. Nonlethal sec71-1 and sec72-1 mutations eliminate proteins associated with the Sec63p-BiP complex from S. cerevisiae. Mol Cell Biol 5:933–942
- Fekkes P, De Wit JG, Van Der Wolk JPW, Kimsey HH, Kumamoto CA, Driessen AJM. 1998. Preprotein transfer to the Escherichia coli translocase requires the co-operative binding of SecB and the signal sequence to SecA. Mol Microbiol 29:1179–1190
- Feldheim D, Yoshimura K, Admon A, Schekman R. 1993. Structural and functional characterization of Sec66p, a new subunit of the polypeptide translocation apparatus in the yeast endoplasmic reticulum. Mol Cell Biol 4:931–939
- Ferbitz L, Maier T, Patzelt H, Bukau B, Deuerling E, Ban N. 2004. Trigger factor in complex with the ribosome forms a molecular cradle for nascent proteins. Nature 431:590–596
- Finke K, Plath K, Panzner S, Prehn S, Rapoport TA, Hartmann E, Sommer T. 1996. A second trimeric complex containing homologs of the Sec61p complex functions in protein transport across the ER membrane of S. cerevisiae. EMBO J 15:1482–1494
- Flanagan JJ, Chen JC, Miao Y, Shao Y, Lin J, Bock PE, Johnson AE. 2003. Signal recognition particle binds to ribosome-bound signal sequences with fluorescence-detected subnanomolar affinity that does not diminish as the nascent chain lengthens. J Biol Chem 278:18628–18637
- Flourakis M, Van Coppenolle F, Lehen’kyi Vy, Beck B, Skryma R, Prevarskaya N. 2006. Passive calcium leak via translocon is a first step for iPLA2-pathway regulated store operated channels activation. FASEB J 20:1215–1217
- Flower AM. 2001. SecG function and phospholipid metabolism in Escherichia coli. J Bacteriol 183:2006–2012
- Flower AM, Hines LL, Pfennig PL. 2000. SecG is an auxiliary component of the protein export apparatus of Escherichia coli. Mol Gen Genet 263:131–136
- Focia PJ, Shepotinovskaya IV, Seidler JA, Freymann DM. 2004. Heterodimeric GTPase core of the SRP targeting complex. Science 303:373–377
- Fons RD, Bogert BA, Hegde RS. 2003. Substrate-specific function of the translocon-associated protein complex during translocation across the ER membrane. J Cell Biol 160:529–539
- Frauenfeld J, Gumbart J, v d Sluis EO, Funes S, Gartmann M, Beatrix B, et al. 2011. Cryo-EM structure of the ribosome-SecYE complex in the membrane environment. Nat Struct Mol Biol 18:614–621
- Fulga TA, Sinning I, Dobberstein B, Pool MR. 2001. SRbeta coordinates signal sequence release from SRP with ribosome binding to the translocon. EMBO J 20:2338–2347
- Garcia PD, Walter P. 1988. Full-length prepro-alpha-factor can be translocated across the mammalian microsomal membrane only if translation has not terminated. J Cell Biol 106:1043–1048
- Gatsos X, Perry AJ, Anwari K, Dolezal P, Wolynec PP, Likić VA, et al. 2008. Protein secretion and outer membrane assembly in Alphaproteobacteria. FEMS Microbiol Rev 32:995–1009
- Gelis I, Bonvin AMJJ, Keramisanou D, Koukaki M, Gouridis G, Karamanou S, et al. 2007. Structural basis for signal-sequence recognition by the translocase motor SecA as determined by NMR. Cell 131:756–769
- Gilmore R, Blobel G, Walter P. 1982a. Protein translocation across the endoplasmic reticulum. I. Detection in the microsomal membrane of a receptor for the signal recognition particle. J Cell Biol 95:463–469
- Gilmore R, Walter P, Blobel G. 1982b. Protein translocation across the endoplasmic reticulum. II. Isolation and characterization of the signal recognition particle receptor. J Cell Biol 95:470–477
- Goder V, Bieri C, Spiess M. 1999. Glycosylation can influence topogenesis of membrane proteins and reveals dynamic reorientation of nascent polypeptides within the translocon. J Cell Biol 147:257–266
- Gogala M, Becker T, Beatrix B, Armache J-P, Barrio-Garcia C, Berninghausen O, Beckmann R. 2014. Structures of the Sec61 complex engaged in nascent peptide translocation or membrane insertion. Nature 506:107–110
- Gold VAM, Robson A, Bao H, Romantsov T, Duong F, Collinson I. 2010. The action of cardiolipin on the bacterial translocon. Proc Natl Acad Sci USA 107:10044–10049
- Gordon SM, Kindt TJ. 1976. BIP: A low molecular weight protein coisolated with beta-2 microglublin. Biochem a Biophys Res Commun 72:984–990
- Gorlich D, Rapoport TA. 1993. Protein translocation into proteoliposomes reconstituted from purified components of the endoplasmic reticulum membrane. Cell 75:615–630
- Görlich D, Hartmann E, Prehn S, Rapoport TA. 1992. A protein of the endoplasmic reticulum involved early in polypeptide translocation. Nature 357:47–52
- Götz C, Müller A, Montenarh M, Zimmermann R, Dudek J. 2009. The ER-membrane-resident Hsp40 ERj1 is a novel substrate for protein kinase CK2. Biochem a Biophys Res Commun 388:637–642
- Gouridis G, Karamanou S, Gelis I, Kalodimos CG, Economou A. 2009. Signal peptides are allosteric activators of the protein translocase. Nature 462:363–367
- Gruss OJ, Feick P, Frank R, Dobberstein B. 1999. Phosphorylation of components of the ER translocation site. Eur J Biochem 260:785–793
- Gu SQ, Peske F, Wieden HJ, Rodnina MV, Wintermeyer W. 2003. The signal recognition particle binds to protein L23 at the peptide exit of the Escherichia coli ribosome. RNA 9:566–573
- Gumbart J, Schulten K. 2008. The roles of pore ring and plug in the SecY protein-conducting channel. J Gen Physiol 132:709–719
- Gumbart J, Trabuco LG, Schreiner E, Villa E, Schulten K. 2009. Regulation of the protein-conducting channel by a bound ribosome. Structure 17:1453–1464
- Gutierrez JA, Crowley PJ, Cvitkovitch DG, Brady LJ, Hamilton IR, Hillman JD, Bleiweis AS. 1999. Streptococcus mutans ffh, a gene encoding a homologue of the 54 kDa subunit of the signal recognition particle, is involved in resistance to acid stress. Microbiology 145:357–366
- Hainzl T, Huang SH, Sauer-Eriksson AE. 2002. Structure of the SRP19-RNA complex and implications for signal recognition particle assembly. Nature 417:767–771
- Hainzl T, Huang SH, Sauer-Eriksson AE. 2005. Structural insights into SRP RNA: An induced fit mechanism for SRP assembly. RNA 11:1043–1050
- Halic M, Becker T, Pool MR, Spahn CM, Grassucci RA, Frank J, Beckmann R. 2004. Structure of the signal recognition particle interacting with the elongation-arrested ribosome. Nature 427:808–814
- Halic M, Blau M, Becker T, Mielke T, Pool MR, Wild K, Sinning I, Beckmann R. 2006. Following the signal sequence from ribosomal tunnel exit to signal recognition particle. Nature 444:507–511
- Hamman BD, Chen J-C, Johnson EE, Johnson AE. 1997. The aqueous pore through the translocon has a diameter of 40–60 Å during cotranslational protein translocation at the ER membrane. Cell 89:535–544
- Hamman BD, Hendershot LM, Johnson AE. 1998. BiP maintains the permeability barrier of the ER membrane by sealing the lumenal end of the translocon pore before and early in translocation. Cell 92:747–758
- Hand NJ, Klein R, Laskewitz A, Pohlschröder M. 2006. Archaeal and bacterial SecD and SecF homologs exhibit striking structural and functional conservation. J Bacteriol 188:1251–1259
- Hann BC, Walter P. 1991. The signal recognition particle in S. cerevisiae. Cell 67:131–144
- Harada Y, Li H, Lennarz WJ. 2009. Oligosaccharyltransferase directly binds to ribosome at a location near the translocon-binding site. Proc Natl Acad Sci USA 106:6945–6949
