Abstract
The distribution, pharmacology and function of the arginine vasopressin (Avp) 1b receptor subtype (Avpr1b) has proved more challenging to investigate compared to other members of the Avp receptor family. Avp is increasingly recognised as an important modulator of the hypothalamic–pituitary–adrenal (HPA) axis, an action mediated by the Avpr1b present on anterior pituitary corticotrophs. The Avpr1b is also expressed in some peripheral tissues including pancreas and adrenal, and in the hippocampus (HIP), paraventricular nucleus and olfactory bulb of the rodent brain where its function is unknown. The central distribution of Avpr1bs is far more restricted than that of the Avpr1a, the main Avp receptor subtype found in the brain. Whether Avpr1b expression in rodent tissues is dependent on differences in the length of microsatellite dinucleotide repeats present in the 5′ promoter region of the Avpr1b gene remains to be determined. One difficulty of functional studies on the Avpr1b, especially its involvement in the HPA axis response to stress, which prompted the generation of Avpr1b knockout (KO) mouse models, was the shortage of commercially available Avpr1b ligands, particularly antagonists. Research on mice lacking functional Avpr1bs has highlighted behavioural deficits in social memory and aggression. The Avpr1b KO also appears to be an excellent model to study the contribution of the Avpr1b in the HPA axis response to acute and perhaps some chronic (repeated) stressors where corticotrophin-releasing hormone and other genes involved in the HPA axis response to stress do not appear to compensate for the loss of the Avpr1b.
Introduction
Arginine vasopressin (Avp) is a cyclic nonapeptide that exerts diverse biological effects through a number of receptors (R), three of which have been cloned to date in mammals; the vasopressin Avpr1a, Avpr1b and Avpr2—Avp also binds to the structurally related oxytocin (Oxt) receptor (Oxtr) with high affinity. Each member of the Avp receptor family has a discrete peripheral distribution and function; however, data for the central distribution of these receptors are still incomplete. The Avp receptor family are G protein-coupled receptors: the Avpr1a and Avpr1b subtypes are both coupled to Gq/11 and signal via phospholipase C (Jard et al. Citation1987; Thibonnier et al. Citation2001). The Avpr2 receptor subtype is coupled to Gs which, when activated, elevates cAMP levels by recruiting adenylate cyclase. It should be noted that many G protein-coupled receptors (GPCRs) couple to multiple signal transduction pathways, including the Avpr1b (Thibonnier et al. Citation2001)—this may be related to the number of receptors and signal transduction components expressed in a given cell. The Oxtr predominantly signals via Gq/11 but is a promiscuous receptor in that it may signal through a variety of different α subunits (Reversi et al. Citation2005; Chini and Manning Citation2007). The tissue distribution and physiological function of each receptor demonstrates Avp's primary function in fluid balance and homeostasis. The Avpr1a is predominantly found in vascular smooth muscle where it is involved in maintaining blood pressure via its classical pressor action (Koshimizu et al. Citation2006). The renal Avpr2 is responsible for water resorption in kidney collecting ducts by promoting the translocation of aquaporin-2 channels to the plasma membrane (Knepper Citation1997). The Avpr1b is primarily located in the anterior lobe corticotrophs of the pituitary gland (Jard et al. Citation1986; Lolait et al. Citation1995). Avp in hypophysial portal blood acts on pituitary Avpr1bs to release adrenocorticotrophic hormone (ACTH) as part of the neuroendocrine response to stress (Antoni Citation1993). The contraction of uterine smooth muscle during parturition and milk let down during lactation are actions mediated by Oxt and the Oxtr. The distribution and function of Avp receptors is not limited to those sites mentioned above and all receptors except the Avpr2 are expressed in the brain. The central role each of these receptors plays has not yet been fully elucidated but the growing amount of data from pharmacological and knockout (KO) studies suggests some functional overlap (e.g. in modulating some social behaviour).
The availability of several specific ligands for these receptors has considerably aided in the characterisation of the Avpr1a, Avpr2 and Oxtr but, until recently, research on the Avpr1b has been hampered by a lack of Avpr1b-specific ligands. Avpr1b research has focussed primarily on the detection of receptor transcript levels (indirectly inferring receptor protein levels), genetic KO studies and experiments with the naturally Avp-deficient Brattleboro (di/di) rats. This review details recent pharmacological and KO data on the role of the Avpr1b in brain, pituitary and peripheral tissues with particular emphasis on its function in the hypothalamic–pituitary–adrenal (HPA) axis response to stress.
V1B receptor distribution
The Avpr1a and the Oxtr have been well characterised in the rodent brain and are thought to be the likely substrates for central actions of Avp and/or Oxt (de Wied et al. Citation1993; Burbach et al. Citation1995; Barberis and Tribollet Citation1996). More recent data from KO animals suggest specific behavioural deficits in social memory and aggression are directly due to the absence of central Avpr1bs (CitationWersinger et al. 2002). The search for central Avpr1bs has proved more elusive than that of the Avpr1as and Oxtrs as the shortage of specific ligands has prevented binding studies to visualise the Avpr1b protein. Nevertheless, a number of studies utilising immunohistochemistry and in situ hybridisation histochemistry (ISHH) have shown that the Avpr1b is expressed centrally, while reverse transcription-polymerase chain reaction (RT-PCR) and functional studies have demonstrated Avpr1bs in a number of peripheral tissues (e.g. Lolait et al. Citation1995; Vaccari et al. Citation1998; Hernando et al. Citation2001; O'Carroll et al. Citation2008).
Although the highest concentration of Avpr1bs is found within anterior pituitary corticotrophs (Jard et al. Citation1986; Antoni Citation1993; Lolait et al. Citation1995), several studies suggest a wide central (Barberis and Tribollet Citation1996; Vaccari et al. Citation1998; Hernando et al. Citation2001) and peripheral (Lolait et al. Citation1995; Saito et al. Citation1995; Ventura et al. Citation1999; Oshikawa et al. Citation2004) distribution in rodents. Analysis of various brain regions and peripheral tissues suggests that Avpr1b transcript levels may be too low to be reliably detected by Northern blot analysis and often depends on RT-PCR to detect possible Avpr1b expression (Lolait et al. Citation1995). A recent distribution study using ISHH with probes directed to 5′ or 3′ untranslated regions of the Avpr1b mRNA details a more restricted pattern of Avpr1b mRNA in mouse brain than previously reported (Young et al. Citation2006). The riboprobes used by Young and co-workers had low sequence identity to other Avp receptors to minimise cross-reactivity with related mRNA sequences. This study shows Avpr1b mRNA to be most prominent in the CA2 pyramidal neurons of the mouse and human HIP while receptor transcripts are also found in the paraventricular nucleus (PVN) and amygdala, albeit at a lower level. All of these studies infer receptor expression by determining mRNA transcript levels rather than receptor protein levels. However, in the absence of specific radiolabelled ligands of high-specific activity (which may not provide detailed anatomical resolution), mRNA expression coupled with immunohistochemical techniques can accurately reflect receptor protein distribution and quantity.
The peripheral distribution of the Avpr1b is much more restricted than that of the Avpr1a, which is ubiquitously expressed (Oshikawa et al. Citation2004; Fujiwara et al. Citation2007a). Avpr1b mRNA has been detected by RT-PCR in the rodent pancreas (Saito et al. Citation1995; Ventura et al. Citation1999; Oshikawa et al. Citation2004), adrenal gland (Grazzini et al. Citation1996; Ventura et al. Citation1999; Oshikawa et al. Citation2004), spleen (Lolait et al. Citation1995; Oshikawa et al. Citation2004), kidney (Lolait et al. Citation1995; Saito et al. Citation1995), heart (Lolait et al. Citation1995; Saito et al. Citation1995), liver (Saito et al. Citation1995) and lung (Lolait et al. Citation1995; Saito et al. Citation1995). Additionally, the thymus (Lolait et al. Citation1995), colon (Ventura et al. Citation1999), small intestine, bladder (Saito et al. Citation1995), breast and uterus (Lolait et al. Citation1995) and white adipose tissue (Fujiwara et al. Citation2007a) reportedly contain Avpr1b mRNA, however, these have not been ratified by all studies (e.g. only one out of five studies observe Avpr1b mRNA in rodent liver). In any event, the functional significance of varying amounts of low levels of Avpr1b mRNA detected by RT-PCR in whole brain or peripheral tissue samples is unknown. Disparities between laboratories may reflect methodological (e.g. detection of amplified PCR products) and/or strain differences. The mRNA-expressing tissues where there appears to be strong functional correlations are the pancreas and adrenal gland.
