Abstract
4-Hydroxy-2-nonenal (4-HNE) is a reactive aldehyde and a lipid peroxidation product formed in biological tissues under physiological and pathological conditions. Its concentration increases with oxidative stress and induces deleterious modifications of proteins and membranes. Mitochondrial and cytosolic isoforms of creatine kinase were previously shown to be affected by 4-HNE. In the present study, we analyzed the effect of 4-HNE on mitochondrial creatine kinase, an abundant protein from the mitochondrial intermembrane space with a key role in mitochondrial physiology. We show that this effect is double: 4-HNE induces a step-wise loss of creatine kinase activity together with a fast protein aggregation. Protein-membrane interaction is affected and amyloid-like networks formed on the biomimetic membrane. These fibrils may disturb mitochondrial organisation both at the membrane and in the inter membrane space.
Introduction
4-Hydroxy-2-nonenal (4-HNE) is a major peroxidation product of ω-6 polyunsaturated fatty acids (e.g. linoleic acid, and arachidonic acid) constituting membrane phospholipids (Esterbauer et al., Citation1991; van Kuijk et al., Citation1990). This short-chain aldehyde which is formed and metabolized in biological tissues readily reacts with several class of cellular components. It was notably shown to alter protein function by reacting with cysteine, histidine or lysine residues (Esterbauer et al., Citation1991; Nadkarni & Sayre, Citation1995), to react with the amine group of phosphatidylethanolamines (Bacot et al., Citation2003; Guichardant et al., Citation1998) and to modify membrane properties (Lecompte et al., Citation2005). Its concentration drastically increases during oxidative stress and under pathological conditions such as neurodegenerative diseases, including Parkinson’s disease, amyotrophic lateral sclerosis, and Alzheimer’s disease (Zarkovic, Citation2003). 4-HNE attains high levels (∼20 μM) in brain (Markesbery & Lovell, Citation1998; Sayre et al., Citation1997) and plasma (McGrath et al., Citation2001) of Alzheimer patients compared to aged matched controls, pointing out its involvement in this disease (Poli & Schaur, Citation2000). Even much higher local concentrations of 4-HNE (50 μM to 5 mM) accumulate in the vicinity of the membrane and membrane bound proteins (Esterbauer et al., Citation1991; Reed et al., Citation2008). Moreover, proteomic studies have shown that a large number of proteins in Alzheimer patient brain – and notably also in an early stage of the disease with mild cognitive impairment – are modified by reaction with 4-HNE (Butterfield et al., Citation2006; Reed et al., Citation2008). 4-HNE is known to alter cellular physiology in other tissues as well. It has been shown that 4-HNE binds to rat mitochondrial membranes, oxidizes phospholipids and protein thiols (Chen et al., Citation1995), and alters membrane fluidity (Chen & Yu, Citation1994). Considerable amounts of HNE-modified proteins have been detected in isolated perfused rat heart after ischemia and reperfusion (Eaton et al., Citation1999; Siems et al., Citation1991) and, in muscle, 4-HNE has been reported to impair insulin signaling and glucose uptake (Pillon et al., Citation2012). More recently, Ramananthan et al. reported that incubation with 1 mM 4-HNE altered the structure of isolated bovine heart mitochondria (Ramanathan et al., Citation2012). In their study aiming at identifying carbonylated proteins inside the diaphragm of septic rats, Barreiro et al. (Citation2005) showed that carbonylation was mediated by lipid peroxidation products in general and by 4-HNE in particular. Among carbonylated proteins they identified mitochondrial creatine kinase (mtCK), a very abundant protein of the mitochondrial intermembrane space (Kupriyanov et al., Citation1981; Kuznetsov & Saks, Citation1986; Quemeneur et al., Citation1990; Vial et al., Citation1986) which plays an essential role both in cell energy homeostasis (Guzun et al., Citation2012; Saks et al., Citation2010) and mitochondrial structure (Lenz et al., Citation2007; Miller et al., Citation1997). These roles are related to the ability of the octameric form of mtCK to bind to the outer face of the inner mitochondrial membrane (Marcillat et al., Citation1987). Membrane binding occurs mainly via electrostatic interactions between cardiolipin (CL) (Schlame & Augustin, Citation1985) and the positively charged residues of the protein C-terminal end (Lys 380, Lys 379 and Lys 369) (Schlattner et al., Citation2004), even if hydrophobic interactions are also involved (Maniti et al., Citation2009b). The composition of CL side chains, with a large majority of linoleyl chains, and its implication in mitochondrial electron chain transport make this phospholipid extremely susceptible to free radical oxidation by reactive oxygen species (Yin & Zhu, Citation2012) leading to the formation of 4-HNE as one of the peroxidation products (Yin & Zhu, Citation2012).
Since mitochondrial creatine kinase (mtCK) is abundant in the intermembrane space (Kupriyanov et al., Citation1981; Kuznetsov & Saks, Citation1986; Quemeneur et al., Citation1990; Vial et al., Citation1986) and is known to be one of the prime targets of 4-HNE (Barreiro et al., Citation2005), we studied the effects of 4-HNE on mtCK structure and membrane binding properties. Moreover, 4-HNE was shown to inhibit creatine kinase activity of the cytosolic brain isoform (CK-BB) in a dose-dependent manner and to oxidatively modify specific aminoacid residues (Eliuk et al., Citation2007). We report here that 4-HNE not only inactivates mtCK, but also induces intensive association of protein molecules both in solution and in a membrane bound form. Networks of mtCK, reminiscent of amyloid fibrils, can be put in relation with pathological conditions in which this protein was shown to form crystalline structures (O’Gorman et al., Citation1997a, Citation1997b; Stadhouders et al., Citation1994).
The impact of this organization on other mitochondrial membrane-linked functions is unknown but one can expect that an array of membrane bound creatine kinase will have an effect on diffusive movements of proteins within the membrane. More specifically, perturbation of cardiolipin distribution within the mitochondrial membrane is likely to affect the function of signaling platforms in which this phospholipid is involved (Schug & Gottlieb, Citation2009).
Materials and methods
4-HNE was chemically synthesized in the Organic Chemistry Laboratory at INSA-Lyon, (Villeurbanne, France), as described elsewhere (Soulere et al., Citation2007) and resuspended in ethanol at 100 mM. 4-HNE was tested by GC-MS () and its purity was estimated to be over 95%. Two isomers syn and anti, resulting from the derivation of aldehyde group to pentafluorobenzyloxime, were present and showed the same fragments with different relative intensities (). Bovine heart cardiolipin (CL), egg yolk phosphatidylcholine (PC), egg yolk phosphatidylethanolamine (PE), as well as Tris and dithiotreitol (DTT), were obtained from Sigma (Saint Quentin Fallavier, France). For differential capacity measurements egg yolk PC and egg yolk PE were obtained from Lipid Products (Nutfield, Redhill, Surrey, UK).