- Harms N, Koningstein G, Dontje W, Muller M, Oudega B, Luirink J, de Cock H. 2001. The early interaction of the outer membrane protein phoe with the periplasmic chaperone Skp occurs at the cytoplasmic membrane. J Biol Chem 276:18804–18811
- Harris CR, Silhavy TJ. 1999. Mapping an interface of SecY (PrlA) and SecE (PrlG) by using synthetic phenotypes and in vivo cross-linking. J Bacteriol 181:3438–3444
- Hartman H, Smith TF. 2010. GTPases and the origin of the ribosome. Biol Direct 5:36
- Hartmann E, Gorlich D, Kostka S, Otto A, Kraft R, Knespel S, et al. 1993. A tetrameric complex of membrane proteins in the endoplasmic reticulum. Eur J Biochem 214:375–381
- Hartmann E, Sommer T, Prehn S, Görlich D, Jentsch S, Rapoport TA. 1994. Evolutionary conservation of components of the protein translocation complex. Nature 367:654–657
- Hasona A, Crowley PJ, Levesque CM, Mair RW, Cvitkovitch DG, Bleiweis AS, Brady LJ. 2005. Streptococcal viability and diminished stress tolerance in mutants lacking the signal recognition particle pathway or YidC2. Proc Natl Acad Sci USA 102:17466–17471
- Hegde RS, Bernstein HD. 2006. The surprising complexity of signal sequences. Trends Biochem Sci 31:563–571
- Hegde RS, Keenan RJ. 2011. Tail-anchored membrane protein insertion into the endoplasmic reticulum. Nat Rev Mol Cell biol 12:787–798
- Hegde RS, Voigt S, Rapoport TA, Lingappa VR. 1998. TRAM regulates the exposure of nascent secretory proteins to the cytosol during translocation into the endoplasmic reticulum. Cell 92:621–631
- Heinrich SU, Mothes W, Brunner J, Rapoport TA. 2000. The Sec61p complex mediates the integration of a membrane protein by allowing lipid partitioning of the transmembrane domain. Cell 102:233–244
- Heinrich SU, Rapoport TA. 2003. Cooperation of transmembrane segments during the integration of a double-spanning protein into the ER membrane. EMBO J 22:3654–3663
- Helmers J, Schmidt D, Glavy JS, Blobel G, Schwartz T. 2003. The beta-subunit of the protein-conducting channel of the endoplasmic reticulum functions as the guanine nucleotide exchange factor for the beta-subunit of the signal recognition particle receptor. J Biol Chem 278:23686–23690
- Hermesh O, Jansen R-P. 2013. Take the (RN)A-train: Localization of mRNA to the endoplasmic reticulum. Biochim Biophys Acta – Mol Cell Res 1833:2519–2525
- Herskovits AA, Bibi E. 2000. Association of Escherichia coli ribosomes with the inner membrane requires the signal recognition particle receptor but is independent of the signal recognition particle. Proc Natl Acad Sci USA 97:4621–4626
- Herskovits AA, Shimoni E, Minsky A, Bibi E. 2002. Accumulation of endoplasmic membranes and novel membrane-bound ribosome-signal recognition particle receptor complexes in Escherichia coli. J Cell Biol 159:403–410
- Hessa T, Kim H, Bihlmaier K, Lundin C, Boekel J, Andersson H, et al. 2005. Recognition of transmembrane helices by the endoplasmic reticulum translocon. Nature 433:377–381
- Higy M, Gander S, Spiess M. 2005. Probing the environment of signal-anchor sequences during topogenesis in the endoplasmic reticulum. Biochemistry 44:2039–2047
- Hizlan D, Robson A, Whitehouse S, Gold Vicki A, Vonck J, Mills D, et al. 2012. Structure of the SecY complex unlocked by a preprotein mimic. Cell Rep 1:21–28
- Hoffmann A, Becker Annemarie H, Zachmann-Brand B, Deuerling E, Bukau B, Kramer G. 2012. Concerted action of the ribosome and the associated chaperone trigger factor confines nascent polypeptide folding. Mol Cell 48:63–74
- Holtkamp W, Lee S, Bornemann T, Senyushkina T, Rodnina MV, Wintermeyer W. 2012. Dynamic switch of the signal recognition particle from scanning to targeting. Nat Struct Mol Biol 19:1332–1337
- Hori O, Miyazaki M, Tamatani T, Ozawa K, Takano K, Okabe M, et al. 2006. Deletion of SERP1/RAMP4, a component of the endoplasmic reticulum (ER) translocation sites, leads to ER stress. Mol Cell Biol 26:4257–4267
- Hou B, Lin PJ, Johnson AE. 2012. Membrane protein TM segments are retained at the translocon during integration until the nascent chain cues FRET-detected release into bulk lipid. Mol Cell 48:398–408
- Houben EN, Zarivach R, Oudega B, Luirink J. 2005. Early encounters of a nascent membrane protein: specificity and timing of contacts inside and outside the ribosome. J Cell Biol 170:27–35
- Huber D, Boyd D, Xia Y, Olma MH, Gerstein M, Beckwith J. 2005. Use of thioredoxin as a reporter to identify a subset of Escherichia coli signal sequences that promote signal recognition particle-dependent translocation. J Bacteriol 187:2983–2991
- Huber D, Rajagopalan N, Preissler S, Rocco MA, Merz F, Kramer G, Bukau B. 2011. SecA interacts with ribosomes in order to facilitate posttranslational translocation in bacteria. Mol Cell 41:343–353
- Hunt JF, Weinkauf S, Henry L, Fak JJ, McNicholas P, Oliver DB, Deisenhofer J. 2002. Nucleotide control of interdomain interactions in the conformational reaction cycle of SecA. Science 297:2018–2026
- Ingolia NT, Ghaemmaghami S, Newman JRS, Weissman JS. 2009. Genome-wide analysis in vivo of translation with nucleotide resolution using ribosome profiling. Science 324:218–223
- Ismail N, Crawshaw SG, Cross BCS, Haagsma AC, High S. 2008. Specific transmembrane segments are selectively delayed at the ER translocon during opsin biogenesis. Biochem J 411:495–506
- Ismail N, Hedman R, Schiller N, von Heijne G. 2012. A biphasic pulling force acts on transmembrane helices during translocon-mediated membrane integration. Nat Struct Mol Biol 19:1018–1022
- Ito K, Akiyama Y. 2005. Cellular functions, mechanism of action, and regulation of FtsH protease. Annul Rev Microbiol 59:211–231
- Ito K, Wittekind M, Nomura M, Shiba K, Yura T, Miura A, Nashimoto H. 1983. A temperature-sensitive mutant of E. coli exhibiting slow processing of exported proteins. Cell 32:789–797
- Jagath JR, Matassova NB, de Leeuw E, Warnecke JM, Lentzen G, Rodnina MV, et al. 2001. Important role of the tetraloop region of 4.5S RNA in SRP binding to its receptor FtsY. RNA 7:293–301
- Janska H, Kwasniak M, Szczepanowska J. 2013. Protein quality control in organelles – AAA/FtsH story. Biochim Biophys Acta – Mol Cell Res 1833:381–387
- Jaqaman K, Grinstein S. 2012. Regulation from within: The cytoskeleton in transmembrane signaling. Trends Cell Biol 22:515–526
- Jarchow S, Luck C, Gorg A, Skerra A. 2008. Identification of potential substrate proteins for the periplasmic Escherichia coli chaperone Skp. Proteomics 8:4987–4994
- Jermy AJ, Willer M, Davis E, Wilkinson BM, Stirling CJ. 2006. The Brl domain in Sec63p is required for assembly of functional endoplasmic reticulum translocons. J Biol Chem 281:7899–7906
- Jiang Y, Cheng Z, Mandon EC, Gilmore R. 2008. An interaction between the SRP receptor and the translocon is critical during cotranslational protein translocation. J Cell Biol 180:1149–1161
- Johnson N, Haβdenteufel S, Theis M, Paton AW, Paton JC, Zimmermann R, High S. 2013. The signal sequence influences post-translational ER translocation at distinct stages. PLoS ONE 8:e75394
- Josefsson LG, Randall LL. 1981a. Different exported proteins in E. coli show differences in the temporal mode of processing in vivo. Cell 25:151–157
- Josefsson LG, Randall LL. 1981b. Processing in vivo of precursor maltose-binding protein in Escherichia coli occurs post-translationally as well as co-translationally. J Biol Chem 256:2504–2507
