Abstract
The deposition of insoluble amyloid fibrils resulting from the aggregation of the human islet amyloid polypeptide (hIAPP) within the islet of Langerhans is a pathological feature of type 2 diabetes mellitus (T2DM). Increasing evidence indicates that biological membranes play a key role in amyloid aggregation, modulating among others the kinetics of amyloid formation, and being the target of toxic species generated during amyloid formation. In T2DM patients, elevated levels of cholesterol, an important determinant of the physical state of biological membranes, are observed in β-cells and are thought to directly impair β-cell function and insulin secretion. However, it is not known whether cholesterol enhances membrane-interaction or membrane-insertion of hIAPP. In this study, we investigated the effect of cholesterol incorporated in zwitterionic and anionic membranes. Our circular dichroism and liquid state NMR data reveal that 10–30% of cholesterol slightly affects the aggregational and conformational behaviour of hIAPP. Additional fluorescence results indicate that 10 and 20% of cholesterol slightly slow down the kinetics of oligomer and fibril formation while anionic lipids accelerate this kinetics. This behavior might be caused by differences in membrane insertion and therefore in membrane binding of hIAPP. The membrane binding affinity was evaluated using 1H NMR experiments and our results show that the affinity of hIAPP for membranes containing cholesterol is significantly smaller than that for membranes containing anionic lipids. Furthermore, we found that hIAPP-induced membrane damage is synchronized to fibril formation in the absence and in the presence of cholesterol.
Introduction
The deposition of amyloid in the islets of Langerhans of the pancreas is a characteristic pathological feature of type 2 diabetes mellitus (T2DM). In 1987, the main component of human islet amyloid was identified as a 37-amino acid residue peptide called human islet amyloid polypeptide (hIAPP) or amylin (Cooper et al., Citation1987; Westermark et al., Citation1987). hIAPP is stored and secreted together with insulin by the pancreatic β-cells and is released in response to the stimuli that lead to insulin secretion (Kahn et al., Citation1990; Lukinius et al., Citation1989; Stridsberg et al., Citation1993). hIAPP plays, in its soluble monomeric form, a role in the regulation of glucose homeostasis, in gastric emptying and in other cellular processes (Akesson et al., Citation2003; Karlsson, Citation1999; Reda et al., Citation2002; Rushing et al., Citation2001). Human IAPP has a strong amyloidogenic propensity that has been linked to insulin-producing β-cells damage, eventually resulting in T2DM. The amyloid deposits also named amyloid fibrils are composed of β-sheet aggregates with a characteristic structure similar to those found in Alzheimer’s disease, Parkinson’s disease, spongiform encephalopathy and a variety of other degenerative diseases (Chiti & Dobson, Citation2006; Selkoe, Citation2003; Sipe, Citation1994). hIAPP amyloid formation is also a key factor in the failure of islet cell transplants and prevention of amyloid formation enhances graft survival (Potter et al., Citation2010; Westermark et al., Citation2008).
Aggregated hIAPP has cytotoxic properties and is believed to be of critical importance for the loss of β-cells in T2DM (Clark et al., Citation1988; Konarkowska et al., Citation2006; Lorenzo et al., Citation1994). The basis of hIAPP-induced cytotoxicity is not completely understood, and various mechanisms have been reported such as receptor-mediated mechanisms, endoplasmic reticulum (ER) stress, permeabilization of the plasma and mitochondria membranes (Brender et al., Citation2012; Cao et al., Citation2013a; Westermark et al., Citation2011). Extensive investigations have been performed focusing on hIAPP-mediated cellular membrane damage (Cao et al., Citation2013b) and several models have been proposed, including the formation of membrane pores similar to ion channels (Anguiano et al., Citation2002; Cao et al., Citation2013c; Demuro et al., Citation2005; Mirzabekov et al., Citation1996) and a membrane disruption caused by the assembly of hIAPP fibrils at the membrane, possibly by forcing the curvature of the bilayer to unfavorable angles, or by the uptake of lipids during fibrillogenesis (Engel et al., Citation2008; Khemtémourian et al., Citation2008; Sparr et al., Citation2004). To gain further insight into the mechanism of cell toxicity, it is important to better understand how the peptide affects the physical properties of the membrane.
Membranes have a heterogeneous composition which varies between species, cell types, and organelles. One major component of cellular membranes is cholesterol, which is required to establish proper membrane permeability and fluidity. Extensive studies have focused on cholesterol-lipid membrane interactions and found that cholesterol increases the order, thickness, and rigidity of the bilayer, which restricts bilayers deformations. Some studies showed that for the peptide Aβ involved in Alzheimer’s disease, the presence of cholesterol in the membranes reduced the membrane disruption and that cholesterol inhibited the insertion of the peptide in lipid membranes (Dante et al., Citation2006; Lau et al., Citation2007). In T2DM patients, elevated levels of cholesterol in β-cells are observed and are thought to directly impair β-cell function and insulin secretion (Brunham et al., Citation2007; Hao & Bogan Citation2009). It is therefore likely that cholesterol, by modulating membrane fluidity and/or membrane curvature, also regulates hIAPP-lipid interactions and hIAPP aggregation. However, most studies on hIAPP-membrane interactions were performed using model membranes containing a much higher percentage of anionic lipids than found in the β-cell membrane (Seeliger et al., 2012) and usually lacking gangliosides and cholesterol. These lipidic components could be important since recent work has argued that gangliosides and cholesterol mediate hIAPP membrane interactions and may play a role in the uptake and clearance of hIAPP (Trikha & Jeremic, Citation2011; Wakabayashi & Matsuzaki, Citation2009). Indeed, it was shown that cholesterol inhibits phospholipid catalysis of amylin aggregation in solution and on planar membranes (Cho et al., Citation2008, Citation2009; Trikha & Jeremic, Citation2011). These studies indicate that the incorporation of 20% of cholesterol in anionic and neutral membranes has dual effects on amyloid aggregation; it decreases overall amylin accumulation, whereas it facilitates formation of dense 200–500 nm protein clusters on planar membranes. However, it is not known whether cholesterol enhances membrane-interaction and membrane-insertion of hIAPP.