Figure 1. GC-MS analysis of synthesized 4-HNE: (A) NICI total ion current chromatogram of derivatized 4-HNE; (B) NICI mass spectrum of the isomer eluted at 12.16 min; (C) NICI mass spectrum of the isomer eluted at 12.30 min.
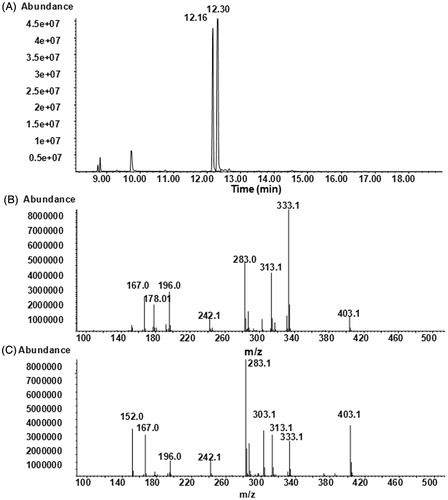
Protein expression and purification
Recombinant rabbit heart mtCK (MW octamer 360,000 Da) was prepared and purified as described elsewhere (Marcillat et al., Citation1999). The purified enzyme was obtained in 20 mM TrisHCl pH 7.4, 0.1 mM EDTA, 0.2 mM DTT, at a protein concentration of about 1 g/l, determined by the Lowry method using bovine serum albumin as standard. The purity of CK was established using SDS-PAGE ().
4-HNE effect on mtCK activity
MtCK (0.5 μM octamer) was incubated with the desired 4-HNE concentration at 21 °C in 20 mM Tris-HCl pH 7.4 buffer containing 0.1 mM EDTA, 0.2 mM DTT. 4-HNE stock solution was prepared in ethanol. Enzyme activity was measured using the pH stat technique (Font et al., Citation1983). No inactivation was observed by incubating the enzyme with the equivalent amount of ethanol.
Effect of 4-HNE on mtCK solution turbidity
Absorbance at 450 nm before and after addition of 4-HNE was measured using a HP 8453 spectrometer. A 0.5 μM mtCK or 0.25 μM mtCK in 20 mM TrisHCl pH 7.4 buffer 0.1 mM EDTA containing 0.2 mM DTT was introduced in a quartz cuvette and 4-HNE was added at the indicated concentrations. The absorbance of the protein solution before 4-HNE addition was taken as base line. Control measurements were performed with equivalent amounts of ethanol. In these conditions, ethanol had no effect on the turbidity of mtCK solution.
FTIR measurements
MtCK was lyophilized and resolubilized in 20 mM Tris-HCl-2H2O (p2H 7.4) buffer at 10 g/l final mtCK concentration. The p2H was measured with a glass electrode and was corrected by a value of 0.4 according to Glasoe & Long (Citation1960). Samples were loaded between two CaF2 circular cells, with a 50 μm Teflon spacer. FTIR spectra were recorded with a Nicolet 510 M FTIR spectrometer which was continuously purged with dry air. The infrared cell was thermostated with a water circulation bath at 21 °C. The nominal spectral resolution was 4 cm−1; 256 scans were collected and co-added per sample spectrum, and Fourier-transformed for each sample. The infrared spectra of the buffer and of residual water vapour were subtracted from the infrared spectrum of the sample. Each spectrum is the average of three independent measurements.
Film formation and surface pressure measurements for Brewster angle microscopy (BAM)
A circular Teflon trough (Riegler & Kirstein, Potsdam, Germany) with a volume of 30 ml and a surface of 27 cm2 was used. The trough was filled with 20 mM Tris-HCl buffer, pH 7.4. Phospholipid monolayers were formed on a clean air-buffer interface by spreading the phospholipids dissolved in chloroform to attain a lateral surface pressure between 30 and 35 mN/m. After pressure stabilization, a final concentration of 4 nM mtCK, modified or not by 4-HNE, was injected in the subphase using a Hamilton syringe. In these conditions, mtCK has previously been shown to be in its octameric form at the air-water interface (Vernoux et al., Citation2006). The surface pressure was monitored for the duration of the experiment at 21 °C.
Visualisation of protein or lipid films by BAM
A commercial Brewster angle microscope manufactured by NFT (Göttingen, Germany) mounted on a 30 ml trough was used to determine the morphology of the phospholipid film (Henon & Meunier, Citation1991; Vollhardt, Citation1996) in the presence or absence of protein. BAM image size was 430 × 540 μm with 2 μm spatial resolution. Measurements were performed at different shutter speeds (integration time) ranging from 1:250–1:2000 s to adapt to different illumination levels. Average and local thickness of the interfacial film were determined from the measured intensity parameter for different shutter speeds, the grey level (GL), according to the procedure previously described (Maniti et al., Citation2009a, Vernoux et al., Citation2009). For ultra thin films, the reflectance depends on both the thickness and refractive index of the monolayer:
(1)
where λ is the laser wavelength, d is the film thickness and n is the refractive index of the interfacial film. For a measured reflectance and at a given refractive index, the BAM software allowed us to determine the average or local thickness of the film, as previously described. According to ellipsometric studies of phosphatidylcholines at the air-water interface (Ducharme et al., Citation1990), the refractive index of a monolayer constituted of lipids in a fluid state used in this study was 1.46 for all lipid monolayers. As an approximation, the same refractive index was used for monolayers in the presence of protein, as the density of lipids at the interface was relatively high, and the protein layer beneath hydrated.
Alternative current polarographic measurements
The measurement of the differential capacity (C) of a hanging mercury drop electrode in respect to the potential (C vs. E) was carried out as described elsewhere (Lecompte et al., Citation1994; Maniti et al., Citation2010) in a Metrohm polarographic cell. Phospholipids were added until a stable and constant value of C was reached over a wide potential range between −0.2 and −0.8 V. In our experiments, 0.4 μg/cm2 of PC:PE:CL (2:1:1) mixture were required to ensure a condensed lipid monolayer at the surface of the electrolyte, i.e. Tris-HCl pH 7.4 buffer containing 50 mM NaCl, at 21 °C. Oxygen was displaced from the solution by argon and equilibrium between the bulk and the surface layer was facilitated by gently stirring the subphase before electrochemical measurements. The starting potential was chosen in the stable region of the monolayer, namely at −0.2 V. The protein, modified or not by 4-HNE, was introduced underneath the monolayer at a final concentration of 4 nM.