In the pancreas, Avp has been shown to act on Avpr1bs present in islets to stimulate the secretion of insulin from β cells where it may act synergistically with corticotrophin-releasing hormone (Crh) (Oshikawa et al. Citation2004; O'Carroll et al. Citation2008). While Avp acts on the Avpr1b to decrease blood sugar levels through insulin release, it can also act in opposition to this by stimulating glucagon release (Yibchok-anun and Hsu Citation1998) and promoting hepatic glycogenolysis (Kirk et al. Citation1979). Importantly, Avp-mediated glycogenolysis acts via the Avpr1a subtype present in hepatic tissue (Morel et al. Citation1992) suggesting bifunctional but opposite roles of Avp in glucose homeostasis by employing two different receptor subtypes. Exactly which role Avp plays in regulating glucose balance at the pancreatic level is dependent on the local glucose concentration present in this tissue (Abu-Basha et al. Citation2002). One study on a glucagon-secreting hamster α-pancreatic cell line suggests that Avp-induced glucagon secretion is mediated via the Avpr1b since the Avpr1b antagonist SSR149415 potently antagonises Avp's effects in these cells (Folny et al. Citation2003). The specificity of SSR149415 has, however, been questioned with evidence of activity in Chinese hamster ovary cell lines expressing recombinant human Oxtrs (Griffante et al. Citation2005; Hodgson et al. Citation2007). This may be an important consideration, as Oxtrs in addition to Avpr1bs are apparently present in pancreatic islets (Oshikawa et al. Citation2004) and have been shown to cause insulin/glucagon release (Jeng et al. Citation1996; Yibchok-anun et al. Citation1999). One laboratory that used agonists and antagonists to Avp/Oxt receptors (but notably none selective for Avpr1bs) in α-cell lines (Yibchok-anun and Hsu Citation1998) and perfused rat pancreas (Yibchok-anun et al. Citation1999) produced contrasting results, the latter study suggesting glucagon secretion in response to Avp, and Oxt is mediated through the activation of Avpr1bs and Oxtrs, respectively, rather than any activity of Avp on Oxtrs. On the other hand, studies in Avpr1b KO mice suggest both Avp and Oxt can stimulate glucagon secretion through Oxtrs (Fujiwara et al. Citation2007b). Avp may play a role in the hypersecretion of glucagon from the pancreas of diabetics (Yibchok-anun et al. Citation2004), which is likely to involve the Avpr1b. Pancreatic β cells isolated from mice lacking functional Avpr1bs unsurprisingly display a blunted insulin secretion (Oshikawa et al. Citation2004), reinforcing the role of Avpr1bs in this tissue. Interestingly, further studies with this Avpr1b KO line show an increased sensitivity of Avpr1b KO mice to the metabolic effects of insulin (Fujiwara et al. Citation2007a). Together with the discovery of Avpr1b mRNA by RT-PCR in white adipose tissue, Fujiwara and co-workers suggest that the disruption in insulin–adipocyte signalling may lead to altered metabolism of glucose in Avpr1b KO mice. Whether this is due to a lack of Avpr1b influence in the pancreas, white adipose tissue, or both, is unclear.
Grazzini and co-workers demonstrated the presence of Avpr1b mRNA by RT-PCR in the medulla but not in the cortex of the rat adrenal gland. The cortex expresses Avpr1a transcripts primarily in the zona glomerulosa (Guillon et al. Citation1995; Grazzini et al. Citation1996) where this receptor regulates steroid secretion in vitro (Grazzini et al. Citation1998). These studies also show that Avp precursor mRNA and Avp peptide are present in the adrenal medulla suggesting Avp can be released within the tissue, possibly acting in an autocrine/paracrine manner to regulate adrenal function. Stimulation of the Avpr1b in the rat adrenal medulla causes catecholamine secretion (Grazzini et al. Citation1998). The presence of Avpr1as in the adrenal cortex and Avpr1bs in the chromaffin cells of the medulla provides strong evidence of an independent modulatory role of each receptor in discrete regions of adrenal tissue. The presence, however, of both Avpr1s in the human (Grazzini et al. Citation1999) adrenal medulla indicates a possible co-expression of Avpr1 receptors in some species. This suggests a possible overlap of function distinct from the roles already noted and may reflect the action of Avp originating from different sources (e.g. pituitary vs. local tissue release (CitationGallo-Payet and Guillon 1998)), although the medullary cell type that expresses Avpr1as has yet to be identified. Notably, the plasma catecholamine response to forced swimming and social isolation stress is attenuated in Avpr1b KO mice (Itoh et al. Citation2006).
The central, pituitary and peripheral expression of the Avpr1b gene may be influenced by activity of elements in the upstream Avpr1b promoter region. In vitro studies using cells transiently transfected with a rat Avpr1b gene promoter sequence have identified regulatory GAGA repeats that influence Avpr1b gene transcription (Volpi et al. Citation2002). This provides a possible mechanism of physiological Avpr1b gene regulation that may enable different levels of Avpr1b expression in different tissues or species. When we compared the 5′ microsatellite region in the 5′ Avpr1b promoter sequence of different mouse strains, a major size difference in microsatellite length between the C57BL6J/OlaHsd strain and Balb/cOlaHsd and 129S2/SvHsd strains was observed (see, ). Further analysis of the sequence details differences in the number of CT and CA repeats between strains (see, ) that may confer changes in promoter activity. Basal promoter activity of a Balb/c 5′ fragment is threefold greater than that of the C57BL/6 strain confirming an increase in Avpr1b promoter activity with the “long” form of microsatellite (see, ), in a reporter assay in COS-7 cells. The impact of microsatellite DNA sequences on receptor expression and behavioural phenotypes has been examined in studies on the effects of Avpr1a expression on social behaviour in voles. Affiliative behaviours such as pair bonding have been attributed to changes in Avpr1a expression patterns caused by microsatellite length variations in the 5′ Avpr1a regulatory region (Hammock and Young Citation2002; Hammock and Young Citation2005). Differences in Avpr1b protein expression that result from variances in gene promoter activity between mouse strains may be a contributing factor to varying susceptibility to stress. Several neurogenic, psychogenic and systemic stressors have been tested in different strains of mice to reveal a strain-dependant stress response (Anisman et al. Citation2001). Interestingly, C57BL/6ByJ mice display higher levels of plasma CORT as well as increases in stress-related behaviours compared to the Balb/cByJ strain, strengthening support for Avpr1b's involvement in mouse stress susceptibility (Anisman et al. Citation2001). It is important to note, however, that similar 5′ microsatellite sequences are not present in the human Avpr1b gene.