In view of these results and observations, we conducted a thorough investigation of hIAPP in neutral membranes containing 0, 10, 20 or 30% of cholesterol and in anionic membranes containing 20% of cholesterol. Using circular dichroism and liquid state NMR, we examined the kinetics of initial events corresponding to hIAPP conformational changes and depletion of the monomeric form. Then, we explored the kinetics of hIAPP-fibril formation in relation to the kinetics of hIAPP-membrane damage. Finally, we further characterized the contribution of cholesterol on hIAPP binding to membranes by liquid state NMR. Our study revealed that: (i) The presence of cholesterol slightly affects the aggregational and conformational behavior of hIAPP, (ii) the processes of fibril formation and membrane damage are still related in the presence of cholesterol, and (iii) the affinity of hIAPP for cholesterol is significantly smaller than that for anionic lipids,
Methods
Materials
Synthetic hIAPP with an amidated C-terminus and disulfide bridge was obtained from Bachem AG (batch number 2500067). The calculated mass (3903.3 Da) of the peptide was confirmed by mass spectrometry. Its amino acid sequence is: KCNTATCATQRLANFLVHSSNNFGAILSSTNVGSNTY. 1,2-dioleoyl-sn-glycero-3-phosphocholine (DOPC) and 1,2-dioleoyl-sn-glycero-3-phospho-L-serine (DOPS) obtained from Avanti Polar Lipids (Alabaster, AL) and cholesterol obtained from Genzyme (Switzerland) were used without further purification. Thioflavin T (ThT) was obtained from Sigma.
Preparation of peptide samples
Peptide was dissolved at a concentration of 1 mM in hexafluoro-isopropanol (HFIP) and incubated for at least 1 h. HFIP was then evaporated and the sample was submitted to vacuum desiccation for at least 30 min. For ThT-fluorescence, membrane leakage and microscopy experiments, the peptide film was dissolved in DMSO to a final peptide concentration of 0.2 mM. The same concentration of DMSO (2.5% in the final volume) was used in all these experiments which enabled us to directly compare the shapes of the curves and the lag times. Instead, for both CD and NMR experiments, no DMSO was used, the resulting peptide film was directly solubilized by addition of a dispersion of unilamellar vesicles.
Preparation of unilamellar vesicles
Each lipid system (PC:Chol 10:0, 9:1, 8:2 and 7:3 and PC/PS/Chol 8:2:0 and 56:20:24, molar ratio) was prepared by dissolving the desired lipids in chloroform in a glass tube. The solvent was evaporated with dry nitrogen gas yielding a lipid film that was subsequently kept in a vacuum desiccator for 30 min. Lipid films were hydrated during at least 30 min in 50 mM Tris/HCl, 200 mM NaCl, pH 7.4 for the fluorescence and microscopy experiments and in 10 mM potassium phosphate buffer, pH 7.4 for the CD and NMR experiments. The lipid suspensions were subjected to 10 freeze-thaw cycles, at temperatures of approximately −190 °C and 50 °C, respectively, and subsequently extruded 19 times through a mini-extruder (Avanti, Alabaster, AL) equipped with polycarbonate membranes (50 and 200 nm cut-off). Calcein-containing vesicles were made using the same protocol, except for the following adaptations. The buffer for hydration of the lipid films was replaced by a solution containing 70 mM calcein and 50 mM Tris (pH 7.4). Free calcein was separated from the calcein-filled unilamellar vesicles using size-exclusion chromatography (Sephadex G50-fine) and elution with 50 mM Tris-HCl, 200 mM NaCl (pH 7.4). The phospholipid content of lipid stock solutions and vesicle preparations was determined by assessing inorganic phosphate content according to Rouser et al., (Citation1970).
CD spectroscopy
CD spectra were measured on a Jasco 815 spectropolarimeter (Jasco Inc., Easton, MD) over the wavelength range 190–260 nm, by using 0.1 cm path length quartz cell (internal volume 200 µl) from Hellma GmbH. CD spectra were recorded at 25 °C, at 0.2 nm intervals and 10 nm.min−1 scan speed. Measurements were carried out in 10 mM potassium phosphate buffer at pH 7.4. Peptide concentrations were 20 μM in the presence of lipids (peptide:lipid ratio 1:10). To follow hIAPP conformational changes, spectra were recorded at regular intervals and the ellipticity at 200 nm was plotted vs. the time of incubation. Experimental values were fitted to a Boltzmann sigmoidal equation:
In this equation, θi and θf are the initial and final ellipticity values at 200 nm, t0.5 is the time corresponding to 50% of transition conformational and dt a time constant. To check reproducibility, the CD experiments were performed three times on different days using different hIAPP stock solutions and different vesicle preparations.
NMR spectroscopy
Measurements were carried out in 10 mM potassium phosphate buffer in D2O at pH 7.4. Peptide concentrations were 50 μM in the presence of unilamellar vesicles of 50 nm diameter (peptide:lipid ratio 1:10). The peptide film was dissolved in phosphate buffer or vesicles solution and immediately transferred in a Shigemi tube of 5 mm diameter. Experiments were recorded at 25 °C on a Bruker AVANCE III NMR spectrometer operating at a 1H frequency of 500 MHz, equipped with a TCI cryoprobe. One-dimensional spectra were acquired over 8192 points using a spectral width of ∼ 6000 Hz. A cosine apodization window has been applied to all 1H spectra prior to Fourier transform. For each spectrum, 256 scans were accumulated leading to an experimental time of about 12 min. Residual solvent signal was suppressed by weak presaturation during the relaxation delay (2 s). The evolution in time of the peptide signal intensity for each lipid system was followed by recording one-dimensional 1H spectra until the peptide signal intensity decayed completely. Peptide 1H resonances (in the amide/aromatic region between 6.6 and 7.7 ppm, where no lipid signals are expected) were then integrated and these values are plotted as a function of time (see ), leading to a sigmoidal curve, that can be fitted by a modified Richards equation (Sani et al., Citation2011):
where I is peptide intensity obtained from an 1H NMR experiment, Ii and If are the initial and final 1H NMR intensities, ai and af are the slopes before and after the oligomerization phase, t0.5 is the time when the 1H NMR intensity reaches 50% of the maximum 1H NMR signal. Since the analysis is done for the integrated intensity of peptide 1H resonances between 6.6 and 7.7 ppm, results are therefore average values. All raw NMR experimental data have been processed using the TopSpin program (Bruker) and further analyzed for the integration and the fit using the modified Richards equation with a home-written program based on Mathematica 8. The program is available upon request.
Figure 1. (A) CD kinetic study in DOPC vesicles. Plot color code: dark blue: CD spectrum recorded after 5 minutes, purple: CD spectrum recorded after 9 hours, green: CD spectrum recorded after 11 hours. (B) Time course of CD ellipticity at 200 nm. (C) Time evolution of the 1D 1H NMR spectra of hIAPP. Plot color code: dark blue: NMR spectrum recorded after 20 minutes; purple: NMR spectrum recorded after 4 hours; orange: NMR spectrum recorded after 8 hours; green: NMR spectrum recorded after 12 hours. (D) Time course of normalized integrals of NMR signal between 6.6 and 7.7 ppm. “This Figure is reproduced in color in the online version of Molecular Membrane Biology”.