Results
4-HNE action on mtCK enzymatic activity
The effect of the oxidative agent 4-HNE on mtCK enzymatic activity was first tested. The enzyme (0.5 μM) was incubated with increasing concentrations of 4-HNE, ranging from 62.5 μM to 1 mM. As shown in , top panels, a dose-dependent decrease in mtCK enzymatic activity was observed. The measured residual activity varied with the 4-HNE concentration used: 60% for 62.5 μM (, diamonds), 30% for 250 μM (, squares) and 10% for the highest concentration used, 1 mM (, triangles). Several steps can been seen in the inactivation time curse. The first one is a 40% drop in enzymatic activity which is observed at all 4-HNE concentrations used. With 250 μM 4-HNE, the initial mtCK activity decrease is followed – after 40 min of action – by a second step leading to a state with about 30% residual activity. With 1 mM 4-HNE, a third step can be seen (10% residual activity) after 50 min.
Figure 3. 4-HNE effect on mtCK enzymatic activity and oligomerization: Top: Normalized enzymatic activity of mtCK (0.5 μM) in the absence (A) or in presence of 62.5 μM (B), 250 μM (C) or 1 mM (D) 4-HNE. Representative curves of at least three independent measurements. Bottom: Turbidity of mtCK solution after addition of 62.5 μM (E), 250 μM (F) or 1 mM 4-HNE (G) to 0.25 μM mtCK (E) or 0.5 μM mtCK (F, G).
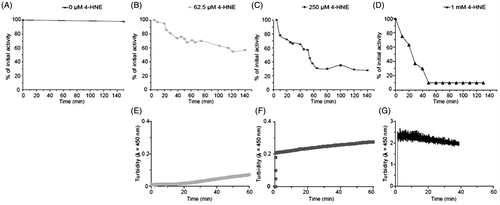
4-HNE-induced oligomerization of mtCK
The turbidity (absorbance at 450 nm) of mtCK solution was measured before and after addition of 4-HNE (, bottom panels). An increase in turbidity was recorded at all 4-HNE concentrations used. With 62.5 μM 4-HNE, a lag phase was observed, and the increase in absorbance became measurable 20 min after 4-HNE addition (). At this incubation time less than 5% of activity was lost, the increase in turbidity coincides with the onset of the main inactivation step. The addition of 250 μM 4-HNE induced an instant increase in turbidity (). In this last condition, mtCK activity was decreased over a much longer time scale. The two phases seen on this inactivation time course () correspond to a monotonous slow and limited increase in turbidity (). After addition of 1 mM 4-HNE, absorbance immediately and drastically increased from 0 up to 2.4 (). Thus, the increase in the turbidity of mtCK solution, related to formation of mtCK oligomers, can be faster than the loss of enzymatic activity. MtCK molecules may associate to form oligomers andthis phenomenon is not strictly linked to the loss of enzymatic activity. The subsequent question was to which extent 4-HNE altered protein structure and membrane interaction.
Structural modifications induced by 4-HNE analyzed by infrared measurements
Infrared spectra of mtCK incubated with or without 4-HNE () showed some remarkable differences in the amide I band region (1700–1600 cm−1). Local minima on the second derivative (dotted lines) were used to identify contributions on mtCK spectrum. Several minima were present, with two major contributions at 1651 cm−1 and 1631 cm−1 ( full line). These two vibrational bands are attributed to α-helices and antiparallel β-sheet contributions (Barth, Citation2007) and are in line with those previously reported for mtCK (Granjon et al., Citation2001) and correspond to the crystalline structure of mtCK (Fritz-Wolf et al., Citation1996). After incubation with 4-HNE, two additional shoulders appeared on the protein spectrum at 1616 and 1684 cm−1 ( full line), with corresponding minima on second derivative spectrum ( dotted line). The relative contributions of each type of secondary structure were determined after deconvolution () of protein FTIR spectra using the residual method (Peak Fit™). The percentage of each type of structure from the total peak area deduced from this deconvolution was plotted as a pie chart in . Spectra of mtCK before and after 4-HNE treatment showed a major α-helical contribution at 1651 cm−1 (62% and 50%, respectively) with additional bands corresponding to random structures around 1648 cm−1 (10%) and β turns around 1660 cm−1 (6 and 9%, respectively). Several antiparallel β-sheet contributions were present, with major low wavenumber bands at 1626 and 1634 cm−1 and corresponding high wavenumber contributions at 1675–1680 cm−1 (Barth, Citation2007; Jackson & Mantsch, Citation1995). Overall, these bands accounted for ∼20% from the mtCK amide I band, before and after treatment with 4-HNE. The secondary structure of the protein was thus not dramatically modified, however one can note a decrease in the 1651 cm−1 band from 62–50%, associated with an increase in 1616 cm−1 band from 1–7% and 1684 cm−1 band from 1–4%. These 1616/1684 cm−1 vibrational bands are usually associated with formation of intermolecular β-sheet structures (Goormaghtigh et al., Citation1994; Jackson & Mantsch, Citation1995). Such intermolecular β-sheet structures are the hallmark for formation of self-assembled amyloid fibers in proteins (Ridgley et al., Citation2013). Thus, the structural modifications of mtCK upon 4-HNE action observed on IR spectra are in favour of intermolecular interactions and formation of protein “amyloid-like” oligomers.
Figure 4. Structural modifications of mtCK after incubation with 4-HNE. Infrared spectra, in the region of amide I band (full lines) and second derivative (dotted lines) of mtCK (A) and of 4-HNE-modified mtCK (B). (C) and (D) Deconvolution of spectra from panel A and B, respectively. (E) and (F) Relative percentage of secondary structure contributions from the total peak area before (E) and after 4-HNE treatment (F).