Figure 1. (A) PCR analysis of the microsatellite region in the 5′ promoter of the mouse Avpr1b gene using genomic DNA from three different mouse strains (1, 2, Balb/cOlaHsd; 3, 4, 129S2/SvHsd; 5, 6, C57BL/6JOlaHsd). The PCR products were generated using 100 ng genomic DNA, 0.5 units of AmpliTaq polymerase (Applied Biosystems, Warrington, UK) and primers: upstream 5′ GCG AGC TCT TTC ACA CAT GCC TAG G 3′ incorporating SacI restriction site (underlined); downstream 5′ CAG GAT CCA CTG AGC ACC AAC TCA C 3′ incorporating a BamH1 restriction site (underlined) with cycling conditions of 95°C 1 min followed by 40 cycles of 94°C for 1 min, 62.5°C 1 min, 72°C 30 s followed by a final 72°C step for 10 min and a 4°C soak. Gel electrophoresis (2% agarose) of a 15-μl sample from the 50-μl reaction volume revealed a 301 bp product (bp1755–2055) with Balb/cOlaHsd and 129S2/SvHsd DNA templates and a ∼186 bp product with C57BL6J/OlaHsd strain DNA template. The PCR products were subcloned into pGEM4Z vector using Bam-H1 and Sac-1 restriction enzymes and sequenced. (B) Alignment of 129SV and C57BL strain microsatellite sequences between bases 1801 and 1988 of Genbank Acc#AF152533 (ending 824 bases upstream of the initiating ATG codon). Microsatellite CA and CT repeats are highlighted with dashes representing nucleotides that are absent in the C57BL mouse 5′ promoter region that gives rise to the shorter sequence. The longer form is present in 129/Svj, J1, SWR/J, AKR/J, FVB and CD1 (USA) 129S2/SvHsd and Balb/cOlaHsd (UK) strains, whereas the shorter form is in C57BL/6Ncr (USA), C57BL/6JOlaHsd (UK) and C57BL/6J (USA) strains. (C) Fragments of 5′ Avpr1b gene promoter region incorporating the microsatellite repeats were generated by PCR using 100 ng genomic DNA, Herculase II Fusion DNA polymerase (Stratagene (Agilent Technologies), Stockport, UK), and primers corresponding to a ∼1.1 kb region of the mouse Avpr1b gene (from bp1755–bp2823, Genbank accession number AF152533) using genomic DNA extracted from the Balb/c, C57BL/6 and 129 Sv mouse strains (Harlan, Bicester, UK). Amplified fragments from each strain were then subcloned into a pGL3 basic dual-luciferase reporter assay system (Promega, Southampton, UK) for expression in COS-7 cells. Values (mean ± SEM) are expressed as a percentage of control pGL3 basic activity. The promoter activity of vectors containing Balb/c and 129 Sv constructs is ∼3 × greater than that of C57BL/6 (*** = P < 0.001). When the Balb/c microsatellite region was replaced with the C57BL/6 microsatellite sequence in the Balb/c reporter construct, activity diminished to that of the C57BL/6 strain.
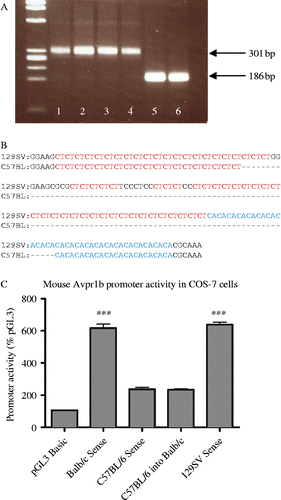
The relatively recent emergence of data from genetic KO studies and the development of promising pharmacological compounds have given the task of characterising the function of central and peripheral Avpr1b renewed vigour. Avpr1b KO mice together with the long-standing subject of Avp research, the Brattleboro rat, serve as robust systems with which to study the role of the Avpr1b and Avp in the HPA axis response to stress.
The HPA axis and stress
The complex homeostatic control that constantly acts to resist challenges and fluctuations in the internal environment that may threaten the survival of an organism is necessary for life. As a result of any deviation in conditions, a host of physiological and behavioural changes occur that allow an organism to adapt to such challenges and restore the homeostatic balance. One such neuroendocrine system that is activated in stressful circumstances is the HPA axis. The end product of HPA axis activation is an increase in circulating glucocorticoids that, together with other stress mediators, act on target cells to enable the organism to cope with the stress. Consequences of elevated glucocorticoids are widespread as cytosolic glucocorticoid receptors are present in most central and peripheral tissues. The most profound effects of elevated glucocorticoid levels are immunological and metabolic changes. Glucocorticoid secretion, in concert with catecholamine release due to rapidly activated sympatho-adrenomedullary system, prepares tissues for the physical “load” that may be required by the body as part of a coordinated response to manage or tackle the stress.
The HPA response to stress relies on several key mediators that fine-tune glucocorticoid release, which is dependent on a number of factors such as the nature of the stressor and the immunological state of the organism, tailoring the response to the specific stressor concerned. When subjected to a stressor, the challenge is perceived in brain regions appropriate to the nature of the stressor. Interpretation of these signals in relevant brain areas, such as various limbic or hindbrain regions, leads to activation (or inhibition) of the PVN (Herman et al. Citation2003).
The parvocellular subdivision of the PVN (pPVN) is the most important site among several hypothalamic nuclei that regulate the HPA response, as this is where stress signals are integrated and adjusted before peripheral signalling is initiated. Once activated, pPVN projections that terminate in the external zone of the median eminence release Avp and Crh into the hypophysial portal blood (Antoni Citation1993). These two ACTH secretagogues act on Avpr1bs and Crh type 1 receptors (Crhr1; coupled to Gs and adenylate cyclase activation) present in pituitary corticotrophs causing the release of ACTH into the peripheral blood system. ACTH via melanocortin receptors present in zona fasciculata cells of the adrenal cortex stimulates a rapid secretion of glucocorticoids into the peripheral blood supply. Glucocorticoids such as corticosterone ((CORT) in rodents) and cortisol (in humans) in turn provide negative feedback control of the HPA axis via pituitary, pPVN and higher brain centre glucocorticoid receptors (e.g. HIP).
The dominance of Crh as the primary ACTH secretagogue is still the prevailing view; however, numerous direct and indirect neuronal inputs into the pPVN, as well as humoral influences from blood or cerebrospinal fluid-borne stress signals, dynamically modulate the contribution of Crh to the stress response. The influence of Avp/Avpr1b may be of greater significance than that of Crh/Crhr1 in some stress circumstances, such as in response to some chronic (repeated) stressors (Ma et al. Citation1999) or to novel stressors superimposed on a repeated stress paradigm (Ma et al. Citation1997). Evidence of a switch from Crh-ergic to vasopressinergic pPVN drive in response to some chronic stressors reinforces the concept that each response is tailored to that specific stressor and that Avp may be an increasingly important mediator in these circumstances (Aguilera Citation1994; Ma et al. Citation1999; Aguilera et al. Citation2008). Moreover, studies of some specific acute stressors indicate that Avp may be preferentially released in favour of Crh (e.g. insulin-induced hypoglycaemia (IIH) in rats (Plotsky et al. Citation1985), ketamine anaesthesia and IIH in sheep (Engler et al. Citation1989)). It is important to emphasise that in some species (e.g. sheep and horse) Avp rather than Crh appears to be the main ACTH secretagogue (Engler et al. Citation1989; Alexander et al. Citation1997).
V1B receptor KO mice
With the generation of Avpr1b KO mice by us in 2002, attention was initially directed towards the deficits observed in cognitive and behavioural tests (CitationWersinger et al. 2002; Wersinger et al. Citation2004). It was noted that mice lacking functional Avpr1bs showed impairments in social recognition and reduced responses in some aggression paradigms whereas other physiological and behavioural test responses were normal (CitationWersinger et al. 2002, Citation2004, Citation2007a). Findings from a second Avpr1b KO line generated by Tanoue and co-workers initially focussed on the disruption of the HPA axis (Tanoue et al. Citation2004), and they reported that KO mice have lower circulating ACTH levels under basal and acute stress conditions. This is in contrast to basal measurements of HPA axis activity seen in our Avpr1b KO mouse colony, which maintain normal resting ACTH levels (Lolait et al. Citation2007a). Notwithstanding basal HPA axis differences, both Avpr1b KO lines have been used to generate a large amount of compatible evidence supporting the involvement of the Avpr1b in stress and aggression, which is also largely consistent with in vivo Avpr1b antagonism with SSR149415 (Griebel et al. Citation2002; CitationBlanchard et al. 2005; Stemmelin et al. Citation2005).