- Junne T, Schwede T, Goder V, Spiess M. 2006. The plug domain of yeast Sec61p is important for efficient protein translocation, but is not essential for cell viability. Mol Cell Biol 17:4063–4068
- Kalies K-U, Rapoport TA, Hartmann E. 1998. The β subunit of the Sec61 complex facilitates cotranslational protein transport and interacts with the signal peptidase during translocation. J Cell Biol 141:887–894
- Karamyshev AL, Johnson AE. 2005. Selective SecA association with signal sequences in ribosome-bound nascent chains: A potential role for SecA in ribosome targeting to the bacterial membrane. J Biol Chem 280:37930–37940
- Karaoglu D, Kelleher DJ, Gilmore R. 1997. The highly conserved Stt3 protein is a subunit of the yeast oligosaccharyltransferase and forms a subcomplex with Ost3p and Ost4p. J Biol Chem 272:32513–32520
- Keenan RJ, Freymann DM, Walter P, Stroud RM. 1998. Crystal structure of the signal sequence binding subunit of the signal recognition particle. Cell 94:181–191
- Kelkar A, Dobberstein B. 2009. Sec61beta, a subunit of the Sec61 protein translocation channel at the endoplasmic reticulum, is involved in the transport of Gurken to the plasma membrane. BMC Cell Biol 10:11
- Keller R, de Keyzer J, Driessen AJM, Palmer T. 2012. Co-operation between different targeting pathways during integration of a membrane protein. J Cell Biol 199:303–315
- Keller RCA. 2011. The prediction of novel multiple lipid-binding regions in protein translocation motor proteins: A possible general feature. Cell Mol Biol Lett 16:40–54
- Kihara A, Akiyama Y, Ito K. 1995. FtsH is required for proteolytic elimination of uncomplexed forms of SecY, an essential protein translocase subunit. Proc Natl Acad Sci USA 92:4532–4536
- Kim S, Coulombe PA. 2010. Emerging role for the cytoskeleton as an organizer and regulator of translation. Nat Rev Mol Cell biol 11:75–81
- Kinch LN, Saier JMH, Grishin NV. 2002. Sec61β – a component of the archaeal protein secretory system. Trends Biochem Sci 27:170–171
- Knoblauch NTM, Rüdiger S, Schönfeld H-J, Driessen AJM, Schneider-Mergener J, Bukau B. 1999. Substrate specificity of the SecB chaperone. J Biol Chem 274:34219–34225
- Knyazev DG, Lents A, Krause E, Ollinger N, Siligan C, Papinski D, et al. 2013. The bacterial translocon SecYEG opens upon ribosome binding. J Biol Chem 288:17941–17946
- Koch HG, Hengelage T, Neumann-Haefelin C, MacFarlane J, Hoffschulte HK, Schimz K-L, et al. 1999. In vitro studies with purified components reveal signal recognition particle (SRP) and SecA/SecB as constituents of two independent protein-targeting pathways of Escherichia coli. Mol Cell Biol 10:2163–2173
- Koch HG, Müller M. 2000. Dissecting the translocase and integrase functions of the Escherichia coli SecYEG translocon. J Cell Biol 150:689–694
- Köhler R, Boehringer D, Greber B, Bingel-Erlenmeyer R, Collinson I, Schaffitzel C, Ban N. 2009. YidC and Oxa1 form dimeric insertion pores on the translating ribosome. Mol Cell 34:344–353
- Kramer G, Boehringer D, Ban N, Bukau B. 2009. The ribosome as a platform for co-translational processing, folding and targeting of newly synthesized proteins. Nat Struct Mol Biol 16:589–597
- Kramer G, Rauch T, Rist W, Vorderwulbecke S, Patzelt H, Schulze-Specking A, et al. 2002. L23 protein functions as a chaperone docking site on the ribosome. Nature 419:171–174
- Kraut-Cohen J, Afanasieva E, Haim-Vilmovsky L, Slobodin B, Yosef I, Bibi E, Gerst JE. 2013. Translation- and SRP-independent mRNA targeting to the endoplasmic reticulum in the yeast Saccharomyces cerevisiae. Mol Cell Biol 24:3069–3084
- Kraut-Cohen J, Gerst JE. 2010. Addressing mRNAs to the ER: Cis sequences act up!. Trends Biochem Sci 35:459–469
- Krehenbrink M, Edwards A, Downie JA. 2011. The superoxide dismutase SodA is targeted to the periplasm in a SecA-dependent manner by a novel mechanism. Mol Microbiol 82:164–179
- Krieg UC, Johnson AE, Walter P. 1989. Protein translocation across the endoplasmic reticulum membrane: identification by photocross-linking of a 39-kD integral membrane glycoprotein as part of a putative translocation tunnel. J Cell Biol 109:2033–2043
- Kroczynska B, Evangelista CM, Samant SS, Elguindi EC, Blond SY. 2004. The SANT2 domain of the murine tumor cell DnaJ-like protein 1 human homologue interacts with α1-antichymotrypsin and kinetically interferes with its serpin inhibitory activity. J Biol Chem 279:11432–11443
- Kudva R, Denks K, Kuhn P, Vogt A, Muller M, Koch HG, Müller M. 2013. Protein translocation across the inner membrane of Gram-negative bacteria: The Sec and Tat dependent protein transport pathways. Res Microbiol 164:505–534
- Kuhn P, Weiche B, Sturm L, Sommer E, Drepper F, Warscheid B, et al. 2011. The bacterial SRP receptor, SecA and the ribosome use overlapping binding sites on the SecY translocon. Traffic 12:563–578
- Kuhn P, Kudva R, Welte T, Sturm L, Koch HG. 2014. Targeting and integration of bacterial membrane proteins. In: Remi Fronzes HR, ed. Bacterial Membranes: Structural and Molecular Biology. Norwich, UK: Caister Academic Press; Horizon Scientific Press
- Kurzchalia TV, Wiedmann M, Girshovich AS, Bochkareva ES, Bielka H, Rapoport TA. 1986. The signal sequence of nascent preprolactin interacts with the 54K polypeptide of the signal recognition particle. Nature 320:634–636
- Kusters R, Dowhan W, de Kruijff B. 1991. Negatively charged phospholipids restore prePhoE translocation across phosphatidylglycerol-depleted Escherichia coli inner membranes. J Biol Chem 266:8659–8662
- Lakkaraju AK, Mary C, Scherrer A, Johnson AE, Strub K. 2008. SRP keeps polypeptides translocation-competent by slowing translation to match limiting ER-targeting sites. Cell 133:440–451
- Lakkaraju AKK, Abrami L, Lemmin T, Blaskovic S, Kunz B, Kihara A, et al. 2012a. Palmitoylated calnexin is a key component of the ribosome-translocon complex. EMBO J 31:1823–1835
- Lakkaraju AKK, Thankappan R, Mary C, Garrison JL, Taunton J, Strub K. 2012b. Efficient secretion of small proteins in mammalian cells relies on Sec62-dependent posttranslational translocation. Mol Cell Biol 23:2712–2722
- Lam VQ, Akopian D, Rome M, Henningsen D, Shan SO. 2010. Lipid activation of the signal recognition particle receptor provides spatial coordination of protein targeting. J Cell Biol 190:623–635
- Lang S, Benedix J, Fedeles SV, Schorr S, Schirra C, Schäuble N, et al. 2012. Different effects of Sec61α, Sec62 and Sec63 depletion on transport of polypeptides into the endoplasmic reticulum of mammalian cells. J Cell Sci 125:1958–1969
- Larsen N, Zwieb C. 1993. The signal recognition particle database (SRPDB). Nucleic Acids Res 21:3019–3020
- Lau JT, Welply JK, Shenbagamurthi P, Naider F, Lennarz WJ. 1983. Substrate recognition by oligosaccharyl transferase. Inhibition of co-translational glycosylation by acceptor peptides. J Biol Chem 258:15255–15260
- Lee AH, Iwakoshi NN, Glimcher LH. 2003. XBP-1 regulates a subset of endoplasmic reticulum resident chaperone genes in the unfolded protein response. Mol Cell Biol 23:7448–7459
- Lee HC, Bernstein HD. 2001. The targeting pathway of Escherichia coli presecretory and integral membrane proteins is specified by the hydrophobicity of the targeting signal. Proc Natl Acad Sci USA 98:3471–3476