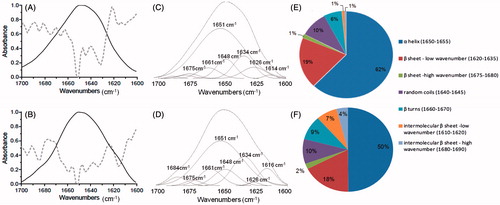
MtCK-membrane interaction after 4-HNE treatment
The effect of 4-HNE on the ability of mtCK to bind to membranes was analyzed using Langmuir monolayers at the air-water interface as membrane model and analyzed by BAM. A lipid monolayer was formed at the air buffer interface by spreading a lipid solution. The organization of the lipid monolayer can be easily controlled by tuning the lateral surface pressure of the monolayer. We have chosen to perform our experiments at a surface pressure around 35 mN/m, as this value is considered to render an accurate image of the phospholipid organization in a bilayer (Marsh, Citation1996). The measurements are done at the Brewster angle, at which no reflection occurs at the air-water interface in the absence of a lipid monolayer. When a PC:PE:CL (2:1:1) monolayer was present at the interface, reflection occurred and the intensity of the reflected light increased. As indicated in the Material and methods section the intensity of the reflected light depends on the thickness of the interfacial layer, which was estimated to be 1.9 nm. The observed monolayer was homogeneous (). As previously shown using Langmuir monolayers and BAM, mtCK readily interacted with the PC:PE:CL (2:1:1) monolayer (Maniti et al., Citation2009b; Vernoux et al., Citation2007). Bright spots, coexisting with large fractal-shaped clusters of high reflectivity ( and ) were observed by BAM. These effects were only observed when CL was present in the monolayer composition, but not when the monolayer was composed of pure PC or PC:PE (not shown), attesting that CL is a partner in this interaction between mtCK and the CL-containing membranes. After 4-HNE treatment (10% residual activity), mtCK adducts also induced formation of bright networks () beneath the PC:PE:CL monolayer, which grew to form continuous networks after longer incubation ( and ). No effect on the monolayer was observed when the monolayer was constituted of pure PC or PC:PE (not shown). Of note, an equivalent final 4-HNE concentration (8 μM) injected beneath a PC:PE:CL (2:1:1) monolayer induced very little changes in the aspect of the monolayer ().
Figure 5. MtCK interaction with PC:PE:CL (2:1:1) monolayer: BAM images of a PC:PE:CL (2:1:1) monolayer before (A) and 2 h after injection of 4 μM 4-HNE (B) or of 4 nM mtCK, unmodified (C, D) or 4-HNE-modified mtCK (E, F and G, 1 h, 1 h 30 and 2 h 30, respectively). Left: detail of images D and G and 3D view (ImageJ 3D surface plot) of these regions. Grey level (GL), shutter speed (S) and average thickness in nm are indicated below the images.
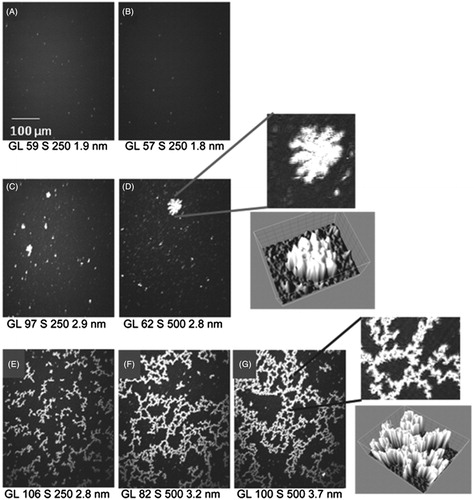
The capacity of protein to insert into the monolayer was then analyzed after 4-HNE treatment using differential capacity (C) measurements, performed with a PC:PE:CL (2:1:1) monolayer in contact with a hanging mercury drop electrode. Variations in C around the potential of zero charge (pzc) of the electrode (−0.45 V Ag/AgCl sat. KCl), where no or negligible electric field is superimposed, permit to assess whether a molecule, or parts of it, penetrate into the monolayer or adsorb at the polar headgroup (Lecompte et al., Citation1994, Citation1998).
The highest value of C corresponds to the pure electrolyte (Lecompte et al., Citation1994). When a compact lipid monolayer is formed at the air-water interface C attains its minimum and remains constant over a large range of potential around pzc (). For the PC:PE:CL (2:1:1) monolayer, in the absence of protein, C was stable and constant between −0.2 V and −0.8 V (, full line). This indicated that the monolayer reached a condensed state, where the lipid molecules formed a compact layer and no electrolyte molecules came in contact with the electrode (Lecompte et al., Citation1994, Citation1998; Lecompte, Citation2005).
Figure 6. Differential capacity vs. potential curves of a hanging mercury drop electrode in contact with a condensed PC:PE:CL (2:1:1) monolayer before (full lines) and after addition of mtCK (dotted lines) or 4-HNE modified mtCK (small dots) at 4 nM final concentration in the supporting electrolyte.
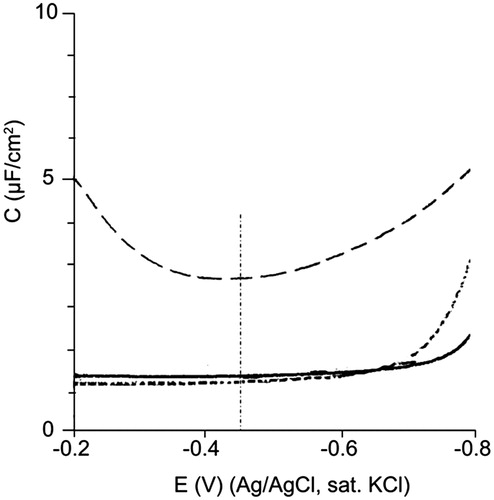
A protein that perturbs the arrangement of phospholipids at the interface also modifies the differential capacity of the system. For a protein that penetrates into the monolayer, i.e. crosses the polar head-hydrocarbon chain interface, the circuit will exhibit properties equivalent to that of two electrical condensers arrayed in parallel, with contributions from both the protein and the lipid monolayer. The value of C will depend on the amount of protein penetrating the membrane, following the equation:
(2)
where CL, and Cp are the differential capacities of the lipid and protein monolayers, respectively, and h the fraction of lipid monolayer that has been penetrated by the protein, i.e. the fraction of phospholipids replaced by protein at the electrode. According to this and because CP is much higher than CL, the expected effect of protein insertion among lipids is an increase in C. In the present conditions, mtCK binding to the lipid monolayer induces an increase of 2.5 μF/cm2 in C (, dotted line). This increase is in the range of those observed with other proteins such as Coagulation Factor Xa (Lecompte et al., Citation2005) and Va (Lecompte et al., Citation1994) in interaction with PS-containing monolayers (2–4 μF/cm2, depending on protein concentration or PS percentage in the monolayer) or apolipoprotein AI (Lecompte et al., Citation2005) in interaction with PC:PE monolayers in various molar ratios (0.5–3.5 μF/cm2). This indicated that parts of protein came into contact with the electrode, thus penetrated into the monolayer, as previously described (Maniti et al., Citation2010). The U-shape of the curve in the presence of protein penetrating between lipids is typical and may reflect re-organization of the mixed monolayer when the potential varies.