Reduced aggression in KO mice
Avp has been implicated as a moderator of several central behaviours that were initially thought, based on pharmacological profiles and due to its much higher prevalence in the brain, to be mediated by the Avpr1a. Experiments in rodents, particularly hamsters, with Avpr1a antagonists have consistently shown that Avpr1as facilitate some aggressive behaviour (Ferris et al. Citation1997, Citation2006; Caldwell and Albers Citation2004), although this is yet to be verified in Avpr1a KO mutants (Wersinger et al. Citation2007b). It is possible that the neural circuitry underlying aggression compensates for the loss of the Avpr1a in the global Avpr1a KO. In contrast, evidence of Avpr1b involvement in aggression comes from both pharmacological and KO data, e.g. antagonism of the Avpr1b with SSR149415 lowers the frequency and duration of aggressive behaviour in hamsters (CitationBlanchard et al. 2005) while Avpr1b KO mice display reduced attack number and longer attack latency compared to wild types. This latter observation has been further categorised as a deficit in the attack component of aggression, as defensive aggression remains intact in mutant animals (CitationWersinger et al. 2002, Citation2007a). Furthermore, the reduced aggression phenotype persists when Avpr1b KO mice are crossed with a more aggressive wild-derived mouse strain, confirming that the reduced aggression observed is not simply a peculiarity of laboratory mouse strains (Caldwell and Young Citation2009). The specific neural substrate(s) vital for Avpr1b's role in aggression is not known, nor is the possible interaction between Avpr1a and Avpr1b (or Oxt and the Oxtr for that matter—see, Winslow et al. Citation2000) in vivo. It should be noted that the central distribution of Avpr1a- and Oxtr-binding sites and Avpr1b mRNA expression are clearly distinct but may overlap in some brain regions (e.g. olfactory system) (see, in Beery et al. Citation2008; Caldwell et al. Citation2008a).
The changes in aggression, as well as differences in social motivation (Wersinger et al. Citation2004) or social memory evident in Avpr1b KO mice may be due to deficits in the processing of accessory olfactory stimuli that are needed to evoke such behaviours (Caldwell et al. Citation2008b). It is suggested that the role of central Avpr1bs may be to couple socially relevant cues detected in the accessory olfactory system to the appropriate behavioural response (Caldwell et al. Citation2008b). Intriguingly, both Avpr1a and Avpr1b genes are expressed in the forebrain olfactory system (Ostrowski et al. Citation1994; Hernando et al. Citation2001). Evidence of pyramidal CA2 Avpr1bs (Hernando et al. Citation2001; Young et al. Citation2006) also suggests a relationship between the deficits in social memory and the uncoupling of social cues from the accessory olfactory system and the formation or recall of relevant memories (Caldwell et al. Citation2008b). Based on studies in Avpr1b KO animals, the central Avpr1b may also be involved in a number of other behaviours (summarised in ). Prepulse inhibition of the startle reflex is attenuated in Avpr1b KO mice (Egashira et al. Citation2005) suggesting that this mouse may be a suitable model to investigate sensorimotor gating. In contrast, no major changes in anxiety-like or depression-like behaviours are observed in Avpr1b KO animals (CitationWersinger et al. 2002; Egashira et al. Citation2005; Caldwell et al. Citation2010). These results conspicuously differ from some of those obtained in Brattleboro rats (Mlynarik et al. Citation2007) or with Avpr1b antagonist administration, mainly in rats (see below), and strongly suggest that the Avpr1b KO mouse is not an appropriate model for examining stress-induced anxiety or depression (e.g. see Kalueff et al. Citation2007).
Table I. Behavioural studies conducted in Avpr1b KO and wild-type mice that suggest central participation of the Avpr1b.
The stress response in KO mice
The impact of Avp in ACTH release is often regarded as ancillary to Crh as Avp alone is a weak ACTH secretagogue but together with Crh it acts synergistically to facilitate ACTH secretion (Gillies et al. Citation1982; Rivier and Vale Citation1983; Antoni Citation1993). Not only do the corticotroph Avpr1b- and Crh-signalling pathways converge to increase ACTH release (Abou-Samra et al. Citation1986), the two receptors may physically heterodimerise (Young et al. Citation2007). We, and others, have subjected adult Avpr1b KO animals to a number of acute and chronic (repeated) stressors of varying severity and nature (Tanoue et al. Citation2004; Itoh et al. Citation2006; Lolait et al. Citation2007a,Citationb; Stewart et al. Citation2008a,Citationb). These studies clearly demonstrate that a functional Avpr1b is essential for mounting a normal HPA response, as manifested by increased plasma ACTH levels, to most acute stressors (summarised in ). The one exception is the response to “severe” restraint where there is no difference in either plasma ACTH level or CORT level between Avpr1b KO and wild-type mice (Lolait et al. Citation2007a). Studies in “severely” restrained Brattleboro rats reveal a similar picture (Zelena et al. Citation2004). It is likely that the restraint procedure employed was sufficiently stressful as to override any contribution from Avp (acting via the Avpr1b), e.g. ACTH secretion may be entirely dependent on Crh acting alone or in concert with other “minor” ACTH secretagogues such as angiotensin II, vasoactive intestinal peptide or serotonin (Carrasco and Van de Kar Citation2003). When comparing the reduced HPA axis response to other acute stressors in Avpr1b KO mice, we consistently find a decreased ACTH response but not always a corresponding lower CORT level. The nature of stressors to which both ACTH and CORT, ACTH but not CORT and neither ACTH or CORT responses are reduced in Avpr1b KO mice does not fall into any existing classification of stress. This work is in agreement with experiments in Brattleboro rats which suggest that the magnitude of Avp contribution is dependent on the context of the stressor (Zelena et al. Citation2009). As shown in , we find some acute and chronic stressors are not influenced by the loss of the Avpr1b (e.g. acute severe restraint), some have a reduced ACTH response only (e.g. acute forced swimming stress) and some have both a reduced ACTH and CORT response in Avpr1b KOs compared to wild types (e.g. acute and repeated novel environment stress). Thus, how Avp influences the HPA axis response to stress could provide a further basis of stressor classification.
Table II. A summary of our recent studies with the Avpr1b KO colony demonstrating the effects of a number of stressors on plasma ACTH and CORT levels in wild type vs. Avpr1b KO mice.
Our studies in Avpr1b KO mice (Lolait et al. Citation2007a,Citationb; Stewart et al. Citation2008a,Citationb) and similar studies on acute stress in Brattleboro rats (Domokos et al. Citation2008; Zelena et al. Citation2009) both often note a disparity between stress-induced ACTH versus CORT release in Avp/Avpr1b-deficient animals. It is important to recognise that the CORT response to ACTH saturates at low circulating ACTH levels (Dallman et al. Citation2002). In addition, plasma hormones levels were only measured at one time point in our studies so any incremental change in CORT levels may have been missed. Furthermore, we have assumed that the dynamics of stress-induced Avp, Crh and/or other ACTH secretagogue is similar in Avpr1b and wild-type mice. Where stress-induced ACTH release in Avpr1b KO mice is not always followed by a proportional CORT attenuation, suggests that CORT may be released independently of ACTH in some circumstances. Interestingly, this pattern of ACTH/CORT profiles in response to acute stress also appears to be present in neonatal Brattleboro rats (Zelena et al. Citation2008). Discrepancies between plasma stress hormone levels appear stressor specific suggesting that a particular stressor may possess specific characteristics that could activate a number of pathways to promote CORT release, e.g. via direct splanchnic or other neural innervation of the adrenal cortex or medulla (Ehrhart-Bornstein et al. Citation1998). Alternatively, adrenal sensitivity to locally synthesised or humoral factors may be altered permitting CORT release even when low levels of circulating ACTH are present. Adrenal hypersensitivity and ACTH-independent pathways of CORT release would likely bypass the normal feedback controls that govern ACTH release from the pituitary (for review see Bornstein et al. Citation2008).