- Leung E, Brown JD. 2010. Biogenesis of the signal recognition particle. Biochem Soc Trans 38:1093–1098
- Leznicki P, Clancy A, Schwappach B, High S. 2010. Bat3 promotes the membrane integration of tail-anchored proteins. J Cell Sci 123:2170–2178
- Li W, Schulman S, Boyd D, Erlandson K, Beckwith J, Rapoport TA. 2007. The plug domain of the SecY protein stabilizes the closed state of the translocation channel and maintains a membrane seal. Mol Cell 26:511–521
- Liang H, VanValkenburgh C, Chen X, Mullins C, Van Kaer L, Green N, Fang H. 2003. Genetic complementation in yeast reveals functional similarities between the catalytic subunits of mammalian signal peptidase complex. J Biol Chem 278:50932–50939
- Liao S, Lin J, Do H, Johnson AE. 1997. Both lumenal and cytosolic gating of the aqueous ER translocon pore are regulated from inside the ribosome during membrane protein integration. Cell 90:31–41
- Lill R, Dowhan W, Wickner W. 1990. The ATPase activity of SecA is regulated by acidic phospholipids, SecY, and the leader and mature domains of precursor proteins. Cell 60:271–280
- Lithgow T, Waksman G. 2013. Seaside transportation – from structure to function of translocation machines. EMBO Rep 14:585–587
- Loibl M, Wunderle L, Hutzler J, Schulz BL, Aebi M, Strahl S. 2014. Protein O-mannosyltransferases associate with the translocon to modify translocating polypeptide chains. J Biol Chem 289:8599–8611
- Luirink J, High S, Wood H, Giner A, Tollervey D, Dobberstein B. 1992. Signal sequence recognition by an Escherichia coli ribonucleoprotein complex. Nature 359:741–743
- Luirink J, ten Hagen-Jongman CM, van der Weijden CC, Oudega B, High S, Dobberstein B, Kusters R. 1994. An alternative protein targeting pathway in Escherichia coli: studies on the role of ftsY. EMBO J 13:2289–2296
- Lütcke H, High S, Römisch K, Ashford A, Dobberstein B. 1992. The methionine-rich domain of the 54 kDa subunit of signal recognition particle is sufficient for the interaction with signal sequences. EMBO J 11:1543–1551
- Lycklama a Nijeholt JA, de Keyzer J, Prabudiansyah I, Driessen AJM. 2013. Characterization of the supporting role of SecE in protein translocation. FEBS Lett 587:3083–3088
- Lyman SK, Schekman R. 1995. Interaction between BiP and Sec63p is required for the completion of protein translocation into the ER of Saccharomyces cerevisiae. J Cell Biol 131:1163–1171
- Lyman SK, Schekman R. 1997. Binding of secretory precursor polypeptides to a translocon subcomplex is regulated by BiP. Cell 88:85–96
- Macfarlane J, Muller M. 1995. The functional integration of a polytopic membrane protein of Escherichia coli is dependent on the bacterial signal-recognition particle. Eur J Biochem 233:766–771
- Mades A, Gotthardt K, Awe K, Stieler J, Döring T, Füser S, Prange R. 2012. Role of human Sec63 in modulating the steady-state levels of multi-spanning membrane proteins. PLoS One 7:e49243
- Maillard AP, Lalani S, Silva F, Belin D, Duong F. 2007. Deregulation of the SecYEG translocation channel upon removal of the plug domain. J Biol Chem 282:1281–1287
- Martoglio B, Hofmann MW, Brunner J, Dobberstein B. 1995. The protein-conducting channel in the membrane of the endoplasmic reticulum is open laterally toward the lipid bilayer. Cell 81:207–214
- Matern Y, Barion B, Behrens-Kneip S. 2010. PpiD is a player in the network of periplasmic chaperones in Escherichia coli. BMC Microbiol 10:251
- Matlack KES, Misselwitz B, Plath K, Rapoport TA. 1999. BiP acts as a molecular ratchet during posttranslational transport of prepro-α factor across the ER membrane. Cell 97:553–564
- Ménétret J-F, Hegde RS, Heinrich SU, Chandramouli P, Ludtke SJ, Rapoport TA, Akey CW. 2005. Architecture of the ribosome-channel complex derived from native membranes. J Mol Biol 348:445–457
- Ménétret J-F, Schaletzky J, Clemons WM, Osborne AR, Skånland SS, Denison C, et al. 2007. Ribosome binding of a single copy of the SecY complex: Implications for protein translocation. Mol Cell 28:1083–1092
- Ménétret JF, Hegde RS, Aguiar M, Gygi SP, Park E, Rapoport TA, et al. 2008. Single copies of Sec61 and TRAP associate with a nontranslating mammalian ribosome. Structure 16:1126–1137
- Merdanovic M, Clausen T, Kaiser M, Huber R, Ehrmann M. 2011. Protein quality control in the bacterial periplasm. Annul Rev Microbiol 65:149–168
- Meyer H-A, Grau H, Kraft R, Kostka S, Prehn S, Kalies K-U, Hartmann E. 2000. Mammalian Sec61 is associated with Sec62 and Sec63. J Biol Chem 275:14550–14557
- Mikhaleva NI, Golovastov VV, Zolov SN, Bogdanov MV, Dowhan W, Nesmeyanova MA. 2001. Depletion of phosphatidylethanolamine affects secretion of Escherichia coli alkaline phosphatase and its transcriptional expression. FEBS Lett 493:85–90
- Miller JD, Bernstein HD, Walter P. 1994. Interaction of E. coli Ffh/4.5S ribonucleoprotein and FtsY mimics that of mammalian signal recognition particle and its receptor. Nature 367:657–659
- Millman JS, Qi HY, Vulcu F, Bernstein HD, Andrews DW. 2001. FtsY binds to the Escherichia coli inner membrane via interactions with phosphatidylethanolamine and membrane proteins. J Biol Chem 276:25982–25989
- Mircheva M, Boy D, Weiche B, Hucke F, Graumann P, Koch HG. 2009. Predominant membrane localization is an essential feature of the bacterial signal recognition particle receptor. BMC Biol 7:76
- Missiakas D, Betton J-M, Raina S. 1996. New components of protein folding in extracytoplasmic compartments of Escherichia coli SurA, FkpA and Skp/OmpH. Mol Microbiol 21:871–884
- Mitra K, Schaffitzel C, Shaikh T, Tama F, Jenni S, Brooks CL, et al. 2005. Structure of the E. coli protein-conducting channel bound to a translating ribosome. Nature 438:318–324
- Montero Llopis P, Jackson AF, Sliusarenko O, Surovtsev I, Heinritz J, Emonet T, Jacobs-Wagner C. 2010. Spatial organization of the flow of genetic information in bacteria. Nature 466:77–81
- Mori H, Ito K. 2006. Different modes of SecY-SecA interactions revealed by site-directed in vivo photo-cross-linking. Proc Natl Acad Sci USA 103:16159–16164
- Morita K, Tokuda H, Nishiyama K-i. 2012. Multiple SecA molecules drive protein translocation across a single translocon with SecG inversion. J Biol Chem 287:455–464
- Moser M, Nagamori S, Huber M, Tokuda H, Nishiyama K-i. 2013. Glycolipozyme MPIase is essential for topology inversion of SecG during preprotein translocation. Proc Natl Acad Sci USA 110:9734–9739
- Müller L, de Escauriaza MD, Lajoie P, Theis M, Jung M, Müller A, et al. 2010. Evolutionary gain of function for the ER membrane protein Sec62 from yeast to humans. Mol Cell Biol 21:691–703
- Müller M, Koch HG, Beck K, Schafer U. 2001. Protein traffic in bacteria: Multiple routes from the ribosome to and across the membrane. Prog Nucleic Acid Res Mol Biol 66:107–157
- Nagamori S, Smirnova IN, Kaback HR. 2004. Role of YidC in folding of polytopic membrane proteins. J Cell Biol 165:53–62
- Nakashima K, Ishida H, Nakatomi A, Yazawa M. 2012. Specific conformation and Ca2+-binding mode of yeast calmodulin: Insight into evolutionary development. J Bacteriol 152:27–35
- Nam S-E, Paetzel M. 2013. Structure of signal peptide peptidase A with C-termini bound in the active sites: Insights into specificity, self-processing, and regulation. Biochemistry 52:8811–8822