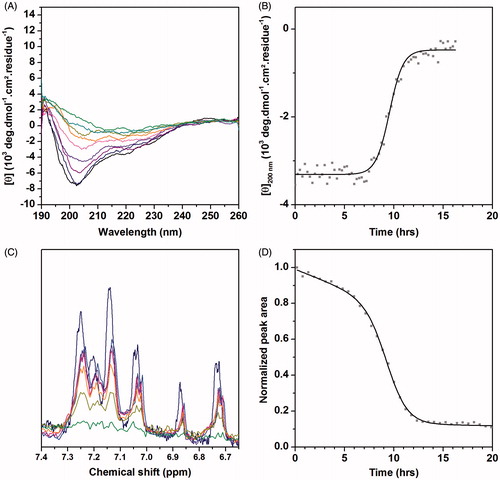
Thioflavin fluorescence assays
The kinetics of hIAPP fibril formation were measured by following the fluorescence intensity increase upon binding of the fluorescent dye ThT to fibrils. A plate reader (Fluostar Optima, Bmg Labtech) and standard 96-well flat-bottom black microtiter plates in combination with a 440 nm excitation filter and a 485 nm emission filter were used. The ThT assay was started by adding 10 μl of an aqueous solution of 0.2 mM hIAPP to 190 μl of a mixture of 10 μM ThT, vesicles (100 µM lipids; peptide:lipid ratio 1:10) and 50 mM Tris/HCl, 200 mM NaCl (pH 7.4). The microtiter plate was shaken for 10 sec directly after addition of all components, but not during the measurements. The ThT assay was performed three times, each in triplicate. The results presented here are the average of the different experiments; errors bars indicate the statistical dispersions.
Electron microscopy
Peptides and vesicles were incubated under the same conditions as in the Thioflavin T assay. Aliquots (25 µl) of this mixture were adsorbed onto glow-discharged carbon-coated 300-mesh copper grids for 2 min. Grids were then blotted and dried. Grids were negatively stained for 45 sec on 2% uranyl acetate, blotted and dried. Grids were examined using a ZEISS 912 Omega electron microscope operating at 80 kV.
Calcein permeability assay in large unilamellar vesicles
A plate reader (Fluostar Optima, Bmg Labtech) was used to perform calcein leakage experiments in standard 96-well transparent microtiter plates. Measurements were conducted on calcein-loaded DOPC, DOPC/Chol 9:1, DOPC/Chol 8:2, DOPC/Chol 7:3, DOPC/DOPS 8:2 and DOPC/DOPS/Chol 56:20:24. The hIAPP peptide was added to a mixture of calcein-containing vesicles in 50 mM Tris/HCl, 200 mM NaCl, pH 7.4. The final concentrations were 100 µM for lipids and 10 µM for peptide (peptide:lipid ratio of 1:10). Directly after addition of all components, the microtiter plate was shaken for 10 sec using the shaking function of the plate reader. The plate was not shaken during the measurements. Fluorescence was measured from the bottom, every minute, using a 485 nm excitation filter and a 520 nm emission filter. The temperature was approximately 28 °C ± 3 °C. The maximum leakage at the end of each measurement was determined via addition of 1 µl of 10% Triton-X100 to a final concentration of 0.05% (v/v). The release of fluorescent dye was normalized according to the following equation:
In this equation, LT is the fraction of dye released (normalized membrane leakage), FT is the measured fluorescence intensity, and F0 and F100 are the fluorescence intensities at time zero and after addition of Triton-X100, respectively. The calcein leakage experiment was performed three times, each in triplicate, on different days. The results presented here are the average of the different experiments and the errors bars indicate the statistical dispersions.
NMR titration on unilamellar vesicles
Peptide film was dissolved in 10 mM potassium phosphate buffer, pH 7.4, with 10% of D2O, to obtain a final concentration of 50 µM. The solution (280 μl) was immediately transferred in a Shigemi tube of 5 mm diameter. The peptide was titrated by unilamellar vesicles of 200 nm diameter composed of DOPC, DOPC/Chol 9:1, DOPC/Chol 8:2, DOPC/Chol 7:3, DOPC/DOPS 8:2 and DOPC/DOPS/Chol 56:20:24. Small quantities of a concentrated stock of vesicles (concentrations between 50 and 70 mM) were added to obtain peptide:lipid ratios of 1:1, 1:5, 1:10, 1:20, 1:50, 1:100, 1:200, and 1:400. Experiments were recorded at 25 °C on a Bruker AVANCE III NMR spectrometer operating at a 1H frequency of 500 MHz, equipped with a TCI cryoprobe. One-dimensional spectra were acquired over 4096 points using a spectral width of 7000 Hz. Solvent resonance was suppressed by a WATERGATE (Liu et al., Citation1998) sequence using pulsed field gradients. For each spectrum, 128 scans were accumulated leading to an experiment time of about 3 min. A cosine apodization window has been applied to all 1H spectra prior to Fourier transform. The decrease of the integral of the 1H resonances as a function of the increase of the peptide:lipid ratio yields the apparent affinity constant Kd. The integral of the 1H resonances between 6.6 and 7.7 ppm was fitted using the following one site binding equation:
where Iobs is the observed integral, Imax the maximum integral (corresponding to the free peptide), P0 the peptide concentration and x represents the ratio between the lipid concentration and peptide concentration, respectively. Since we analyze the evolution of the integral as a function of the peptide:lipid ratio, the results are average values obtained from different NMR signals and the error represents the standard deviation (SD).
Results
Cholesterol exerts a divergent, concentration-dependent effect on the conformational behaviour of hIAPP
Previous studies have shown that upon interaction with zwitterionic membranes hIAPP adopts a random coil conformation, which in time evolves toward a β-sheet conformation (Jayasinghe & Langen, Citation2005; Knight et al., Citation2006). To evaluate the initial peptide structure and to analyze the conformational changes of the peptide after a few hours of incubation in the absence and in the presence of cholesterol, we first performed CD measurements. Immediately upon addition of hIAPP to pure DOPC vesicles, the CD spectrum displays negative ellipticity at 200 nm that is characteristic of a random coil conformation, corresponding to the non-amyloidogenic conformation of monomeric IAPP or small soluble oligomers (). The same result is observed in DOPC vesicles containing 10, 20 and 30% of cholesterol, suggesting that cholesterol does not modulate the initial peptide secondary structure. After few hours of incubation, hIAPP adopts a β-sheet structure, indicative of amyloid fibril formation. The plot of the ellipticity value at 200 nm against time gives a sigmoid curve, in agreement with previous reports (Bartolini et al., Citation2007) (). The time required to reach half of the maximum CD signal at 200 nm (t0.5) is reported in . The random coil to β-sheet transition occurs at 10 ± 2 h in the absence of cholesterol, ∼11–12 ± 4 h in the presence of 10 and 20% of cholesterol, while the transition is more rapid (∼ 7 ± 3 h) in the presence of 30% cholesterol. Our results indicate that cholesterol either slightly accelerates or slows down the random coil to β-sheet transition, depending on its concentration.