As mentioned above, chronic (repeated) stress has often been associated with an alteration in the control of ACTH release from what is a predominantly Crh-mediated drive, seen in acute stress, to an Avp-mediated drive speculated to maintain ACTH levels during adaptation to repeated stress (Harbuz and Lightman Citation1992; Aguilera Citation1994; Ma et al. Citation1997; Aguilera and Rabadan-Diehl Citation2000). Adaptation to repeated stress (i.e. lower ACTH and/or CORT following repeated stress compared to a single episode of acute stress) is species and stressor specific and is not always observed (Marti and Armario Citation1998; Armario et al. Citation2004). The apparent flip in control of ACTH release from Crh to Avp that is seen in some repeated stressors in rats (e.g. restraint) has made Avp and the Avpr1b attractive targets for pharmacological intervention in conditions of repeated or chronic stress, although it should be noted that Avp does not play a role in HPA axis responses to all chronic stressors (e.g. chronic morphine injection (Domokos et al. Citation2008)). The preferential expression of Avp in the pPVN in adaptation to chronic stress observed in rats is associated with an upregulation of Avpr1bs (but not Crh receptors) in the anterior pituitary gland (Aguilera Citation1994). Chronic stress from repeated (daily) exposure to an acute stressor leading to ACTH hyperresponsiveness to a single, novel stressor episode may also involve an increase in PVN Avp (Ma et al. Citation1999) and pituitary Avpr1b expression, and pituitary ACTH hyperresponsiveness (Aguilera Citation1994). However, Avp/Avpr1b does not appear to be responsible for HPA axis hypersensitivity to novel stressors (Chen et al. Citation2008). The mechanism(s) by which hypothalamic Avp and pituitary Avpr1b responsiveness is maintained during stress adaptation suggests the existence of numerous transcriptional and translational regulatory components involved in PVN and pituitary plasticity that dynamically alter Avp and Avpr1b levels according to demand (Volpi et al. Citation2004a,Citationb). One view is that Avp/Avpr1b may alter corticotroph proliferation and pituitary remodelling during prolonged activation of the HPA axis (Subburaju and Aguilera Citation2007). However, studies of repeated stress in Avpr1b KO mice and Brattleboro rats, suggest that the role of the Avpr1b and its cognate ligand in the adaptation of ACTH/CORT levels to chronic stress may not be as convincing as first thought.
For the chronic (repeated) stressors tested in Avpr1b KO mice, there is a reduction in the ACTH response to a final acute stress following 10–14 days of stress repeated once daily. The responses of Avpr1b KO animals and wild-type animals exposed to repeated stress are summarised in . These studies have a number of salient features: firstly, as observed in male Brattleboro rats subjected to repeated restraint (Zelena et al. Citation2004), the reduction in the ACTH response following repeated stress in Avpr1b KO animals is not often accompanied by a similar reduction in CORT responses—this mirrors what we have observed in these animals' responses to acute stress (see above). Secondly, with the exception of the ACTH response to repeated, severe restraint, the ACTH and CORT responses to acute or repeated stress are of equivalent magnitude. This suggests that the Avpr1b participates in the fast ACTH secretion (as seen in the response to acute stress) in repeatedly stressed mice. And finally, of all the repeated stressors studied in wild-type mice from our Apr1b KO colony, adaptation in ACTH responses was only seen with repeated exposure to shaker stress (SS) (). No adaptation in CORT responses was observed in Avpr1b or wild-type mice following any repeated stress paradigm. There is a robust plasma ACTH and CORT increase in response to a single, acute SS episode in wild-type mice; however, after 10 days of repeated SS, the ACTH response is reduced in these animals (see, —graph A: plasma ACTH levels, wild-type acute stress response vs. wild-type chronic stress response). The acute ACTH response in Avpr1b KO mice, while reduced from that seen in wild-type animals, is the same for repeated SS. Furthermore, the ACTH response to repeated SS in Avpr1b KO mice is not different from that seen in wild-type mice. It is tempting to speculate that there is a loss of adaptation to repeated SS in Avpr1b KOs but such a conclusion would be tenuous since ACTH levels in acutely stressed Avpr1b KO mice are already very low. The lack of functional Avpr1b in the KO mice has such a profound effect on the ACTH response to SS in these mice that any non-Avpr1b-mediated adaptation to repeated SS is probably negligible. The studies in Avpr1b KO mice and Brattleboro rats highlight the discrepancy between Avp/Avpr1b-mediated ACTH and CORT secretion during acute and repeated stress—this may have implications on the potential use of Avpr1b antagonists to ameliorate symptoms of HPA axis hyperactivity in stress-related disorders.
Figure 2. Plasma hormone levels of male wild type (+/+) and KO ( − / − ) mice following acute and chronic (repeated) SS. Note that the ACTH response to acute SS in wild type but not Avpr1b KO animals is significantly reduced following repeated SS. Mice of appropriate genotype generated from parental crosses heterozygous for the Avpr1b were randomly assigned to an experimental group containing 7–8 mice. SS consisted of shaking mice in separate clean empty cages on a standard bench top orbital shaker (stroke: 38 mm at 150 rpm) for 10 min. Mice were returned to their home cage for a further 10 min prior to sacrifice. Chronically shaken mice were stressed once daily for 10 days whereas mice in acute groups were handled for 9 days and stressed once on the 10th day. Control mice were handled once daily and all experiments were performed between 0900 and 1130 h in accordance with UK Home Office regulations (Animal Scientific Procedures Act (1986)) and approved by the University of Bristol Ethical Review Board. Plasma ACTH (graph A) and CORT levels (graph B) were determined by ELISA and EIA, respectively (IDS, Tyne and Wear, UK). Data are expressed as mean ± SEM. Significant differences are denoted as ***: P < 0.001, NS: not significant.
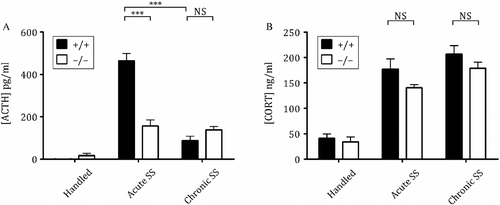
Gene expression in Avpr1b KO mice
Whether the deficits observed in Avpr1b KO mice outlined above are a direct result of disruption of Avpr1b signalling pathways or the result of an altered compensatory expression profile is not known. As far as HPA axis function is concerned, clearly Crh (or Oxt) does not compensate for the loss of the Avpr1b in Avpr1b KO mice. There are some direct changes that occur in KO mice that give rise to phenotypes such as altered glucose metabolism and attenuated ACTH release; however, indirect changes that affect behavioural systems that may lead to the deficits seen in Avpr1b KOs have yet to be identified. We have used ISHH to assess basal gene transcript levels of a number of genes that are closely linked with HPA axis function and find no significant differences in gene expression between Avpr1b KO and wild-type mice (). Comparisons of Oxtr mRNA levels in Avpr1b KO and wild-type mouse anterior pituitaries appear to suggest an upregulation of Oxtrs in Avpr1b KO mice (Nakamura et al. Citation2008). As Oxt at high concentrations can elicit ACTH release via the Avpr1b, and the Oxtr may be expressed in corticotrophs, it has been suggested that increased expression of Oxtrs may be a compensatory mechanism through which Avpr1b KO mice and Brattleboro rats can, to some degree, make up for the lack of Avpr1b/Avp-mediated ACTH release (Nakamura et al. Citation2008). We cannot totally rule out the possibility that mechanisms compensating for the loss of Avpr1b are active in the Avpr1b KO. Changes in neurochemical networks active centrally (e.g. projections to the PVN or signals within the PVN itself) or at the level of the anterior pituitary and adrenal may have occurred. We also cannot exclude a role of central Avpr1bs (or for that matter Avpr1as) in directly or indirectly influencing HPA axis activity. Nevertheless, stress-induced ACTH levels in our Avpr1b KOs are consistently lower (often to basal levels) than wild-type controls, confirming any compensation (e.g. from the action of Crh) is not sufficient to fully counteract the loss of the Avpr1b (Lolait et al. Citation2007a,Citationb; Stewart et al. Citation2008a,Citationb). The role of potential Oxtr-mediated ACTH release in Avpr1b KO mice and Brattleboro rats remains to be clarified, however, as the Oxtr is predominantly located in lactotrophs rather than corticotrophs in the anterior pituitary (Breton et al. Citation1995) it is likely that the Oxtr plays a minor role compared to the Avpr1b in wild-type animals. The use of conditional KOs and emerging pharmacological developments will no doubt help clarify the contribution of the pituitary Oxtr in acute and chronic stress.