- Neumann-Haefelin C, Schafer U, Muller M, Koch HG. 2000. SRP-dependent co-translational targeting and SecA-dependent translocation analyzed as individual steps in the export of a bacterial protein. EMBO J 19:6419–6426
- Nevo-Dinur K, Nussbaum-Shochat A, Ben-Yehuda S, Amster-Choder O. 2011. Translation-independent localization of mRNA in E. coli. Science 331:1081–1084
- Ng DT, Brown JD, Walter P. 1996. Signal sequences specify the targeting route to the endoplasmic reticulum membrane. J Cell Biol 134:269–278
- Nicchitta CV, Blobel G. 1993. Lumenal proteins of the mammalian endoplasmic reticulum are required to complete protein translocation. Cell 73:989–998
- Nilsson I, Ohvo-Rekila H, Slotte JP, Johnson AE, von Heijne G. 2001. Inhibition of protein translocation across the endoplasmic reticulum membrane by sterols. J Biol Chem 276:41748–41754
- Nishiyama K-i, Suzuki T, Tokuda H. 1996. Inversion of the membrane topology of SecG coupled with SecA-dependent preprotein translocation. Cell 85:71–81
- Nishiyama K, Hanada M, Tokuda H. 1994. Disruption of the gene encoding p12 (SecG) reveals the direct involvement and important function of SecG in the protein translocation of Escherichia coli at low temperature. EMBO J 13:3272–3277
- Nishiyama K, Maeda M, Yanagisawa K, Nagase R, Komura H, Iwashita T, et al. 2012. MPIase is a glycolipozyme essential for membrane protein integration. Nat Commun 3:1260
- Nohara T, Nakai M, Goto A, Endo T. 1995. Isolation and characterization of the cDNA for pea chloroplast SecA. Evolutionary conservation of the bacterial-type SecA-dependent protein transport within chloroplasts. FEBS Lett 364:305–308
- Nothaft H, Szymanski CM. 2010. Protein glycosylation in bacteria: Sweeter than ever. Nat Rev Miocobiol 8:765–778
- Nouwen N, de Kruijff B, Tommassen J. 1996. ΔμH+ dependency of in vitro protein translocation into Escherichia coli inner-membrane vesicles varies with the signal-sequence core-region composition. Mol Microbiol 19:1205–1214
- Nouwen N, Driessen AJM. 2002. SecDFyajC forms a heterotetrameric complex with YidC. Mol Microbiol 44:1397–1405
- Nyathi Y, Wilkinson BM, Pool MR. 2013. Co-translational targeting and translocation of proteins to the endoplasmic reticulum. Biochim Biophys Acta 1833:2392–2402
- O’Neil K, DeGrado WF. 1990. How calmodulin binds its targets: Sequence independent recognition of amphiphilic alpha-helices. Trends Biochem Sci 15:59–64
- Ogg SC, Poritz MA, Walter P. 1992. Signal recognition particle receptor is important for cell growth and protein secretion in Saccharomyces cerevisiae. Mol Cell Biol 3:895–911
- Ogg SC, Walter P. 1995. SRP samples nascent chains for the presence of signal sequences by interacting with ribosomes at a discrete step during translation elongation. Cell 81:1075–1084
- Oh E, Becker AH, Sandikci A, Nichols RJ, Typas A, Gross CA, et al. 2011. Selective ribosome profiling reveals the cotranslational chaperone action of trigger factor in vivo. Cell 147:1295–1308
- Öjemalm K, Botelho SC, Stüdle C, von Heijne G. 2013. Quantitative analysis of SecYEG-mediated insertion of transmembrane α-helices into the bacterial inner membrane. J Mol Biol 425:2813–2822
- Öjemalm K, Halling KK, Nilsson I, von Heijne G. 2012. Orientational preferences of neighboring helices can drive ER insertion of a marginally hydrophobic transmembrane helix. Mol Cell 45:529–540
- Oliver DB, Beckwith J. 1981. E. coli mutant pleiotropically defective in the export of secreted proteins. Cell 25:765–772
- Osborne AR, Rapoport TA. 2007. Protein translocation is mediated by oligomers of the SecY complex with one SecY copy forming the channel. Cell 129:97–110
- Osborne R, Silhavy TJ. 1993. PrlA suppressor mutations cluster in regions corresponding to three distinct topological domains. EMBO J 12:3391–3398
- Paetzel M, Dalbey RE, Strynadka NCJ. 1998. Crystal structure of a bacterial signal peptidase in complex with a [beta]-lactam inhibitor. Nature 396:186–190
- Palacios IM. 2007. How does an mRNA find its way? Intracellular localisation of transcripts. Semin Cell Develop Biol 18:163–170
- Palmer T, Berks BC. 2012. The twin-arginine translocation (Tat) protein export pathway. Nat Rev Miocobiol 10:483–496
- Panzner S, Dreier L, Hartmann E, Kostka S, Rapoport TA. 1995. Posttranslational protein transport in yeast reconstituted with a purified complex of Sec proteins and Kar2p. Cell 81:561–570
- Papanikou E, Karamanou S, Economou A. 2007. Bacterial protein secretion through the translocase nanomachine. Nat Rev Miocobiol 5:839–851
- Park E, Ménétret J-F, Gumbart JC, Ludtke SJ, Li W, Whynot A, et al. 2014. Structure of the SecY channel during initiation of protein translocation. Nature 506:102–106
- Park E, Rapoport TA. 2012. Mechanisms of Sec61/SecY-mediated protein translocation across membranes. Annu Rev Biophys 41:21–40
- Parlitz R, Eitan A, Stjepanovic G, Bahari L, Bange G, Bibi E, Sinning I. 2007. Escherichia coli signal recognition particle receptor FtsY contains an essential and autonomous membrane-binding amphipathic helix. J Biol Chem 282:32176–32184
- Pfeffer S, Brandt F, Hrabe T, Lang S, Eibauer M, Zimmermann R, Förster F. 2012. Structure and 3D arrangement of endoplasmic reticulum membrane-associated ribosomes. Structure 20:1508–1518
- Pfeffer S, Dudek J, Gogala M, Schorr S, Linxweiler J, Lang S, et al. 2014. Structure of the mammalian oligosaccharyl-transferase complex in the native ER protein translocon. Nat Commun 5:3072
- Plath K, Mothes W, Wilkinson BM, Stirling CJ, Rapoport TA. 1998. Signal sequence recognition in posttranslational protein transport across the yeast ER membrane. Cell 94:795–807
- Plath K, Wilkinson BM, Stirling CJ, Rapoport TA. 2004. Interactions between Sec complex and prepro-alpha-factor during posttranslational protein transport into the endoplasmic reticulum. Mol Cell Biol 15:1–10
- Pogliano JA, Beckwith J. 1994. SecD and SecF facilitate protein export in Escherichia coli. EMBO J 13:554–561
- Pohlschröder M, Hartmann E, Hand NJ, Dilks K, Haddad A. 2005. Diversity and evolution of protein translocation. Annu Rev Microbiol 59:91–111
- Pool MR. 2009. A trans-membrane segment inside the ribosome exit tunnel triggers RAMP4 recruitment to the Sec61p translocase. J Cell Biol 185:889–902
- Poritz M, Bernstein H, Strub K, Zopf D, Wilhelm H, Walter P. 1990. An E. coli ribonucleoprotein containing 4.5S RNA resembles mammalian signal recognition particle. Science 250:1111–1117
- Poritz MA, Siegel V, Hansen W, Walter P. 1988a. Small ribonucleoproteins in Schizosaccharomyces pombe and Yarrowia lipolytica homologous to signal recognition particle. Proc Natl Acad Sci USA 85:4315–4319
- Poritz MA, Strub K, Walter P. 1988b. Human SRP RNA and E. coli 4.5S RNA contain a highly homologous structural domain. Cell 55:4–6
- Potter MD, Nicchitta CV. 2000. Regulation of ribosome detachment from the mammalian endoplasmic reticulum membrane. J Biol Chem 275:33828–33835
- Potter MD, Nicchitta CV. 2002. Endoplasmic reticulum-bound ribosomes reside in stable association with the translocon following termination of protein synthesis. J Biol Chem 277:23314–23320
- Powers T, Walter P. 1997. Co-translational protein targeting catalyzed by the Escherichia coli signal recognition particle and its receptor. EMBO J 16:4880–4886