Table 1. Kinetic parameters of hIAPP β-sheet formation and monomer depletion in the different vesicles, determined using the time course of absolute CD ellipticity at 200 nm and by 1H NMR, respectively.
Further liquid state 1H NMR studies, using the same experimental conditions as for CD (in terms of temperature, buffer composition, and peptide:lipid ratio), were performed to measure the kinetics of depletion of monomeric, soluble hIAPP. shows the amide/aromatic region of the 1D 1H NMR spectra recorded at 298 K in the presence of DOPC vesicles. Large oligomeric hIAPP species in solution or hIAPP species binding to membranes tumble slowly on the NMR time-scale and will give rise to very broad NMR signals which cannot be detected by liquid state NMR experiments. Spectra revealed a significant loss of signal intensity (∼ 50%) over a period of 8 h suggesting that hIAPP peptide undergoes oligomerization either in its free form or in its bound form with the vesicles. After 12 h, the peptide 1H signals decay to zero indicating that the monomeric peptide form in solution disappears completely. shows the integral of the 1H NMR signals between 6.6 and 7.7 ppm, corresponding to the amide/aromatic signals of the monomeric peptide, plotted against time. Interestingly, the curve can be divided into three distinct regions that match the time evolution of CD ellipticity (). In the first phase, a small linear decrease of the NMR signal is observed without any evolution of CD ellipticity at 200 nm. This behavior may be ascribed to the aggregation of hIAPP monomers into small unstructured oligomeric species that are NMR invisible. Then the sharp drop in NMR intensities suggests that the monomer and small oligomers evolve into bigger oligomers and protofibrils by nucleation and elongation-dependent pathways; finally, the last region corresponds to the spontaneous process of nuclei polymerization or fibril growth, most monomers being recruited in high molecular weight species (). This curve was fitted by a modified Richards logistic function (Sani et al., Citation2011). The time required to reach half of the maximum monomer signal (t0.5) is reported in for each lipid:cholesterol ratio. Our data indicate that, in the presence of DOPC, 50% of hIAPP signal was lost after 9.2 ± 0.2 h. The presence of cholesterol affects this kinetics, with t0.5 values of 6.2 ± 0.3, 9.8 ± 0.1 and 5.1 ± 0.1 h in the presence of 10%, 20% and 30% cholesterol, respectively. Unexpectedly, the kinetics seems to be accelerated in the presence of 10% cholesterol compared to 0% and 20% cholesterol. However, the time needed to observe a complete disappearance of NMR signals lies within the same range for 0, 10 and 20% of cholesterol, (12, 14 and 11 h, respectively). In contrast, the presence of 30% cholesterol significantly increases the rate of monomeric depletion (t0.5 = 5.1 h) and decreases the time of signal disappearance (6 h), which is in agreement with CD results.
To analyze whether charged lipids modulate this effect of cholesterol, the same studies were performed on anionic membranes. It was previously shown that upon interaction with membranes containing negatively charged lipids, hIAPP adopts an α-helical conformation, which in time undergoes a transition to β-sheet (Jayasinghe & Langen, Citation2005). The CD spectrum of hIAPP in DOPC/DOPS (8:2) vesicles confirms such behavior (). The α-helical to β-sheet transition occurs after 1.4 h (). Similar results were observed in DOPC/DOPS/Chol (56:20:24) vesicles with a transition time of approximately 1.6 h (). Thus, our results provide evidence that the presence of cholesterol does not have any significant kinetic effect on the conformational transition of hIAPP in anionic lipid membranes. The proportion of secondary structure elements () is not affected either.
Figure 2. (A) CD spectra of hIAPP in the presence of DOPC/DOPS 8:2 and DOPC/DOPS/Chol 56:20:24 vesicles, t0.5 values are indicated in the insert. (B) Secondary structure content inferred from deconvolution of CD spectra at initial time and after 2 hours. “This Figure is reproduced in color in the online version of Molecular Membrane Biology”.
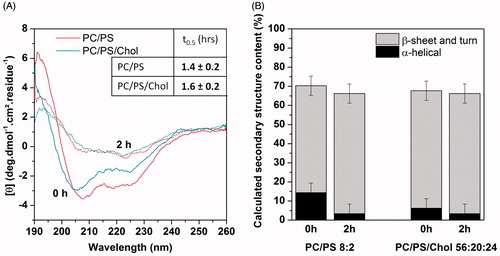
Liquid state NMR spectra of hIAPP were also recorded in the presence of anionic lipids and cholesterol. The 1H NMR spectra of hIAPP freshly incubated in membranes of DOPC/DOPS and DOPC/DOPS/Chol vesicles revealed broad peaks with very low intensity (data not shown). This observation could be explained either by the rather fast kinetics of hIAPP-fibril formation in the presence of negatively charged lipids leading to a lifetime of the monomeric hIAPP which is too short to be observed by liquid state NMR, or by the higher affinity of hIAPP for membranes containing anionic lipids (see below), which lead to large hIAPP-membrane complexes invisible by liquid state NMR.
Altogether, our results demonstrate that 10 and 20% levels of cholesterol do not strongly affect the oligomer formation while 30% of cholesterol seems to accelerate the oligomer formation. We next examined the kinetics of fibril formation and the morphology of fibrils in the absence and in the presence of cholesterol.