Figure 3. Representative ISHH photomicrographs of gene expression in brain and pituitary of male Avpr1b KO and wild-type mice. Riboprobes corresponding to the following sequences were used: 5-HT1a receptor (Htr1a) (bp1230–1597 of Genbank Accession number NM_008308), 5-HT2a receptor (Htr2a) (bp1884–2492 of Acc#NM_172812), 5-HT2c receptor (Htr2c) (bp1435–1965 of Acc#NM_008312), brain-derived neurotrophic factor (Bdnf) (bp310–822 of Acc#AK017559), cannabinoid CB1R (Cnr1) (bp728–1040 of Acc#Y18374), Crh (bp101–686 of Acc#205769), type 1 Crh receptor (Crhr1) (bp160–528 of Acc#NM_007762), glucocorticoid receptor (Nr3c1) (bp439–958 of Acc#X04435), Oxt (bp1753–1865 of Acc# M88355), Avp (bp2966–3388 of Acc# M88354), intronic Avp (bp1965–2406 of Acc#M88354) and pro-opiomelanocortin (Pomc) (bp145-620 of Acc#V01529). The intronic Avp probe was targeted towards intron 1 of the Avp/neurophysin II gene to reflect heteronuclear RNA expression levels. These probes were obtained by PCR using genomic DNA, or brain (Crhr1, Crh, Bdnf, Avp and Oxt) or pituitary (Pomc) cDNA from 129 Sv mouse tissue as template. Restriction sites were incorporated into the 5′ ends of PCR primers to facilitate cloning of the PCR product into vector pGEM4Z. The integrity of all riboprobes was verified by DNA sequencing. ISHH on 12 μm sections with 35S-labelled riboprobes was performed as in ref. (Lolait et al. Citation2007a). Sections were exposed to X-ray film (Hyperfilm MP, Amersham; GE Healthcare, Little Chalfont, UK) for hours (Pomc, Avp & Oxt) to weeks. Sections hybridised with the corresponding sense probes gave negligible, background staining (not shown). Brain regions shown include the nucleus accumbens (NA), PVN, supraoptic nucleus (SON) and the HIP. Sections of brain hybridised with the Nr3c1 probe were cut at a greater pitch around a mediolateral axis to obtain both HIP and PVN in one slice. Photomicrographs were taken at 6.3 × magnification and resized for publication (except Oxt, Avp and intronic Avp probes which are magnified appropriately to show the PVN or PVN/SON in greater detail).
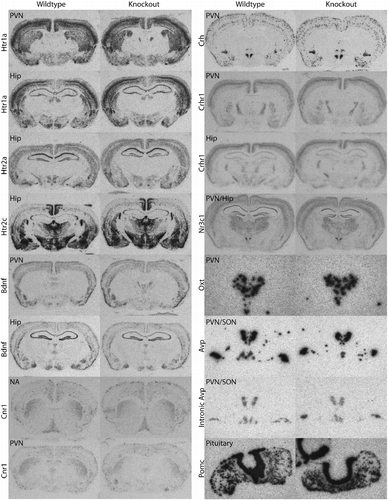
Emerging pharmacological data
A number of agonists (such as desmopressin, an Avpr2 agonist) and antagonists (such as relcovaptan, an Avpr1a antagonist) are available and frequently used to study the Avpr1a, Avpr2 and Oxtr (for review see Lemmens-Gruber and Kamyar Citation2006; Manning et al. Citation2008). Some of these are in clinical trials or have been approved for use as treatments in disorders such as nocturnal enuresis and neurogenic diabetes insipidus. The search for Avpr1b pharmacological tools has gained much impetus due to their potential as treatments for conditions associated with chronic stress such as anxiety and depression (for short review see Griebel et al. Citation2005; Arban Citation2007). The most widely used non-peptide Avpr1b antagonist, SSR149415, has been used extensively in research since its characterisation in 2002 (Serradeil-Le Gal et al. Citation2002) (see, ). SSR149415 is orally active and inhibits some but not all acute stressor-induced ACTH release in rats (Serradeil-Le Gal et al. Citation2002; Ramos et al. Citation2006), and does not affect HPA hypersensitivity to novel stressors (Chen et al. Citation2008). The compound acts at the human Avpr1b and also has some antagonist activity at the human Oxtr in vitro (Griffante et al. Citation2005) but has high selectivity and nanomolar affinity for rodent forms of the Avpr1b (Serradeil-Le Gal et al. Citation2002). In mice and rats, SSR149415 has been tested in a variety of classical models of anxiety (e.g. elevated plus maze, light/dark box test) and depression (e.g. forced swim test, chronic mild stress) as well as other models (e.g. olfactory bulbectomy, Flinder's sensitive line) that are used to determine the efficacy of potential antidepressant and anxiolytic drugs (Griebel et al. Citation2002; Overstreet and Griebel Citation2005; Stemmelin et al. Citation2005; Louis et al. Citation2006; Salomé et al. Citation2006; Shimazaki et al. Citation2006; Hodgson et al. Citation2007; Iijima and Chaki Citation2007). Peripheral and central pretreatment with SSR149415 reduces anxiety and depressive-related behaviour in these tests with high compatibility between the findings of these studies. SSR149415 also reduces aggression in hamsters (CitationBlanchard et al. 2005), significantly reverses the reduction in dentate gyrus cell proliferation caused by chronic mild stress in mice (Alonso et al. Citation2004) and blocks stress-induced hyperalgesia in rats (Bradesi et al. Citation2009). It has also been radiolabelled with tritium and used in receptor autoradiography to reveal low-resolution binding in the human and rat pituitary—no Avpr1b binding sites were observed in sections of rat brain (Serradeil-Le Gal et al. Citation2007). Recently, SSR149415 has failed phase II clinical trials (Kirchhoff et al. Citation2009). Overall, the results of studies with SSR149415 evidence a possible role for the Avpr1b in affective disorders and point to animal model-validated targets with which to treat them.
Table III. Summary of studies conducted with Avpr1b antagonists on HPA activity in vivo.
Table IV. Summary of studies conducted with Avpr1b antagonists on behaviour.
Around the same time, SSR149415 was first reported as an Avpr1b antagonist, the Avp peptido mimetic [1-deamino-4-cyclohexylalanine] arginine vasopressin (d[Cha4]Avp) was described (Derick et al. Citation2002). This agonist was the first to show efficacy at nanomolar concentrations and stimulate the release of ACTH/CORT without exhibiting vascular or renal activity (Derick et al. Citation2002). Other peptide agonists selective for the rat Avpr1b have been generated by modifying positions 4 and 8 of the Avp analogue deamino-[Cys]arginine vasopressin (Pena et al. Citation2007a). Many of these modified Avp analogues display high selectivity for the Avpr1b and bind with sub-nanomolar affinities (Pena et al. Citation2007a) and thus could well be useful in the study of the Avp receptors in rodents; however, they may be of limited use as human therapeutics due to their peptidergic nature. The agonists created since d[Cha4]Avp do, however, have an increasingly refined agonist profile. One such member of the recent modified range of Avp analogue agonists, d[Leu4,Lys8]Avp, is noted to be a full agonist at human, rat and mouse Avpr1bs in vitro as well as stimulates ACTH and insulin release at low doses from mouse pituitary and perfused rat pancreas, respectively (Pena et al. Citation2007b). This effect of d[Leu4,Lys8]Avp on mouse and rat tissue is blocked when co-administered with SSR149415 (Pena et al. Citation2007b).