- Prilusky J, Bibi E. 2009. Studying membrane proteins through the eyes of the genetic code revealed a strong uracil bias in their coding mRNAs. Proc Natl Acad Sci USA 106:6662–6666
- Prinz A, Behrens C, Rapoport TA, Hartmann E, Kalies K-UU. 2000. Evolutionarily conserved binding of ribosomes to the translocation channel via the large ribosomal RNA. EMBO J 19:1900–1906
- Raden D, Song W, Gilmore R. 2000. Role of the cytoplasmic segments of Sec61α in the ribosome-binding and translocation-promoting activities of the Sec61 complex. J Cell Biol 150:53–64
- Raetz CR. 1978. Enzymology, genetics, and regulation of membrane phospholipid synthesis in Escherichia coli. Microbiol Rev 42:614–659
- Randall LL, Hardy SJS. 1977. Synthesis of exported proteins by membrane-bound polysomes from Escherichia coli. Eur J Biochem 75:43–53
- Randall LL, Topping TB, Smith VF, Diamond DL, Hardy SJ 1998. SecB: A chaperone from Escherichia coli. Methods Enzymol 290:444–459
- Rapiejko PJ, Gilmore R. 1997. Empty site forms of the SRP54 and SR alpha GTPases mediate targeting of ribosome-nascent chain complexes to the endoplasmic reticulum. Cell 89:703–713
- Rapp M, Granseth E, Seppälä S, von Heijne G. 2006. Identification and evolution of dual-topology membrane proteins. Nat Struct Mol Biol 13:112–116
- Raue U, Oellerer S, Rospert S. 2007. Association of protein biogenesis factors at the yeast ribosomal tunnel exit is affected by the translational status and nascent polypeptide sequence. J Biol Chem 282:7809–7816
- Reid DW, Nicchitta CV. 2012. Primary role for endoplasmic reticulum-bound ribosomes in cellular translation identified by ribosome profiling. J Biol Chem 287:5518–5527
- Reithinger JH, Kim JEH, Kim H. 2013. Sec62 protein mediates membrane insertion and orientation of moderately hydrophobic signal anchor proteins in the endoplasmic reticulum (ER). J Biol Chem 288:18058–18067
- Rensing SA, Maier UG. 1994. The SecY protein family: Comparative analysis and phylogenetic relationships. Mol Phylogen Evol 3:187–191
- Rietveld AG, Koorengevel MC, de Kruijff B. 1995. Non-bilayer lipids are required for efficient protein transport across the plasma membrane of Escherichia coli. EMBO J 14:5506–5513
- Römisch K, Ribes V, High S, Lütcke H, Tollervey D, Dobberstein B. 1990. Structure and function of signal recognition particle (SRP). Mol Biol Rep 14:71–72
- Sääf A, Andersson H, Gafvelin G, Heijnet Gv. 2009. SecA-dependence of the translocation of a large periplasmic loop in the Escherichia coli MalF inner membrane protein is a function of sequence context. Mol Membr Biol 12:209–215
- Sachelaru I, Petriman NA, Kudva R, Kuhn P, Welte T, Knapp B, et al. 2013. YidC occupies the lateral gate of the SecYEG translocon and is sequentially displaced by a nascent membrane protein. J Biol Chem 288:16295–16307
- Sadlish H, Pitonzo D, Johnson AE, Skach WR. 2005. Sequential triage of transmembrane segments by Sec61alpha during biogenesis of a native multispanning membrane protein. Nat Struct Mol Biol 12:870–878
- Samuelson JC, Jiang F, Yi L, Chen M, de Gier JW, Kuhn A, Dalbey RE. 2001. Function of YidC for the insertion of M13 procoat protein in Escherichia coli: Translocation of mutants that show differences in their membrane potential dependence and Sec requirement. J Biol Chem 276:34847–34852
- Sandikci A, Gloge F, Martinez M, Mayer MP, Wade R, Bukau B, Kramer G. 2013. Dynamic enzyme docking to the ribosome coordinates N-terminal processing with polypeptide folding. Nat Struct Mol Biol 20:843–850
- Satoh Y, Matsumoto G, Mori H, Ito K. 2003. Nearest neighbor analysis of the SecYEG complex. 1. Identification of a SecY-SecG interface. Biochemistry 42:7434–7441
- Saurí A, McCormick PJ, Johnson AE, Mingarro I. 2007. Sec61α and TRAM are sequentially adjacent to a nascent viral membrane protein during its ER integration. J Mol Biol 366:366–374
- Schachter H, Freeze HH. 2009. Glycosylation diseases: Quo vadis? Biochim Biophys Acta 1792:925–930
- Schäfer U, Beck K, Müller M. 1999. Skp, a molecular chaperone of gram-negative bacteria, is required for the formation of soluble periplasmic intermediates of outer membrane proteins. J Biol Chem 274:24567–24574
- Schaffitzel C, Oswald M, Berger I, Ishikawa T, Abrahams JP, Koerten HK, et al. 2006. Structure of the E. coli signal recognition particle bound to a translating ribosome. Nature 444:503–506
- Schaletzky J, Rapoport TA. 2006. Ribosome binding to and dissociation from translocation sites of the endoplasmic reticulum membrane. Mol Cell Biol 17:3860–3869
- Schatz PJ, Beckwith J. 1990. Genetic analysis of protein export in Escherichia coli. Annu Rev Genet 24:215–248
- Schatz PJ, Bieker KL, Ottemann KM, Silhavy TJ, Beckwith J. 1991. One of three transmembrane stretches is sufficient for the functioning of the SecE protein, a membrane component of the E. coli secretion machinery. EMBO J 10:1749–1757
- Schäuble N, Lang S, Jung M, Cappel S, Schorr S, Ulucan Ö, et al. 2012. BiP-mediated closing of the Sec61 channel limits Ca2+ leakage from the ER. EMBO J 31:3282–3296
- Schiebel E, Driessen AJM, Hartl F-U, Wickner W. 1991. µH+ and ATP function at different steps of the catalytic cycle of preprotein translocase. Cell 64:927–939
- Schlenker O, Hendricks A, Sinning I, Wild K. 2006. The structure of the mammalian signal recognition particle (SRP) receptor as prototype for the interaction of small GTPases with Longin domains. J Biol Chem 281:8898–8906
- Schlünzen F, Wilson DN, Tian P, Harms JM, McInnes SJ, Hansen HA, et al. 2005. The binding mode of the trigger factor on the ribosome: implications for protein folding and SRP interaction. Structure 13:1685–1694
- Schröder K, Martoglio B, Hofmann M, Holscher C, Hartmann E, Prehn S, et al. 1999. Control of glycosylation of MHC class II-associated invariant chain by translocon-associated RAMP4. EMBO J 18:4804–4815
- Schwartz T, Blobel G. 2003. Structural basis for the function of the β subunit of the eukaryotic signal recognition particle receptor. Cell 112:793–803
- Schwarz F, Aebi M. 2011. Mechanisms and principles of N-linked protein glycosylation. Curr Opin Struct Biol 21:576–582
- Seitl I, Wickles S, Beckmann R, Kuhn A, Kiefer D. 2013. The C-terminal regions of YidC from Rhodopirellula baltica and Oceanicaulis alexandrii bind to ribosomes and partially substitute for SRP receptor function in Escherichia coli. Mol Microbiol 91:408–421
- Shao S, Hegde RS. 2011. Membrane protein insertion at the endoplasmic reticulum. Annu Rev Cell Dev Biol 27:25–56
- Shaw AS, Rottier PJ, Rose JK. 1988. Evidence for the loop model of signal-sequence insertion into the endoplasmic reticulum. Proc Natl Acad Sci USA 85:7592–7596
- Shibatani T, David LL, McCormack AL, Frueh K, Skach WR. 2005. Proteomic analysis of mammalian oligosaccharyltransferase reveals multiple subcomplexes that contain Sec61, TRAP, and two potential new subunits. Biochemistry 44:5982–5992
- Shiozuka K, Tani K, Mizushima S, Tokuda H. 1990. The proton motive force lowers the level of ATP required for the in vitro translocation of a secretory protein in Escherichia coli. J Biol Chem 265:18843–18847
- Siegel V, Walter P. 1985. Elongation arrest is not a prerequisite for secretory protein translocation across the microsomal membrane. J Cell Biol 100:1913–1921