Cholesterol affects to some extent the kinetics but not the morphology of hIAPP fibrils
Time-dependent spectroscopic measurements of the increase in ThT fluorescence intensity upon binding to hIAPP fibrils were carried out to follow the fibrillation kinetics of 10 μM hIAPP in the absence and in the presence of cholesterol. The aggregation of hIAPP can be described by a sigmoidal transition with a well-defined lag phase in which monomers and oligomers dominate the population, followed by a growth phase during which fibrils elongate and, finally, a plateau. displays the ThT fluorescence curves for hIAPP in the presence of increasing concentrations of cholesterol. In the absence of cholesterol, the transition from monomer/small soluble oligomers to fibril formation occurs at 25 ± 5 h. In the presence of cholesterol, our results reveal a quite similar profile of kinetics of hIAPP-fibril formation with lag times of 26 ± 4 h, 20 ± 2 h and 21 ± 2 h for the vesicles containing, respectively, 10, 20 and 30% of cholesterol, suggesting that the cholesterol does not significantly affect the kinetics of fibril formation. Previous results indicated that the presence of negatively charged lipids accelerates the rate of hIAPP-fibril formation (Caillon et al., Citation2013; Knight and Miranker Citation2004), which led us to study the hIAPP-fibril formation in the presence of anionic lipid and cholesterol. As expected, our data indicate that anionic membranes do accelerate the hIAPP-fibril formation. In addition, these results show that cholesterol slightly affects hIAPP-fibril formation with lag times of 7.0 ± 1 h and 5.6 ± 1 h in the absence and in the presence of cholesterol, respectively.
Figure 3. (A) Kinetics of hIAPP fibril formation in the presence of vesicles of different compositions. (B to G) Negatively stained microscopy images of hIAPP after incubation with vesicles of different compositions: (B) DOPC/Chol 10:0, (C) DOPC/Chol 9:1, (D) DOPC/Chol 8:2, (E) DOPC/Chol 7:3, (F) DOPC/DOPS 8:2, (G) DOPC/DOPS/Chol 56:20:24. “This Figure is reproduced in color in the online version of Molecular Membrane Biology”.
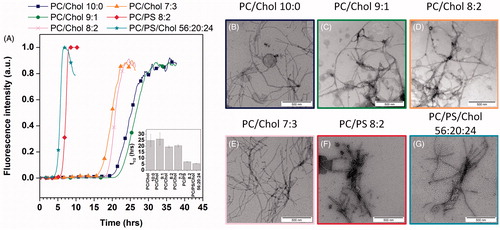
The effect of cholesterol on fibril morphology was analyzed by transmission electron microscopy (TEM). The results are displayed in . shows that hIAPP in DOPC forms fibrils which exhibit the typical morphology of long and twisted amyloid fibrils with widths between 10 and 15 nm. Similar observations are made in both zwitterionic and anionic membranes containing cholesterol suggesting that the net charge of the membrane has no effect on the morphology of the fibrils.
In the presence of cholesterol, hIAPP-induced membrane damage is synchronized with fibril formation
Then, the effect of the peptide on membrane barrier properties was examined under different conditions: In lipid vesicles with and without cholesterol under the same experimental conditions (temperature, buffer, peptide concentration and peptide:lipid ratio) as for fibril formation. Membrane damage was assayed quantitatively by analysing the extent of leakage of a fluorescent dye (calcein) entrapped in unilamellar vesicles, which is an established method to measure membrane damage (Hasper et al., Citation2006). The high concentration of calcein inside intact vesicles leads to self-quenching. Disruption of the membrane of the vesicle by the peptide allows calcein to escape, eliminating the self-quenching effect and therefore increasing the fluorescence of calcein. As shown in , hIAPP induces significant leakage in DOPC vesicles to about 50% of the total vesicle content. The process of leakage is characterized by an S-shaped curve with a lag time of approximately 23 ± 1 h. In the presence of 10, 20 and 30% of cholesterol, a qualitatively similar S-shaped curve is obtained, with lag times of 22 ± 1 h, 25 ± 2 h and 27 ± 1 h, respectively. Our data indicate that 20 and 30% of cholesterol slightly slows down the kinetics of membrane damage in zwitterionic membrane. In contrast, cholesterol in anionic membrane tends to accelerate the membrane leakage with a lag time of 10 ± 1 h in DOPC/DOPS/Chol and 12 ± 1 h in DOPC/DOPS, respectively.
Figure 4. (A) Membrane permeabilization induced by hIAPP on vesicles of different compositions. (B) Representation of the lag time of fibril formation experiments and membrane permeabilization experiments. “This Figure is reproduced in color in the online version of Molecular Membrane Biology”.
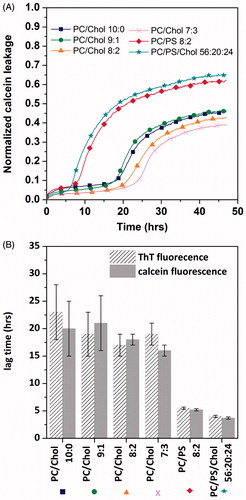
It is interesting to note that the time-scale of dye release shares the same sigmoidal profile as fibril formation, suggesting that the membrane disruption is correlated with fibril formation, as previously postulated for hIAPP in DOPC/DOPS vesicles at pH 7.4 and pH 5.5 (Engel et al., Citation2008). Indeed, the comparison of the kinetic profiles of membrane leakage with the kinetics of fibril formation shows that both processes are characterized by a lag phase and a sigmoidal transition ( and ). Importantly, the lag times for both processes overlap, as indicated by the similar values for lag phase (tlagphase) () and for the midpoints (t0.5) of the sigmoidal transitions (data not shown), suggesting a direct correlation between the kinetics of membrane leakage and of fibril formation in the presence of cholesterol.
The affinity of hIAPP for membranes containing cholesterol is significantly smaller than that for membranes containing anionic lipids
We have shown above that 10 and 20% levels of cholesterol slightly slow down the kinetics of oligomer and fibril formation while anionic lipids accelerate this kinetics. A possible hypothesis is that this variation is caused by differences in membrane insertion and therefore in membrane binding affinity. To test if hIAPP may directly interact with cholesterol-containing membranes, we evaluated the membrane binding affinity by recording 1H NMR experiments. Indeed, we carried out the titration of hIAPP peptide with vesicles containing or not cholesterol. When lipids are added to a solution of hIAPP, the peptide signals gradually decrease owing to the peptide association with membranes (). It is then possible to determine the affinity constant of hIAPP to vesicles (Kd) from signals intensity observed at different peptide:lipid ratios, using the binding equation described in the methods section (see Equation (4)). and shows the titration curves and the affinity constant obtained for different vesicles compositions. These results indicate that the presence of cholesterol slightly affects the affinity of hIAPP for DOPC vesicles: Kd is 1.4 ± 0.3 mM for DOPC vesicles, and increases to 3.5 ± 1.3, 3.7 ± 1.0 and 2.9 ± 0.4 mM for DOPC/Chol 9:1, DOPC/Chol 8:2 and DOPC/Chol 7:3 vesicles, respectively. In contrast, the affinity of hIAPP for vesicles containing anionic lipids is significantly higher suggesting a tighter binding. Indeed, Kd values obtained in the presence of DOPC/DOPS 8:2 and DOPC/DOPS/Chol 56:20:24 are 200 ± 70 µM and 170 ± 75 µM, respectively. These findings corroborate our previous results which indicate a stronger interaction of hIAPP with anionic lipids than zwitterionic lipids. The NMR titration experiments show that cholesterol decreases the binding affinity of monomeric hIAPP for the membranes; they are in agreement with the previous CD and fluorescence observations suggesting that cholesterol slows down the process of fibril formation.