For a protein that is adsorbed by the condensed lipid monolayer the capacity of the system (C) can be described by two condensers, the lipid membrane and the protein layer, which are arrayed in series. The capacity of the system can thus be expressed as:
(3)
In this case, the differential capacity is expected to decrease with the amount of protein adsorbed by the lipids. The extent of the decrease will depend on the proportion of phospholipid monolayer area covered by the protein, and on the values of CL and CP.
Injection of the 4-HNE-pretreated mtCK induced a 20% decrease in the value of C (, small dots). For several other proteins previously studied (Lecompte et al., Citation1994, Citation1998), the decrease in C induced by protein adsorption is masked by the greater increase in C due to protein insertion. The decrease in C recorded in the case of the 4-HNE-modified mtCK may illustrate massive protein adsorption at the monolayer, without insertion, in line with the accumulation of protein networks observed on BAM images ().
Discussion
Mitochondrial respiratory chain is a major source of reactive oxygen species (ROS); therefore, mitochondrial proteins are a prime target for their damaging effects (Kowaltowski & Vercesi, Citation1999; Marcillat et al., Citation1988; Ott et al., Citation2007). Pathogenesis of many diseases such as cancer, diabetes, ischemia/reperfusion injury, and neurodegenerative disorders, but also aging, implicates mitochondrial oxidative stress-induced dysfunction (Enns, Citation2003; Fariss et al., Citation2005; Sastre et al., Citation2003). Several oxidative stress-related degenerative diseases present energy metabolism dysfunction and are associated to a decrease in CK activity. For instance, an aberrant cytosol/membrane partitioning of CK, as well as CK inactivation are observed in Alzheimer’s disease (David et al., Citation1998), amyotrophic lateral sclerosis (Wendt et al., Citation2002) or Parkinson’s disease (Van Laar et al., Citation2008). In vivo or in vitro studies have shown inhibitory actions of several ROS on mtCK activity (Li et al., Citation2011; Sharma et al., Citation2007; Stachowiak et al., Citation1998). In view of mtCK abundance in mitochondrial intermembrane space and prominent biological role, we focused on the effect of the lipid peroxidation product 4-HNE on the protein activity as well as on the consequences of its association to phospholipid membranes.
We report here two major effects of 4-HNE on mtCK. First, a dose-dependent decrease in mtCK enzymatic activity was observed after incubation with 4-HNE. The inactivation of mtCK by 4-HNE occurred in several steps, which suggests a complex process, where several residues are involved (). The loss of enzymatic activity does not occur instantaneously, even at high 4-HNE: mtCK ratio, which may indicate that some of the 4-HNE sensitive residues are not directly accessible, but embedded in the protein structure. One of the prime target residues is the conserved active site cysteine Cys278 (Furter et al., Citation1993). This residue has been identified as one of the targets for peroxinitrite modifications (Wendt et al., Citation2003). Moreover, the equivalent residue Cys283 in the brain cytosolic isoform of creatine kinase (CK-BB) was shown to be modified by 4-HNE at concentrations as low as 10 μM and this modification induced a decrease in enzyme activity (Eliuk et al., Citation2007). It is clear that the inactivation process of mtCK occurs with a relatively high concentration of 4-HNE (62.5 μM to 1 mM) for 0.5 μM mtCK octamer. However, we have to keep in mind that mtCK octamer contains 608 residues that are susceptible to react with 4-HNE and form adducts (Lys, His, Cys, Arg). In the case of CK-BB, Eliuk et al. effectively showed different levels of residual activity as function of 4-HNE concentration. In their paper, Eliuk et al. obtained a complete enzyme inactivation only when additional His66, His191, and His296 residues from the active site were modified, at higher 4-HNE concentrations (300 μM). Additionally, it is noteworthy that mtCK loses its activity in the absence of reducing agents, so we recorded enzyme inactivation in a dithiothreitol (DTT)-containing medium. The presence of DTT may explain the high level of aldehyde necessary to inactivate mtCK. Of note, Horakova et al. (Citation2002) showed a preventive effect of glutathione generated in rat brain homogenate on CK inactivation by 4-HNE. However, despite the relatively high DTT concentration (200 μM), 4-HNE inactivates mtCK, indicating that even in a complex medium, mtCK is susceptible to be altered by 4-HNE.
The second effect of 4-HNE on mtCK is the formation of oligomers through intermolecular β-sheet structures as deduced from the turbidity increase () and from IR spectra (). This effect occurs quickly, within seconds after 4-HNE addition () for 4-HNE concentrations above 250 μM, and at 4-HNE concentrations much lower than that used for complete enzyme inactivation. It is thus tempting to suppose that 4-HNE generation may result in mtCK assembly and oligomerization and accumulation in the mitochondrial intermembrane space. Moreover, the effects of 4-HNE on mtCK-induced membrane organisation were drastic. MtCK was previously shown to form organized proteo-lipid complexes with CL (Maniti et al., Citation2009b). It was previously suggested that this phenomenon may be related to a structural role of this protein in mitochondria (Lenz et al., Citation2007) and also with an anti-apoptotic role of the protein which contributes to formation of CL-enriched platforms that may regulate other protein-lipid interactions like cytochrome c binding to the mitochondrial inner membrane (Epand et al., Citation2007; Maniti et al., Citation2009b). Although binding of mtCK to the mitochondrial membrane involves Lys residues at the C-term of the protein and is thus mainly electrostatic in nature, we have previously shown using differential capacity measurements that mtCK is able to penetrate CL-containing monolayers. Similar experiments performed with mtCK pre-treated wit 4-HNE showed that the modified enzyme has lost this capacity, although it was still able to bind to membrane surface (). This suggests that 4-HNE-modified mtCK binds the membrane mainly through electrostatic adsorption and the protein loses its capacity to insert into the membrane compared with the untreated mtCK. Although Lys residues in general are known targets for 4-HNE action, the equivalent residues (Lys 358 and Lys 381), in the case of the brain isoform of creatine kinase studied by Eliuk et al. were only modified at very high 4-HNE concentration (5000 μM) which is above those tested here (Eliuk et al., Citation2007). In view of the very high level of sequence and structure similarities in the guanidino kinase family, this would suggest that lysine residues involved in mtCK membrane binding are not modified by 4-HNE.