- Siegel V, Walter P. 1986. Removal of the Alu structural domain from signal recognition particle leaves its protein translocation activity intact. Nature 320:81–84
- Skowronek MH, Rotter M, Haas IG. 1999. Molecular characterization of a novel mammalian DnaJ-like Sec63p homolog. Biol Chem 380:1133–1138
- Smith MA, Clemons WM, DeMars CJ, Flower AM. 2005. Modeling the effects of prl mutations on the Escherichia coli SecY complex. J Bacteriol 187:6454–6465
- Sommer N, Junne T, Kalies KU, Spiess M, Hartmann E. 2013. TRAP assists membrane protein topogenesis at the mammalian ER membrane. Biochim Biophys Acta 1833:3104–3111
- Song W, Raden D, Mandon EC, Gilmore R. 2000. Role of Sec61α in the regulated transfer of the ribosome-nascent chain complex from the signal recognition particle to the translocation channel. Cell 100:333–343
- Soromani C, Zeng N, Hollemeyer K, Heinzle E, Klein M-C, Tretter T, et al. 2012. N-acetylation and phosphorylation of Sec complex subunits in the ER membrane. BMC Cell Biol 13:34
- Stephens SB, Dodd RD, Brewer JW, Lager PJ, Keene JD, Nicchitta CV. 2005. Stable ribosome binding to the endoplasmic reticulum enables compartment-specific regulation of mRNA translation. Mol Cell Biol 16:5819–5831
- Sugai R, Takemae K, Tokuda H, Nishiyama K-i. 2007. Topology inversion of SecG is essential for cytosolic SecA-dependent stimulation of protein translocation. J Biol Chem 282:29540–29548
- Tai VW, Imperiali B. 2001. Substrate specificity of the glycosyl donor for oligosaccharyl transferase. J Org Chem 66:6217–6228
- Tamborero S, Vilar M, Martinez-Gil L, Johnson AE, Mingarro I. 2011. Membrane insertion and topology of the translocating chain-associating membrane protein (TRAM). J Mol Biol 406:571–582
- Terzi L, Pool MR, Dobberstein B, Strub K. 2004. Signal recognition particle Alu domain occupies a defined site at the ribosomal subunit interface upon signal sequence recognition. Biochemistry 43:107–117
- Tian H, Beckwith J. 2002. Genetic screen yields mutations in genes encoding all known components of the Escherichia coli signal recognition particle pathway. J Bacteriol 184:111–118
- Tjalsma H, Bolhuis A, van Roosmalen ML, Wiegert T, Schumann W, Broekhuizen CP, et al. 1998. Functional analysis of the secretory precursor processing machinery of Bacillus subtilis: Identification of a eubacterial homolog of archaeal and eukaryotic signal peptidases. Genes Develop 12:2318–2331
- Tomkiewicz D, Nouwen N, Driessen AJM. 2007. Pushing, pulling and trapping – modes of motor protein supported protein translocation. FEBS Lett 581:2820–2828
- Tomkiewicz D, Nouwen N, van Leeuwen R, Tans S, Driessen AJM. 2006. SecA supports a constant rate of preprotein translocation. J Biol Chem 281:15709–15713
- Tong J, Dolezal P, Selkrig J, Crawford S, Simpson AGB, Noinaj N, et al. 2011. Ancestral and derived protein import pathways in the mitochondrion of Reclinomonas americana. Mol Biol Evol 28:1581–1591
- Träger C, Rosenblad MA, Ziehe D, Garcia-Petit C, Schrader L, Kock K, et al. 2012. Evolution from the prokaryotic to the higher plant chloroplast signal recognition particle: The signal recognition particle RNA is conserved in plastids of a wide range of photosynthetic organisms. Plant Cell 24:4819–4836
- Tseng TT, Gratwick KS, Kollman J, Park D, Nies DH, Goffeau A, Saier MH. 1999. The RND permease superfamily: An ancient, ubiquitous and diverse family that includes human disease and development proteins. J Mol MicroBio Biotechnol 1:107–125
- Tsukazaki T, Mori H, Echizen Y, Ishitani R, Fukai S, Tanaka T, et al. 2011. Structure and function of a membrane component SecDF that enhances protein export. Nature 474:235–238
- Tsukazaki T, Mori H, Fukai S, Ishitani R, Mori T, Dohmae N, et al. 2008. Conformational transition of Sec machinery inferred from bacterial SecYE structures. Nature 455:988–991
- Tyedmers J, Lerner M, Bies C, Dudek J, Skowronek MH, Haas IG, et al. 2000. Homologs of the yeast Sec complex subunits Sec62p and Sec63p are abundant proteins in dog pancreas microsomes. Proc Natl Acad Sci USA 97:7214–7219
- Tyedmers J, Lerner M, Wiedmann M, Volkmer J, Zimmermann R. 2003. Polypeptide-binding proteins mediate completion of co-translational protein translocation into the mammalian endoplasmic reticulum. EMBO Rep 4:505–510
- Tyson JR, Stirling CJ. 2000. LHS1 and SIL1 provide a lumenal function that is essential for protein translocation into the endoplasmic reticulum. EMBO J 19:6440–6452
- Ullers RS, Houben EN, Raine A, ten Hagen-Jongman CM, Ehrenberg M, Brunner J, et al. 2003. Interplay of signal recognition particle and trigger factor at L23 near the nascent chain exit site on the Escherichia coli ribosome. J Cell Biol 161:679–684
- Urbanus ML, Scotti PA, Froderberg L, Saaf A, de Gier JW, Brunner J, et al. 2001. Sec-dependent membrane protein insertion: sequential interaction of nascent FtsQ with SecY and YidC. EMBO Rep 2:524–529
- Valencia-Burton M, McCullough RM, Cantor CR, Broude NE. 2007. RNA visualization in live bacterial cells using fluorescent protein complementation. Nat Meth 4:421–427
- Valent Q, Kendall D, High S, Kusters R, Oudega B, Luirink J. 1995. Early events in preprotein recognition in E. coli: interaction of SRP and trigger factor with nascent polypeptides. EMBO J 14:5494–5505
- Valent QA, Scotti PA, High S, de Gier JW, von Heijne G, Lentzen G, et al. 1998. The Escherichia coli SRP and SecB targeting pathways converge at the translocon. EMBO J 17:2504–2512
- van Bloois E, Dekker HL, Froderberg L, Houben EN, Urbanus ML, de Koster CG, et al. 2008. Detection of cross-links between FtsH, YidC, HflK/C suggests a linked role for these proteins in quality control upon insertion of bacterial inner membrane proteins. FEBS Lett 582:1419–1424
- van Dalen A, de Kruijff B. 2004. The role of lipids in membrane insertion and translocation of bacterial proteins. Biochim Biophys Acta – Mol Cell Res 1694:97–109
- Van den Berg B, Clemons WM, Collinson I, Modis Y, Hartmann E, Harrison SC, Rapoport TA. 2004. X-ray structure of a protein-conducting channel. Nature 427:36–44
- van der Does C, Swaving J, van Klompenburg W, Driessen AJM. 2000. Non-bilayer lipids stimulate the activity of the reconstituted bacterial protein translocase. J Biol Chem 275:2472–2478
- van der Laan M, Nouwen N, Driessen AJM. 2004. SecYEG proteoliposomes catalyze the Deltaphi-dependent membrane insertion of FtsQ. J Biol Chem 279:1659–1664
- van der Sluis EO, Driessen AJM. 2006. Stepwise evolution of the Sec machinery in proteobacteria. Trends Microbiol 14:105–108
- van der Sluis EO, Nouwen N, Driessen AJM. 2002. SecY–SecY and SecY–SecG contacts revealed by site-specific crosslinking. FEBS Lett 527:159–165
- van der Sluis EO, van der Vries E, Berrelkamp G, Nouwen N, Driessen AJM. 2006. Topologically fixed SecG is fully functional. J Bacteriol 188:1188–1190
- van Meer G, Voelker DR, Feigenson GW. 2008. Membrane lipids: Where they are and how they behave. Nat Rev Mol Cell biol 9:112–124
- VanValkenburgh C, Chen X, Mullins C, Fang H, Green N. 1999. The catalytic mechanism of endoplasmic reticulum signal peptidase appears to be distinct from most eubacterial signal peptidases. J Biol Chem 274:11519–11525
- Vassylyev DG, Mori H, Vassylyeva MN, Tsukazaki T, Kimura Y, Tahirov TH, Ito K. 2006. Crystal structure of the translocation ATPase SecA from Thermus thermophilus reveals a parallel, head-to-head dimer. J Mol Biol 364:248–258