Since the development of SSR149415, there have been some recent reports of a non-peptide antagonist (Org) that is highly selective for the human and rat Avpr1b (Craighead et al. Citation2008). Pretreatment of rats with this compound causes a significant reduction in ACTH release after restraint stress or lipopolysaccharide (LPS) challenge (Spiga et al. Citation2009a) and to a heterotypic stressor after repeated restraint (Spiga et al. Citation2009b) (see ). However, Org does not affect repeated restraint stress-induced ACTH/CORT adaptation in rats (Spiga et al. Citation2009a). Another set of Avpr1b antagonists (ABT-436 and ABT-558) have subnanomolar affinity for the human Avpr1b with approximately 30-fold lower affinity for rat and mouse Avpr1bs (Wernet et al. Citation2008). These compounds attenuate acute restraint stress-induced ACTH release (Behl et al. Citation2008) and appear to have increased anxiolytic-like and antidepressant-like potency and efficacy compared to SSR149415 (van Gaalen et al. Citation2008). More recently, other non-peptide antagonists have been described: “p”, a tetrahydroquinoline sulphonamide derivative, with high selectivity for the rat and human Avpr1b (Kis approximately 21 nM and 44 nM, respectively) (Scott et al. Citation2009), and compounds generated from a series of pyrrole-pyrazinone and pyrazole-pyrazinone derivatives which also appear to show good selectivity and high potency (e.g., compound 11 pIC50 = 8.4) for the human Avpr1b expressed in Chinese hamster ovary cells (Arban et al. Citation2010)—to our knowledge the effects of these compounds on HPA axis activity or behaviour have not been reported to date.
In conclusion, the distribution and functional studies of the Avpr1b have established its major role in the pituitary where it plays a pivotal part in the regulation of the HPA response to stress, and in particular to acute stress. Additionally, we see several, perhaps minor, metabolic and endocrine roles in the periphery. Behavioural changes generated from experiments in Avpr1b KO animals, together with recent Avpr1b antagonist data, have highlighted a more elusive central role for this receptor. The behavioural implications of Avp, acting via the Avpr1b, in aggression and stress, and the integral connection between stress, anxiety and depression make the Avpr1b an attractive target for pharmacological intervention. Increased Avp secretion and enhanced pituitary responsiveness to Avp have been reported in some subtypes of depression (e.g. melancholic depression)(see, Dinan and Scott Citation2005 for a review). Furthermore, polymorphisms in the Avpr1b gene have been associated with depression (van West et al. Citation2004), childhood-onset mood disorder (Dempster et al. Citation2007) and attention-deficit hyperactivity disorder (van West et al. Citation2009). The progress made in generating compounds selective for this receptor may have considerable implications for potential treatments for a number of disease states as well as for Avp research in general. The development of new compounds that can be radiolabelled to high specific activity is a critical step in Avpr1b research as determination of its central distribution may well provide an anatomical template to assign how changes in behaviours or disease states are influenced. Further developments in molecular (e.g. conditional Avpr1b KOs; the use of Avpr1b-specific small-interfering RNAs to selectively silence Avpr1b gene expression in specific brain regions) and pharmacological tools for use in rodents and primates (e.g. positron emission topography (PET) ligands, see Schonberger et al. Citation2010) will help elucidate the full function of the Avpr1b and thus the therapeutic value research into this receptor may hold.
Acknowledgements
J.A.R received funding from the Neuroendocrinology Charitable Trust (UK) and the Schering-Plough Corporation; A.M.O'C received funding from the Wellcome Trust and BBSRC. This research was supported by the NIMH Intramural Research Program (Z01-MH-002498-21). S.J.L received funding from the Wellcome Trust.
Declaration of interest: J.A.R and S.J.L have used the Avpr1b antagonist Org (supplied by the Schering-Plough Corporation) in unpublished studies in mice. The authors alone are responsible for the content and writing of the paper.
Notice of correction
Please note that the following amendments have been made to this paper, first published Online Early 9 September 2010: the removal of a duplication of text within Table I caption on page 6; in Table IV on page 12, the heading ‘Anxiety related behaviour’ has been moved up to the 2nd line in column one from its previous position at line 12; and a spelling error, incorrect year of publication and incorrect volume details have been amended in the Zhou et al. 2008 reference list and citations in Tables III and IV.
References
- Abou-Samra AB, Catt KJ, Aguilera G. 1986. Involvement of protein kinase C in the regulation of adrenocorticotropin release from rat anterior pituitary cells. Endocrinology. 118:212–217.
- Abu-Basha EA, Yibchok-anun S, Hsu WH. 2002. Glucose dependency of arginine vasopressin-induced insulin and glucagon release from the perfused rat pancreas. Metab Clin Exp. 51:1184–1190.
- Aguilera G. 1994. Regulation of pituitary ACTH secretion during chronic stress. Front Neuroendocrinol. 15:321–350.
- Aguilera G, Rabadan-Diehl C. 2000. Vasopressinergic regulation of the hypothalamic–pituitary–adrenal axis: Implications for stress adaptation. Regul Pept. 96:23–29.
- Aguilera G, Subburaju S, Young S, Chen J. 2008. The parvocellular vasopressinergic system and responsiveness of the hypothalamic pituitary adrenal axis during chronic stress. Prog Brain Res. 170:29–39.
- Alexander SL, Roud HK, Irvine CH. 1997. Effect of insulin-induced hypoglycaemia on secretion patterns and rates of corticotrophin-releasing hormone, arginine vasopressin and adrenocorticotrophin in horses. J Endocrinol. 153:401–409.
- Alonso R, Griebel G, Pavone G, Stemmelin J, Le Fur G, Soubrie P. 2004. Blockade of CRF1 or V1b receptors reverses stress-induced suppression of neurogenesis in a mouse model of depression. Mol Psychiatry. 9:278–286.
- Armario A, Vallès A, Dal-Zotto S, Màrquez C, Belda X. 2004. A single exposure to severe stressors causes long-term desensitisation of the physiological responses to the homotypic stressor. Stress. 7:157–172.
- Anisman H, Hayley S, Kelly O, Borowski T, Merali Z. 2001. Psychogenic, neurogenic, and systemic stressor effects on plasma corticosterone and behavior: Mouse strain-dependent outcomes. Behav Neurosci. 115:443–454.
- Antoni FA. 1993. Vasopressinergic control of pituitary adrenocorticotropin secretion comes of age. Front Neuroendocrinol. 14:76–122.
- Arban R. 2007. V1b receptors: New probes for therapy. Endocrinology. 148:4133–4135.
- Arban R, Bianchi F, Buson A, Cremonesi S, Di Fabio R, Gentile G, Micheli F, Pasquarello A, Pozzan A, Tarsi L, Terreni S, Tonelli F. 2010. Pyrrolo[1,2-α]pyrazine and pyrazolo[1,5-α]pyrazine: Novel, potent, and selective series of vasopressin1b receptor antagonists. Bioorg Med Chem Lett. 20:5044–5049.
- Barberis C, Tribollet E. 1996. Vasopressin and oxytocin receptors in the central nervous system. Crit Rev Neurobiol. 10:119–154.
- Beery AK, Lacey EA, Francis DD. 2008. Oxytocin and vasopressin receptor distributions in a solitary and a social species of tuco-tuco (Ctenomys haigi and Ctenomys sociabilis). J Comp Neurol. 507:1847–1859.
- Behl B, Dreschner KU, van Gaalen MM, Gross G, Netz A, Oost T, Schoemaker H, Sullivan JP, Wernet W. 2008. Inhibition of HPA axis activation by the V1b receptor antagonists ABT-436 and ABT-558. In: Proceedings of Society for Neuroscience, Washington DC, USA. Abstract#560.17/FF5.
- Blanchard RJ, Griebel G, Farrokhi C, Markham C, Yang M, Blanchard DC. AVP V1b selective antagonist SSR149415 blocks aggressive behaviors in hamsters. Pharmacol Biochem Behav. 80:189–194.
- Bornstein SR, Engeland WC, Ehrhart-Bornstein M, Herman JP. 2008. Dissociation of ACTH and glucocorticoids. Trends Endocrinol Metab. 19:175–180.
- Bradesi S, Martinez V, Lao L, Larsson H, Mayer EA. 2009. Involvement of vasopressin 3 receptors in chronic psychological sress-induced visceral hyperalgesia in rats. Am J Physiol Gastrointest Liver Physiol. 296:G302–G309.