After 4-HNE action, mtCK binding mechanism is perturbed. MtCK-4-HNE adduct formed large networks below the phospholipid monolayer (). Similar networks were described by Ta et al. (Citation2011) for toxic amyloid variants interacting with negatively charged monolayers, together with formation of antiparallel β-sheet structures. Those two phenomena were associated with a toxic peptide phenotype. 4-HNE was also shown to covalently modify the amyloid β-peptide at multiple locations accelerating the formation of Alzheimer protofibrils (Siegel et al., Citation2007). Other mitochondrial protein aggregation after oxidative stress has been reported in several situations (Hashimoto et al., Citation2003; Marcillat et al., Citation1988). Analysis of muscle biopsy from patients suffering of mitochondrial myopathies revealed the presence of mitochondrial crystalline inclusions in which mtCK octamers were the main component (Stadhouders et al., Citation1994). Moreover, it was also shown that creatine depletion results in accumulation of mtCK within crystalline inclusions in mitochondria of skeletal muscle (O’Gorman et al., Citation1997a, Citation1997b) or cardiomyocytes (Eppenberger-Eberhardt et al., Citation1991). In our results, the particular networks observed on monolayers mimicking the inner mitochondrial membrane could be associated to such pathological manifestations. As it has already been suggested (O’Gorman et al., Citation1997a), CL could be a foundation for mtCK crystallization. Under normal circumstances, mtCK has the capacity to segregate CL and form specific CL-protein complexes. We showed here that 4-HNE oxidative modifications inactivate mtCK and render the protein more prone to form oligomers both in solution and on the lipid monolayer. At the mitochondrial level, loss of mtCK activity and formation of fibril-like networks could lead to energy impairment, loss or modification of mitochondrial structure and eventually to cell death.
Abbreviations | ||
CK | = | creatine kinase |
mtCK | = | mitochondrial creatine kinase |
4-HNE | = | 4-hydroxy-2-nonenal |
CL | = | cardiolipin |
PC | = | phosphatidylcholine |
PE | = | phosphatidylethanolamine |
BAM | = | Brewster angle microscopy |
a.c. | = | alternative current |
C | = | differential capacity. |
Acknowledgements
We thank Prof. Alain Doutheau, Dr Bernard Chantegrel and Dr Christian Deshayes for the kind gift of 4-HNE.
Declaration of interest
The authors report no conflicts of interest. The authors alone are responsible for the content and writing of the paper.
We acknowledge funding from the University Claude Bernard Lyon 1, CNRS and Rhône-Alpes region (Grant number: 04 022407 01).
References
- Bacot S, Bernoud-Hubac N, Baddas N, Chantegrel B, Deshayes C, Doutheau A, et al. 2003. Covalent binding of hydroxy-alkenals 4-HDDE, 4-HHE, and 4-HNE to ethanolamine phospholipid subclasses. J Lipid Res 44:917–926
- Barreiro E, Gea J, Di Falco M, Kriazhev L, James S, Hussain SN. 2005. Protein carbonyl formation in the diaphragm. Am J Respir Cell Mol Biol 32:9–17
- Barth A. 2007. Infrared spectroscopy of proteins. Biochim Biophys Acta 1767:1073–1101
- Butterfield DA, Reed T, Perluigi M, De Marco C, Coccia R, Cini C, Sultana R. 2006. Elevated protein-bound levels of the lipid peroxidation product, 4-hydroxy-2-nonenal, in brain from persons with mild cognitive impairment. Neurosci Lett 397:170–173
- Chen JJ, Bertrand H, Yu BP. 1995. Inhibition of adenine nucleotide translocator by lipid peroxidation products. Free Radic Biol Med 19:583–590
- Chen JJ, Yu BP. 1994. Alterations in mitochondrial membrane fluidity by lipid peroxidation products. Free Radic Biol Med 17:411–418
- David S, Shoemaker M, Haley BE. 1998. Abnormal properties of creatine kinase in Alzheimer’s disease brain: correlation of reduced enzyme activity and active site photolabeling with aberrant cytosol-membrane partitioning. Brain Res Mol Brain Res 54:276–287
- Ducharme D, Max JJ, Salesse C, Leblanc RM. 1990. Ellipsometric study of the physical states of phosphatidylchoilnes at the air-water interface. J Phys Chem 94:1925–1932
- Eaton P, Li JM, Hearse DJ, Shattock MJ. 1999. Formation of 4-hydroxy-2-nonenal-modified proteins in ischemic rat heart. Am J Physiol 276:H935–943
- Eliuk SM, Renfrow MB, Shonsey EM, Barnes S, Kim H. 2007. Active site modifications of the brain isoform of creatine kinase by 4-hydroxy-2-nonenal correlate with reduced enzyme activity: mapping of modified sites by Fourier transform-ion cyclotron resonance mass spectrometry. Chem Res Toxicol 20:1260–1268
- Enns GM. 2003. The contribution of mitochondria to common disorders. Mol Genet Metab 80:11–26
- Epand RF, Tokarska-Schlattner M, Schlattner U, Wallimann T, Epand RM. 2007. Cardiolipin clusters and membrane domain formation induced by mitochondrial proteins. J Mol Biol 365:968–980
- Eppenberger-Eberhardt M, Riesinger I, Messerli M, Schwarb P, Muller M, Eppenberger HM, Wallimann T. 1991. Adult rat cardiomyocytes cultured in creatine-deficient medium display large mitochondria with paracrystalline inclusions, enriched for creatine kinase. J Cell Biol 113:289–302
- Esterbauer H, Schaur RJ, Zollner H. 1991. Chemistry and biochemistry of 4-hydroxynonenal, malonaldehyde and related aldehydes. Free Radic Biol Med 11:81–128
- Fariss MW, Chan CB, Patel M, Van Houten B, Orrenius S. 2005. Role of mitochondria in toxic oxidative stress. Mol Interv 5:94–111
- Font B, Vial C, Goldschmidt D, Eichenberger D, Gautheron DC. 1983. Effects of SH group reagents on creatine kinase interaction with the mitochondrial membrane. Arch Biochem Biophys 220:541–548
- Fritz-Wolf K, Schnyder T, Wallimann T, Kabsch W. 1996. Structure of mitochondrial creatine kinase. Nature 381:341–345
- Furter R, Furter-Graves EM, Wallimann T. 1993. Creatine kinase – the reactive cysteine is required for synergism but is nonessential for catalysis. Biochemistry 32:7022–7029