- Vitrac H, Bogdanov M, Heacock P, Dowhan W. 2011. Lipids and topological rules of membrane protein assembly: balance between long and short range lipid-protein interactions. J Biol Chem 286:15182–15194
- von Heijne G. 1984. Analysis of the distribution of charged residues in the N-terminal region of signal sequences: Implications for protein export in prokaryotic and eukaryotic cells. EMBO J 3:2315–2318
- von Heijne G. 1985. Signal sequences. The limits of variation. J Mol Biol 184:99–105
- von Heijne G. 1986. A new method for predicting signal sequence cleavage sites. Nucleic Acids Res 14:4683–4690
- von Heijne G. 1990. The signal peptide. J Membr Biol 115:195–201
- von Heijne G, Gavel Y. 1988. Topogenic signals in integral membrane proteins. Eur J Biochem 174:671–678
- Voss M, Schröder B, Fluhrer R. 2013. Mechanism, specificity, and physiology of signal peptide peptidase (SPP) and SPP-like proteases. Biochim Biophys Acta – Biomembr 1828:2828–2839
- Walter P, Blobel G. 1980. Purification of a membrane-associated protein complex required for protein translocation across the endoplasmic reticulum. Proc Natl Acad Sci USA 77:7112–7116
- Walter P, Blobel G. 1981. Translocation of proteins across the endoplasmic reticulum III. Signal recognition protein (SRP) causes signal sequence-dependent and site-specific arrest of chain elongation that is released by microsomal membranes. J Cell Biol 91:557–561
- Walter P, Blobel G. 1982. Signal recognition particle contains a 7S RNA essential for protein translocation across the endoplasmic reticulum. Nature 299:691–698
- Walter P, Ibrahimi I, Blobel G. 1981. Translocation of proteins across the endoplasmic reticulum. I. Signal recognition protein (SRP) binds to in-vitro-assembled polysomes synthesizing secretory protein. J Cell Biol 91:545–550
- Wang L, Dobberstein B. 1999. Oligomeric complexes involved in translocation of proteins across the membrane of the endoplasmic reticulum. FEBS Lett 457:316–322
- Wang P, Shim E, Cravatt B, Jacobsen R, Schoeniger J, Kim AC, et al. 2008. Escherichia coli signal peptide peptidase A is a serine-lysine protease with a lysine recruited to the nonconserved amino-terminal domain in the S49 protease family. Biochemistry 47:6361–6369
- Watanabe M, Blobel G. 1989. SecB functions as a cytosolic signal recognition factor for protein export in E. coli. Cell 58:695–705
- Weiche B, Bürk J, Angelini S, Schiltz E, Thumfart JO, Koch HG. 2008. A cleavable N-terminal membrane anchor is involved in membrane binding of the Escherichia coli SRP receptor. J Mol Biol 377:761–773
- Welte T, Kudva R, Kuhn P, Sturm L, Braig D, Müller M, et al. 2012. Promiscuous targeting of polytopic membrane proteins to SecYEG or YidC by the Escherichia coli signal recognition particle. Mol Cell Biol 23:464–479
- Whitehouse S, Gold VAM, Robson A, Allen WJ, Sessions RB, Collinson I. 2012. Mobility of the SecA 2-helix-finger is not essential for polypeptide translocation via the SecYEG complex. J Cell Biol 199:919–929
- Wild K, Sinning I, Cusack S. 2001. Crystal structure of an early protein-RNA assembly complex of the signal recognition particle. Science 294:598–601
- Wirth A, Jung M, Bies C, Frien M, Tyedmers J, Zimmermann R, Wagner R. 2003. The Sec61p complex is a dynamic precursor activated channel. Mol Cell 12:261–268
- Wittke S, Dunnwald M, Johnsson N. 2000. Sec62p, a component of the endoplasmic reticulum protein translocation machinery, contains multiple binding sites for the Sec-complex. Mol Cell Biol 11:3859–3871
- Wu ZC, de Keyzer J, Kedrov A, Driessen AJM. 2012. Competitive binding of the SecA ATPase and ribosomes to the SecYEG translocon. J Biol Chem 287:7885–7895
- Xing L, Bassell GJ. 2013. mRNA localization: An orchestration of assembly, traffic and synthesis. Traffic 14:2–14
- Xu Z, Knafels JD, Yoshino K. 2000. Crystal structure of the bacterial protein export chaperone SecB. Nat Struct Mol Biol 7:1172–1177
- Yamaguchi A, Hori O, Stern DM, Hartmann E, Ogawa S, Tohyama M. 1999. Stress-associated endoplasmic reticulum protein 1 (Serp1)/ribosome-associated membrane protein 4 (Ramp4) stabilizes membrane proteins during stress and facilitates subsequent glycosylation. J Cell Biol 147:1195–1204
- Yamamoto H, Fujita H, Kida Y, Sakaguchi M. 2012. Pleiotropic effects of membrane cholesterol upon translocation of protein across the endoplasmic reticulum membrane. Biochemistry 51:3596–3605
- Yamamoto H, Kida Y, Sakaguchi M. 2013. Phosphatidylserine-binding protein lactadherin inhibits protein translocation across the ER membrane. Biochem Biophys Res Commun 434:620–626
- Young BP. 2001. Sec63p and Kar2p are required for the translocation of SRP-dependent precursors into the yeast endoplasmic reticulum in vivo. EMBO J 20:262–271
- Yuan J, Zweers J, Dijl J, Dalbey R. 2010. Protein transport across and into cell membranes in bacteria and archaea. Cell Mol Life Sci 67:179–199
- Zafar S, Nasir A, Bokhari H. 2011. Computational analysis reveals abundance of potential glycoproteins in Archaea, Bacteria and Eukarya. Bioinformation 6:352–355
- Zhang X, Rashid R, Wang K, Shan So. 2010. Sequential checkpoints govern substrate selection during cotranslational protein targeting. Science 328:757–760
- Zhang X, Schaffitzel C, Ban N, Shan S-o. 2009a. Multiple conformational switches in a GTPase complex control co-translational protein targeting. Proc Natl Acad Sci USA 106:1754–1759
- Zhang Y-J, Tian H-F, Wen J-F. 2009b. The evolution of YidC/Oxa/Alb3 family in the three domains of life: A phylogenomic analysis. BMC Evol Biol 9:137
- Zhao X, Jäntti J. 2009. Functional characterization of the trans-membrane domain interactions of the Sec61 protein translocation complex beta-subunit. BMC Cell Biol 10:76
- Zheng N, Gierasch LM. 1997. Domain interactions in E. coli SRP: stabilization of M domain by RNA is required for effective signal sequence modulation of NG domain. Mol Cell 1:79–87
- Zhou Y, Xue S, Yang JJ. 2013. Calciomics: Integrative studies of Ca2+-binding proteins and their interactomes in biological systems. Metallomics 5:29–42
- Zimmer J, Nam Y, Rapoport TA. 2008. Structure of a complex of the ATPase SecA and the protein-translocation channel. Nature 455:936–943
- Zimmermann R, Eyrisch S, Ahmad M, Helms V. 2011. Protein translocation across the ER membrane. Biochim Biophys Acta 1808:912–924
- Zimmermann R, Sagstetter M, Lewis M, Pelham HR. 1988. Seventy-kilodalton heat shock proteins and an additional component from reticulocyte lysate stimulate import of M13 procoat protein into microsomes. EMBO J 7:2875–2880
- Zopf D, Bernstein H, Johnson A, Walter P. 1990. The methionine-rich domain of the 54 kd protein subunit of the signal recognition particle contains an RNA binding site and can be crosslinked to a signal sequence. EMBO J 9:4511–4517
- Zopf D, Bernstein HD, Walter P. 1993. GTPase domain of the 54-kD subunit of the mammalian signal recognition particle is required for protein translocation but not for signal sequence binding. J Cell Biol 120:1113–1121
- Zwieb C, Bhuiyan S. 2010. Archaea signal recognition particle shows the way. Archaea 2010:485051
- Zwizinski C, Wickner W. 1980. Purification and characterization of leader (signal) peptidase from Escherichia coli. J Biol Chem 255:7973–7977