Figure 5. (A) 1H NMR spectra of hIAPP in the absence and in the presence of different concentrations of DOPC vesicles. (B) Experimental NMR data and their corresponding fit for different vesicles compositions. (C) Dissociation constant Kd of hIAPP for different vesicles compositions. “This Figure is reproduced in color in the online version of Molecular Membrane Biology”.
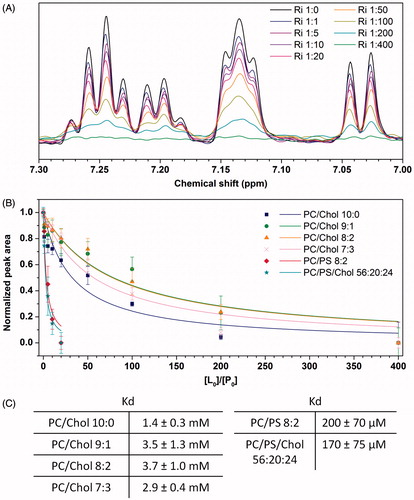
Discussion and conclusion
Cholesterol is an integral component of all animal cell membranes and is essential for cellular functions where it contributes to the structure and dynamics of the membrane and is involved in the regulation of many integral proteins. However, cholesterol is not distributed uniformly within cell membranes and the surface of the cell may show variations in the cholesterol content depending on the physiopathological context. The aim of this research was to thoroughly investigate the effect of 0, 10, 20 and 30% of cholesterol on the aggregational and conformational behaviours of hIAPP as well as its interactions with membranes.
Our results suggest that cholesterol modulates to some extent both aggregational and conformational properties of hIAPP as well as hIAPP-membrane interactions but does not have an effect on the morphology of final fibrils, as it was shown for Aβ, the peptide involved in Alzheimer’s disease (Lau et al., Citation2007). Indeed, TEM images show fibrils with the typical morphology for all investigated vesicles compositions. The CD and 1H NMR data suggest that the presence of 30% of cholesterol induces faster conformational change and monomer depletion, while in the presence of lower amounts of cholesterol, the hIAPP-conformational transition and monomer depletion take longer. These seemingly contradictory results could be ascribed to changes in lipid distribution or segregation. Indeed, we hypothesize that cholesterol at low concentrations (10–20%) may be uniformly distributed within the lipid bilayer, limiting the peptide insertion. However, cholesterol at a concentration of 30% may form clusters in the membrane and induce cholesterol-free regions where hIAPP may insert. In support of this hypothesis, previous monolayer studies showed for Aβ, that the membrane insertion ability was critically controlled by the ratio of cholesterol to phospholipids (Ji et al., Citation2002). Their results indicated that there was a better insertion when the content of cholesterol rises above 30% mol. It seems therefore reasonable to suggest that at low levels (10–20%), cholesterol is able to intercalate between phospholipids in lipid bilayers, thus minimizing the free volume and reducing membrane insertion of hIAPP peptide while at a concentration of 30%, the cholesterol tends to form clusters leading to cholesterol-poor sites in the phospholipid membrane enabling hIAPP to insert.
To understand the molecular basis of the effect of cholesterol or negatively charged lipids on hIAPP, we attempted to quantify the binding affinity of hIAPP initially prepared in the monomeric state for vesicles containing cholesterol or anionic lipids or both. Isothermal titration calorimetry is the most convenient method to determine binding constants; however the rapid aggregation of hIAPP impedes the accurate determination of thermodynamic parameters and the interpretation of the results. Indeed, aggregation of the peptide will cause not only an enthalpy change associated with hIAPP self-association but also the enthalpy changes depend on the different types of oligomeric species present. To overcome this problem, we decided to determine the binding constant by solution 1H NMR since this method is fast (a 1D 1H NMR spectrum can be recorded in a few minutes in the µM to mM range) and enables direct detection of free peptide. Thus, the binding constant determination using liquid state NMR should be rather accurate, provided that the Kd is in the mM range. These NMR experiments indicate that the membrane composition modulates the relative affinity of hIAPP for the membrane, which may affect both membrane permeabilization and fibril formation processes. Indeed, the NMR data reveal that hIAPP has a low affinity for zwitterionic membranes in the absence and in the presence of cholesterol (Kd between 1.4 and 3.7 mM). However, hIAPP seems to have a slightly higher affinity without cholesterol and in the presence of 30% of cholesterol compared to 10 and 20% of cholesterol. We may suggest as aforementioned that in the presence of 10 or 20% of cholesterol, the cholesterol is evenly distributed in the membrane and fills the interstitial space in the lipid acyl chains reducing the ability of the peptide to insert. A higher percentage of cholesterol (30%) likely leads to the coexistence of cholesterol-enriched domain and cholesterol-free domain in which the peptide could better insert and bind with a higher affinity. In the presence of negatively charged lipids, the affinity of hIAPP for membranes is increased (Kd around 170 and 200 µM) both in the absence and in the presence of cholesterol. This suggests that hIAPP-membrane interactions are driven at least in part by electrostatic forces. Similar Kd values in PC/PS vesicles have been obtained by another research group, who determined the binding constant by CD and found a value of 190 ± 85 µM (Sciacca et al., Citation2012). In addition to an enhancement in binding affinities, the presence of negatively charges also induces a stabilisation of helical conformations which may be related to a membrane insertion of hIAPP. Indeed, we may hypothesize that in the presence of anionic lipids, hIAPP is located within the lipid bilayer, while in the absence of anionic lipids hIAPP is mainly positioned at the interface of the lipid bilayer as described for hIAPP1–19 (Xu et al., Citation2011).