- Glasoe PK, Long FA. 1960. Use of glass electrodes to measure acidities in deuterium oxyde. J Phys Chem 64:188–190
- Goormaghtigh E, Cabiaux V, Ruysschaert JM. 1994. Determination of Soluble and Membrane Protein Structure by Fourier Transform Infrared Spectroscopy. New York: Plenum Press
- Granjon T, Vacheron MJ, Vial C, Buchet R. 2001. Mitochondrial creatine kinase binding to phospholipids decreases fluidity of membranes and promotes new lipid-induced beta structures as monitored by red edge excitation shift, laurdan fluorescence, and FTIR. Biochemistry 40:6016–6026
- Guichardant M, Taibi-Tronche P, Fay LB, Lagarde M. 1998. Covalent modifications of aminophospholipids by 4-hydroxynonenal. Free Radic Biol Med 25:1049–1056
- Guzun R, Gonzalez-Granillo M, Karu-Varikmaa M, Grichine A, Usson Y, Kaambre T, et al. 2012. Regulation of respiration in muscle cells in vivo by VDAC through interaction with the cytoskeleton and MtCK within Mitochondrial Interactosome. Biochim Biophys Acta 1818:1545–1554
- Hashimoto M, Rockenstein E, Crews L, Masliah E. 2003. Role of protein aggregation in mitochondrial dysfunction and neurodegeneration in Alzheimer’s and Parkinson’s diseases. Neuromol Med 4:21–35
- Henon S, Meunier J. 1991. Microscope at the Brewster angle: direct observation of first-order phase transitions in monolayers. Rev Sci Instrum 62:936–939
- Horakova L, Ondrejickova O, Vajdova M, Korytar P, Durackova Z, Schaur RJ. 2002. Mechanisms of iron-induced oxidative modifications of creatine kinase in rat brain in vitro. Possible involvement of HNE. Gen Physiol Biophys 21:327–336
- Jackson M, Mantsch HH. 1995. The use and misuse of FTIR spectroscopy in the determination of protein structure. Crit Rev Biochem Mol Biol 30:95–120
- Kowaltowski AJ, Vercesi AE. 1999. Mitochondrial damage induced by conditions of oxidative stress. Free Radic Biol Med 26:463–471
- Kupriyanov VV, Elizarova GV, Saks VA. 1981. Determination of the molar content of creatine-kinase in heart-mitochondria using Sh reagents. Biochemistry-Moscow 46:762–771
- Kuznetsov AV, Saks VA. 1986. Affinity modification of creatine kinase and ATP-ADP translocase in heart mitochondria: determination of their molar stoichiometry. Biochem Biophys Res Commun 134:359–366
- Lecompte MF. 2005. Interaction of an amphitropic protein (factor Xa) with membrane models in a complex system. Biochim Biophys Acta 1724:307–314
- Lecompte MF, Bouix G, Mann KG. 1994. Electrostatic and hydrophobic interactions are involved in factor Va binding to membranes containing acidic phospholipids. J Biol Chem 269:1905–1910
- Lecompte MF, Bras AC, Dousset N, Portas I, Salvayre R, Ayrault-Jarrier M. 1998. Binding steps of apolipoprotein A-I with phospholipid monolayers: adsorption and penetration. Biochemistry 37:16165–16171
- Lecompte MF, Clavilier J, Rolland C, Collet X, Negre-Salvayre A, Salvayre R. 2005. Effect of 4-hydroxynonenal on phosphatidylethanolamine containing condensed monolayer and on its interaction with apolipoprotein A-I. FEBS Lett 579:5074–5078
- Lenz H, Schmidt M, Welge V, Kueper T, Schlattner U, Wallimann T, et al. 2007. Inhibition of cytosolic and mitochondrial creatine kinase by siRNA in HaCaT- and HeLaS3-cells affects cell viability and mitochondrial morphology. Mol Cel Biochem 306:153–162
- Li C, Sun SQ, Park D, Jeong HO, Chung HY, Liu XX, Zhou HM. 2011. Hydrogen peroxide targets the cysteine at the active site and irreversibly inactivates creatine kinase. Int J Biol Macromol 49:910–916
- Maniti O, Cheniour M, Marcillat O, Vial C, Granjon T. 2009a. Morphology modifications in negatively charged lipid monolayers upon mitochondrial creatine kinase binding. Mol Membr Biol 26:171–185
- Maniti O, Lecompte MF, Marcillat O, Desbat B, Buchet R, Vial C, Granjon T. 2009b. Mitochondrial creatine kinase binding to phospholipid monolayers induces cardiolipin segregation. Biophys J 96:2428–2438
- Maniti O, Lecompte MF, Marcillat O, Vial C, Granjon T. 2010. Mitochondrial creatine kinase interaction with cardiolipin-containing biomimetic membranes is a two-step process involving adsorption and insertion. Eur Biophys J 39:1649–1655
- Marcillat O, Goldschmidt D, Eichenberger D, Vial C. 1987. Only one of the two interconvertible forms of mitochondrial creatine kinase binds to heart mitoplasts. Biochim Biophys Acta 890:233–241
- Marcillat O, Perraut C, Granjon T, Vial C, Vacheron MJ. 1999. Cloning, Escherichia coli expression, and phase-transition chromatography-based purification of recombinant rabbit heart mitochondrial creatine kinase. Prot Expr Purif 17:163–168
- Marcillat O, Zhang Y, Lin SW, Davies KJ. 1988. Mitochondria contain a proteolytic system which can recognize and degrade oxidatively-denatured proteins. Biochem J 254:677–683
- Markesbery WR, Lovell MA. 1998. Four-hydroxynonenal, a product of lipid peroxidation, is increased in the brain in Alzheimer’s disease. Neurobiol Aging 19:33–36
- Marsh D. 1996. Lateral pressure in membranes. Biochim Biophys Acta 1286:183–223
- McGrath LT, McGleenon BM, Brennan S, McColl D, McIlroy S, Passmore AP. 2001. Increased oxidative stress in Alzheimer’s disease as assessed with 4-hydroxynonenal but not malondialdehyde. QJM 94:485–490
- Miller K, Sharer K, Suhan J, Koretsky AP. 1997. Expression of functional mitochondrial creatine kinase in liver of transgenic mice. Am J Physiol 272:C1193–1202
- Nadkarni DV, Sayre LM. 1995. Structural definition of early lysine and histidine adduction chemistry of 4-hydroxynonenal. Chem Res Toxicol 8:284–291
- O’Gorman E, Fuchs KH, Tittmann P, Gross H, Wallimann T. 1997a. Crystalline mitochondrial inclusion bodies isolated from creatine depleted rat soleus muscle. J Cell Sci 110:1403–1411
- O’Gorman E, Piendl T, Muller M, Brdiczka D, Wallimann T. 1997b. Mitochondrial intermembrane inclusion bodies: the common denominator between human mitochondrial myopathies and creatine depletion, due to impairment of cellular energetics. Mol Cell Biochem 174:283–289
- Ott M, Gogvadze V, Orrenius S, Zhivotovsky B. 2007. Mitochondria, oxidative stress and cell death. Apoptosis 12:913–922
- Pillon NJ, Croze ML, Vella RE, Soulere L, Lagarde M, Soulage CO. 2012. The lipid peroxidation by-product 4-hydroxy-2-nonenal (4-HNE) induces insulin resistance in skeletal muscle through both carbonyl and oxidative stress. Endocrinology 153:2099–2111
- Poli G, Schaur RJ. 2000. 4-Hydroxynonenal in the pathomechanisms of oxidative stress. IUBMB Life 50:315–321
- Quemeneur E, Eichenberger D, Vial C. 1990. Immunological determination of the oligomeric form of mitochondrial creatine kinase in situ. FEBS Lett 262:275–278
- Ramanathan R, Mancini RA, Suman SP, Cantino ME. 2012. Effects of 4-hydroxy-2-nonenal on beef heart mitochondrial ultrastructure, oxygen consumption, and metmyoglobin reduction. Meat Sci 90:564–571
- Reed T, Perluigi M, Sultana R, Pierce WM, Klein JB, Turner DM, et al. 2008. Redox proteomic identification of 4-hydroxy-2-nonenal-modified brain proteins in amnestic mild cognitive impairment: insight into the role of lipid peroxidation in the progression and pathogenesis of Alzheimer’s disease. Neurobiol Dis 30:107–120
- Ridgley DM, Claunch EC, Barone JR, Ridgley DM, Claunch EC, Barone JR. 2013. Characterization of large amyloid fibers and tapes with Fourier Transform Infrared (FT-IR) and raman spectroscopy. Appl Spectroscopy 67:1417–1426
- Saks V, Guzun R, Timohhina N, Tepp K, Varikmaa M, Monge C, et al. 2010. Structure-function relationships in feedback regulation of energy fluxes in vivo in health and disease: mitochondrial interactosome. Biochim Biophys Acta 1797:678–697
- Sastre J, Pallardo FV, Vina J. 2003. The role of mitochondrial oxidative stress in aging. Free Radic Biol Med 35:1–8
- Sayre LM, Zelasko DA, Harris PL, Perry G, Salomon RG, Smith MA. 1997. 4-Hydroxynonenal-derived advanced lipid peroxidation end products are increased in Alzheimer’s disease. J Neurochem 68:2092–2097
- Schlame M, Augustin W. 1985. Association of creatine kinase with rat heart mitochondria: high and low affinity binding sites and the involvement of phospholipids. Biomed Biochim Acta 44:1083–1088
- Schlattner U, Gehring F, Vernoux N, Tokarska-Schlattner M, Neumann D, Marcillat O, et al. 2004. C-terminal lysines determine phospholipid interaction of sarcomeric mitochondrial creatine kinase. J Biol Chem 279:24334–24342
- Schug ZT, Gottlieb E. 2009. Cardiolipin acts as a mitochondrial signalling platform to launch apoptosis. Biochim Biophys Acta 1788:2022–2031
- Sharma AB, Sun J, Howard LL, Williams AG, Mallet RT. 2007. Oxidative stress reversibly inactivates myocardial enzymes during cardiac arrest. Am J Physiol 292:H198–H206
- Siegel SJ, Bieschke J, Powers ET, Kelly JW. 2007. The oxidative stress metabolite 4-hydroxynonenal promotes Alzheimer protofibril formation. Biochemistry 46:1503–1510
- Siems W, Kowalewski J, David H, Grune T, Bimmler M. 1991. Discrepancy between biochemical normalization and morphological recovery of jejunal mucosa during postischemic reperfusion in presence of the xanthine oxidase inhibitor oxypurinol. Cell Mol Biol 37:213–226
- Soulere L, Queneau Y, Doutheau A. 2007. An expeditious synthesis of 4-hydroxy-2E-nonenal (4-HNE), its dimethyl acetal and of related compounds. Chem Phys Lipids 150:239–243
- Stachowiak O, Dolder M, Wallimann T, Richter C. 1998. Mitochondrial creatine kinase is a prime target of peroxynitrite-induced modification and inactivation. J Biol Chem 273:16694–16699
- Stadhouders AM, Jap PHK, Winkler HP, Eppenberger HM, Wallimann T. 1994. Mitochondrial creatine kinase: a major constituent of pathological inclusions seen in mitochondrial myopathies. Proc Natl Acad Sci USA 91:5089–5093
- Ta HP, Berthelot K, Coulary-Salin B, Desbat B, Gean J, Servant L, et al. 2011. Comparative studies of nontoxic and toxic amyloids interacting with membrane models at the air-water interface. Langmuir 27:4797–4807
- Van Kuijk FJ, Thomas DW, Stephens RJ, Dratz EA. 1990. Gas chromatography-mass spectrometry of 4-hydroxynonenal in tissues. Methods Enzymol 186:399–406
- Van Laar VS, Dukes AA, Cascio M, Hastings TG. 2008. Proteomic analysis of rat brain mitochondria following exposure to dopamine quinone: implications for Parkinson disease. Neurobiol Dis 29:477–489
- Vernoux N, Granjon T, Marcillat O, Besson F, Vial C. 2006. Interfacial behavior of cytoplasmic and mitochondrial creatine kinase oligomeric states. Biopolymers 81:270–281
- Vernoux N, Maniti O, Besson F, Granjon T, Marcillat O, Vial C. 2007. Mitochondrial creatine kinase adsorption to biomimetic membranes: a Langmuir monolayer study. J Colloid Interf Sci 310:436–445
- Vernoux N, Maniti O, Marcillat O, Vial C, Granjon T. 2009. Mitochondrial creatine kinase interaction with heterogeneous monolayers: effect on lipid lateral organization. Biochimie 91:752–764
- Vial C, Marcillat O, Goldschmidt D, Font B, Eichenberger D. 1986. Interaction of creatine kinase with phosphorylating rabbit heart mitochondria and mitoplasts. Arch Biochem Biophys 251:558–566
- Vollhardt D. 1996. Morphology and phase behavior of monolayers. Adv Colloid Interface Sci 64:143–171
- Wendt S, Dedeoglu A, Speer O, Wallimann T, Beal FM, Andreassen OA. 2002. Reduced creatine kinase activity in transgenic amyotrophic lateral sclerosis mice. Free Radic Biol Med 32:920–926
- Wendt S, Schlattner U, Wallimann T. 2003. Differential effects of peroxynitrite on human mitochondrial creatine kinase isoenzymes. Inactivation, octamer destabilization, and identification of involved residues. J Biol Chem 278:1125–1130
- Yin H, Zhu M. 2012. Free radical oxidation of cardiolipin: chemical mechanisms, detection and implication in apoptosis, mitochondrial dysfunction and human diseases. Free Radic Res 46:959–974
- Zarkovic K. 2003. 4-hydroxynonenal and neurodegenerative diseases. Mol Aspects Med 24:293–303