Engel et al., (Citation2008) demonstrated that hIAPP membrane damage was linked to hIAPP fibril formation at pH 7.4 in the presence of DOPC/DOPS vesicles. They postulated that, at least under their conditions, it is the growth of hIAPP fibrils at the membrane surface rather than the formation of oligomeric species that causes hIAPP-induced membrane damage. Thus, as soon as the fibril develops on the membrane surface, the structural integrity of the membrane is simultaneously compromised. Later, a similar study was performed at pH 5.5 (the pH of the β-cells granules of the pancreas where mature hIAPP is stored) and confirmed the synchronization of the kinetics profiles for hIAPP fibril growth and the induction of dye leakage (Khemtémourian et al., Citation2011). In the present study, our results show that in the presence of 0, 10, 20 and 30% of cholesterol the kinetics of fibril formation and the kinetics of membrane damage are still synchronized, indicating that the process of fibril formation on membrane surfaces is responsible for abolishing the membrane barrier function, in agreement with previous results (Engel et al., Citation2008; Khemtémourian et al., Citation2009, Citation2011). In addition, in we clearly see that the fibrils seem to propagate along the vesicles surface suggesting a strong interaction and eventually membrane damage, as previously postulated (Caillon et al., Citation2013; Engel et al., Citation2008). The present findings seem to be consistent with observations made by other research groups not only for hIAPP (Friedman et al., 2009) but also for Aβ peptide involved in Alzheimer’s disease (Jan et al., Citation2011). Indeed, the latter study provides evidence that it is not a specific oligomeric state of Aβ42 that induces cell death and toxicity but rather the dynamic process of fibril formation. Therefore, the increasing number of experimental data in terms of diversity of membrane compositions and peptides investigated provides compelling evidence for the generality of the mechanism of amyloid membrane damage in relation to fibril growth at the membrane.
Acknowledgements
Géraldine Toutirais (IBPS FRE 3631 – Institut de Biologie Paris-Seine, service de microscopie électronique – Université Pierre et Marie Curie, France) is acknowledged for the electron microscopy.
Declaration of interest
The authors report no conflict of interest. The authors alone are responsible for the content and writing of the paper.
References
- Akesson B, Panagiotidis G, Westermark P, Lundquist I. 2003. Islet amyloid polypeptide inhibits glucagon release and exerts a dual action on insulin release from isolated islets. Regul Pept 111:55–60
- Anguiano M, Nowak RJ, Lansbury PT Jr. 2002. Protofibrillar islet amyloid polypeptide permeabilizes synthetic vesicles by a pore-like mechanism that may be relevant to type II diabetes. Biochemistry 41:11338–11343
- Bartolini M, Bertucci C, Bolognesi ML, Cavalli A, Melchiorre C, Andrisano V. 2007. Insight into the kinetic of amyloid beta (1–42) peptide self-aggregation: Elucidation of inhibitors’ mechanism of action. ChemBioChem 8:2152–2161
- Brender JR, Salamekh S, Ramamoorthy A. 2012. Membrane disruption and early events in the aggregation of the diabetes related peptide IAPP from a molecular perspective. Acc Chem Res 45:454–462
- Brunham LR, Kruit JK, Pape TD, Timmins JM, Reuwer AQ, Vasanji Z, et al. 2007. beta-cell ABCA1 influences insulin secretion, glucose homeostasis and response to thiazolidinedione treatment. Nature Med 13:340–347
- Caillon L, Lequin O, Khemtémourian L. 2013. Evaluation of membrane models and their composition for islet amyloid polypeptide-membrane aggregation. Biochim Biophys Acta 1828:2091–2098
- Cao P, Marek P, Noor H, Patsalo V, Tu LH, Wang H, et al. 2013a. Islet amyloid: From fundamental biophysics to mechanisms of cytoxicity. FEBS Lett 587:1106–1118
- Cao P, Abedini A, Raleigh DP. 2013b. Aggregation of islet amyloid polypeptide: From physical chemistry to cell biology. Curr Opin Struct Biol 23:82–89
- Cao P, Abedini A, Wang H, Tu LH, Zhang X, Schmidt AM, Raleigh DP. 2013c. Islet amyloid polypeptide toxicity and membrane interactions. Proc Nat Acad Sci USA 110:19279–19284
- Chiti F, Dobson CM. 2006. Protein misfolding, functional amyloid, and human disease. Annu Rev Biochem 75:333–366
- Cho WJ, Jena BP, Jeremic AM. 2008. Nano-scale imaging and dynamics of amylin-membrane interactions and its implication in type II diabetes mellitus. Methods Cell Biol 90:267–286
- Cho WJ, Trikha S, Jeremic AM. 2009. Cholesterol regulates assembly of human islet amyloid polypeptide on model membranes. J Mol Biol 393:765–775
- Clark A, Wells CA, Buley ID, Cruickshank JK, Vanhegan RI, Matthews DR, et al. 1988. Islet amyloid, increased A-cells, reduced B-cells and exocrine fibrosis: Quantitative changes in the pancreas in type 2 diabetes. Diabetes Res 9:151–159
- Cooper GJ, Willis AC, Clark A, Turner RC, Sim RB, Reid KB. 1987. Purification and characterization of a peptide from amyloid-rich pancreases of type 2 diabetic patients. Proc Natl Acad Sci USA 84:8628–8632
- Dante S, Hauss T, Dencher NA. 2006. Cholesterol inhibits the insertion of the Alzheimer’s peptide Aβ(25-35) in lipid bilayer. Eur Biophys J 35:523–531
- Demuro A, Mina E, Kayed R, Milton SC, Parker I, Glabe CG. 2005. Calcium dysregulation and membrane disruption as a ubiquitous neurotoxic mechanism of soluble amyloid oligomers. J Biol Chem 280:17294–17300
- Engel MFM, Khemtémourian L, Kleijer CC, Meeldijk HJ, Jacobs J, Verkleij AJ, et al. 2008. Membrane damage by human islet amyloid polypeptide through fibril growth at the membrane. Proc Natl Acad Sci USA 105:6033–6038
- Friedman R, Pellarin R, Caflisch A. 2009. Amyloid aggregation on lipid bilayers and its impact on membrane permeability. J Mol Biol 387:407–415
- Hao M, Bogan JS. 2009. Cholesterol regulates glucose-stimulated insulin secretion through phosphatidylinositol 4,5-bisphosphate. J Biol Chem 284:29489–29498
- Hasper HE, Kramer NE, Smith JL, Hillman JD, Zachariah C, Kuipers OP, et al. 2006. An alternative bactericidal mechanism of action for lantibiotic peptides that target lipid II. Science 313:1636–1637
- Jan A, Adolfsson O, Allaman I, Buccarello AL, Magistretti PJ, Pfeifer A, et al. 2011. Abeta42 neurotoxicity is mediated by ongoing nucleated polymerization process rather than by discrete Abeta42 species. J Biol Chem 286:8585–8596
- Jayasinghe SA, Langen R. 2005. Lipid membranes modulate the structure of islet amyloid polypeptide. Biochemistry 44:12113–12119
- Ji SR, Wu JY, Sui SF. 2002. Cholesterol is an important factor affecting the memrbane insertion of β-amyloid peptide (Aβ1-40), which may potentially inhibit the fibril formation. J Biol Chem 277:6273–6279
- Kahn SE, D’Alessio DA, Schwartz MW, Fujimoto WY, Ensinck JW, Taborsky GJ Jr, Porte D Jr. 1990. Evidence of cosecretion of islet amyloid polypeptide and insulin by beta-cells. Diabetes 39:634–638
- Karlsson E. 1999. IAPP as a regulator of glucose homeostasis and pancreatic hormone secretion (review). Int J Mol Med 3:577–584
- Khemtémourian L, Lahoz Casarramona G, Syulen DPL, Hackeng TM, Meeldijk JD, De Kruijff B, et al. 2009. Impaired processing of human pro-islet amyloid polypeptide is not a causative factor for fibril formation or membrane in vitro. Biochemistry 48:10918–10925
- Khemtémourian L, Domenech E, Doux JPF, Koorengevel MC, Killian JA. 2011. Low pH acts as inhibitor of membrane damage induced by human islet amyloid polypeptide. J Am Chem Soc 133:15598–15604
- Khemtémourian L, Killian JA, Höppener JWM, Engel MFM. 2008. Recent insights in islet amyloid polypeptide-induced membrane disruption and its role in beta-cell death in type 2 diabetes mellitus. Exp Diabetes Res 2008:421287
- Knight JD, Miranker AD. 2004. Phospholipid catalysis of diabetic amyloid assembly. J Mol Biol 341:1175–1187
- Knight JD, Hebda JA, Miranker AD. 2006. Conserved and cooperative assembly of membrane-bound α-helical states of islet amyloid polypeptide. Biochemistry 45:9496–9508
- Konarkowska B, Aitken JF, Kistler J, Zhang S, Cooper GJ. 2006. The aggregation potential of human amylin determines its cytotoxicity towards islet beta-cells. FEBS J 273:3614–3624
- Lau TL, Gehman JD, Wade JD, Masters CL, Barnham KJ, Separovic F. 2007. Cholesterol and Clioquinol modulation of Aβ(1-42) interaction with phospholipid bilayers and metals. Biochim Biophys Acta 1768:3135–3144
- Liu M, Mao X, Ye C, Huang H, Nicholson JK, Lindon JC. 1998. Improved WATERGATE pulse sequences for solvent suppression in NMR spectroscopy. J Magn Reson 132:125–129
- Lorenzo A, Razzaboni B, Weir GC, Yankner BA. 1994. Pancreatic islet cell toxicity of amylin associated with type-2 diabetes mellitus. Nature 368:756–760
- Lukinius A, Wilander E, Westermark GT, Engstrom U, Westermark P. 1989. Co-localization of islet amyloid polypeptide and insulin in the B cell secretory granules of the human pancreatic islets. Diabetologia 32:240–244
- Mirzabekov TA, Lin MC, Kagan BL. 1996. Pore formation by the cytotoxic islet amyloid peptide amylin. J Biol Chem 271:1988–1992
- Potter KJ, Abedini A, Marek P, Klimek AM, Butterworth S, Driscoll M, et al. 2010. Islet amyloid deposition limits the viability of human islet grafts but not porcine islet grafts. Proc Natl Acad Sci USA 107:4305–4310
- Reda TK, Geliebter A, Pi-Sunyer FX. 2002. Amylin, food intake, and obesity. Obes Res 10:1087–1091
- Rouser G, Fkeischer S, Yamamoto A. 1970. Two dimensional then layer chromatographic separation of polar lipids and determination of phospholipids by phosphorus analysis of spots. Lipids 5:494–496
- Rushing PA, Hagan MM, Seeley RJ, Lutz TA, D’Alessio DA, Air EL, Woods SC. 2001. Inhibition of central amylin signaling increases food intake and body adiposity in rats. Endocrinology 142:5035
- Sani MA, Gehman JD, Separovic F. 2011. Lipid matrix plays a role in Abeta fibril kinetics and morphology. FEBS Lett 585:749–754
- Sciacca MFM, Brender JR, Lee DK, Ramamoorthy A. 2012. Phosphatidylethanolamine enhaces amyloid fiber-dependent membrane fragmentation. Biochemistry 51:7676–7684
- Seeliger J, Weise K, Opitz N, Winter R. 2012. The effect of abeta on IAPP aggregation in the presence of an isolated beta-cell membrane. J Mol Biol 421:348–363
- Selkoe DJ. 2003. Folding proteins in fatal ways. Nature 426:900–904
- Sipe JD. 1994. Amyloidosis. Crit Rev Clin Lab Sci 31:325–354
- Sparr E, Engel MFM, Sakharov DV, Sprong M, Jacobs J, de Kruijff B, et al. 2004. Islet amyloid polypeptide-induced membrane leakage involves uptake of lipids by forming amyloid fibers. FEBS Lett 577:117–120
- Stridsberg M, Sandler S, Wilander E. 1993. Cosecretion of islet amyloid polypeptide (IAPP) and insulin from isolated rat pancreatic islets following stimulation or inhibition of beta-cell function. Regul Pept 45:363–370
- Trikha S, Jeremic AM. 2011. Clustering and internalization of toxic amylin oligomers in pancreatic cells require plasma membrane cholesterol. J Biol Chem 286:36086–36097
- Wakabayashi M, Matsuzaki K. 2009. Ganglioside-induced amyloid formation by human islet amyloid polypeptide in lipid rafts. FEBS Lett 583:2854–2858
- Westermark GT, Westermark P, Berne C, Korsgren O. 2008. Widespread amyloid deposition in transplanted human pancreatic islets. N Engl J Med 359:977–979
- Westermark P, Andersson A, Westermark GT. 2011. Islet amyloid polypeptide, islet amyloid, and diabetes mellitus. Physiol Rev 91:795–826
- Westermark P, Wernstedt C, Wilander E, Hayden DW, O’Brien TD, Johnson KH. 1987. Amyloid fibrils in human insulinoma and islets of Langerhans of the diabetic cat are derived from a neuropeptide-like protein also present in normal islet cells. Proc Natl Acad Sci USA 84:3881–3885
- Xu W, Wei G, Su H, Nordenskiöld L, Mu Y. 2011. Effect of cholesterol on pore formation in lipid bilayers induced by human islet amyloid polypeptide fragments: A coarse-grained molecular dynamics study. Phys Rev 84:051922