- Breton C, Pechoux C, Morel G, Zingg HH. 1995. Oxytocin receptor messenger ribonucleic acid: Characterization, regulation, and cellular localization in the rat pituitary gland. Endocrinology. 136:2928–2936.
- Burbach PJ, Adan RA, Lolait SJ, van Leeuwen FW, Mezey E, Palkovits M, Barberis C. 1995. Molecular neurobiology and pharmacology of the vasopressin/oxytocin receptor family. Cell Mol Neurobiol. 15:573–595.
- Caldwell HK, Albers HE. 2004. Effect of photoperiod on vasopressin-induced aggression in Syrian hamsters. Horm Behav. 46:444–449.
- Caldwell HK, Young WS. 2009. Persistence of reduced aggression in vasopressin 1b receptor knockout mice on a more “wild” background. Physiol Behav. 97:131–134.
- Caldwell HK, Stewart J, Wiedholz LM, Millstein RA, Iacangelo A, Holmes A, Young WS, Wersinger SR. 2006. The acute intoxicating effects of ethanol are not dependent on the vasopressin 1a or 1b receptors. Neuropeptides. 40:325–337.
- Caldwell HK, Lee H-J, Macbeth AH, Young WS. Vasopressin: Behavioral roles of an “original” neuropeptide. Prog Neurobiol. 2008a; 84:1–24.
- Caldwell HK, Wersinger SR, Young WS. The role of the vasopressin 1b receptor in aggression and other social behaviours. Prog Brain Res. 2008b; 170:65–72.
- Caldwell HK, Dike OE, Stevenson EL, Storck K, Young WS. 2010. Social dominence in male vasopressin 1b receptor knockout mice. Horm Behav. 58:257–263.
- Carrasco GA, Van de Kar LD. 2003. Neuroendocrine pharmacology of stress. Eur J Pharmacol. 463:235–272.
- Chen J, Young S, Subburaju S, Sheppard J, Kiss A, Atkinson HC, Wood S, Lightman S, Serradeil-Le Gal C, Aguilera G. 2008. Vasopressin does not mediate hypersensitivity of the hypothalamic pituitary adrenal axis during chronic stress. Ann N Y Acad Sci. 1148:349–359.
- Chini B, Manning M. 2007. Agonist selectivity in the oxytocin/vasopressin receptor family: New insights and challenges. Biochem Soc Trans. 35:737–741.
- Claustre Y, Rouquier L, Desvignes C, Leonetti M, Montegut J, Aubin N, Allouard N, Bougault L, Oury-Donat F, Steinberg R. 2006. Effects of the vasopressin (V1B) areceptor antagonist, SSR149415, and the corticotropin-releasing factor 1 receptor antagonist, SSR125543, on FG 7142-induced increase in acetylcholine and norepinephrine release in the rat. Neuroscience. 141:1481–1488.
- Craighead M, Milne R, Campbell-Wan L, Watson L, Presland J, Thomson FJ, Marston HM, Macsweeney CP. 2008. Characterization of a novel and selective V1B receptor antagonist. Prog Brain Res. 170:527–535.
- Dallman MF, Viau VG, Bhatnagar S, Gomez F, Laugero K, Bell ME. 2002. Corticotrophin-releasing factor, corticosteroids, stress, and sugar: Energy balance, the brain, and behaviour. In: Pfaff DW, Arnold AP, Etgen AM, Fahrbach SE, Rubin RT. editors. Hormones, brain and behaviour. New York: Academic Press. p 571–631.
- DeVito LM, Konigsberg R, Lykken C, Sauvage M, Young WS, Eichenbaum H. 2009. Vasopressin 1b receptor knock-out impairs memory for temporal order. J Neurosci. 29:2676–2683.
- Dempster EL, Burcescu I, Wigg K, Kiss E, Baji I, Gadoros J, Tamas Z, Kennedy JL, Vetro A, Kovacs M, Barr CL. 2007. Evidence for an association between the vasopressin V1b receptor gene (Avpr1b) and childhood-onset mood disorders. Arch Gen. 64:1189–1195.
- Derick S, Cheng LL, Voirol MJ, Stoev S, Giacomini M, Wo NC, Szeto HH, Ben Mimoun M, Andres M, Gaillard RC, Guillon G, Manning M. 2002. [1-deamino-4-cyclohexylalanine] arginine vasopressin: A potent and specific agonist for vasopressin V1b receptors. Endocrinology. 143:4655–4664.
- Dinan TG, Scott LV. 2005. Anatomy of melancholia: Focus on hypothalamic–pituitary–adrenal axis overactivity and the role of vasopressin. J Anat. 207:259–264.
- Domokos A, Mergl Z, Barna I, Makara GB, Zelena D. 2008. Congenital vasopressin deficiency and acute and chronic opiate effects on hypothalamo–pituitary–adrenal axis activity in Brattleboro rats. J Endocrinol. 196:113–121.
- Egashira N, Tanoue A, Higashihara F, Fuchigami H, Sano K, Mishima K, Fukue Y, Nagai H, Takano Y, Tsujimoto G, Stemmelin J, Griebel G, Iwasaki K, Ikeda T, Nishimura R, Fujiwara M. 2005. Disruption of the prepulse inhibition of the startle reflex in vasopressin V1b receptor knockout mice: Reversal by antipsychotic drugs. Neuropsychopharmacology. 30:1996–2005.
- Ehrhart-Bornstein M, Hinson JP, Bornstein SR, Scherbaum WA, Vinson GP. 1998. Intraadrenal interactions in the regulation of adrenocortical steroidogenesis. Endocr Rev. 19:101–143.
- Engin E, Treit D. 2008. Dissociation of the anxiolytic-like effects of Avpr1a and Avpr1b receptor antagonists in the dorsal and ventral hippocampus. Neuropeptides. 42:411–421.
- Engler D, Pham T, Fullerton MJ, Ooi G, Funder JW, Clarke IJ. 1989. Studies of the secretion of corticotropin-releasing factor and arginine vasopressin into the hypophysial-portal circulation of the conscious sheep. I. Effect of an audiovisual stimulus and insulin-induced hypoglycemia. Neuroendocrinology. 49:367–381.
- Ferris CF, Melloni RH, Koppel G, Perry KW, Fuller RW, Delville Y. 1997. Vasopressin/serotonin interactions in the anterior hypothalamus control aggressive behavior in golden hamsters. J Neurosci. 17:4331–4340.
- Ferris CF, Lu S-F, Messenger T, Guillon CD, Heindel N, Miller M, Koppel G, Robert Bruns F, Simon NG. 2006. Orally active vasopressin V1a receptor antagonist, SRX251, selectively blocks aggressive behavior. Pharmacol Biochem Behav. 83:169–174.
- Folny V, Raufaste D, Lukovic L, Pouzet B, Rochard P, Pascal M, Serradeil-Le Gal C. 2003. Pancreatic vasopressin V1b receptors: Characterization in In-R1-G9 cells and localization in human pancreas. Am J Physiol Endocrinol Metab. 285:E566–E576.
- Fujiwara Y, Hiroyama M, Sanbe A, Aoyagi T, Birumachi J-I, Yamauchi J, Tsujimoto G, Tanoue A. Insulin hypersensitivity in mice lacking the V1b vasopressin receptor. J Physiol. 2007a; 584:235–244.
- Fujiwara Y, Hiroyama M, Sanbe A, Yamauchi J, Tsujimoto G, Tanoue A. Mutual regulation of vasopressin- and oxytocin-induced glucagon secretion in V1b vasopressin receptor knockout mice. J Endocrinol. 2007b; 192:361–369.
- van Gaalen MM, Basso AM, Bespalov AY, Rueter LE, Oost T, Breuer M, Olivier B, Beyerbach A, Gross G, Sullivan JP, Schoemaker H, Wernet W. 2008. Antidepressant- and anxiolytic-like effects of the vasopressin V1b receptor antagonists ABT-436 and ABT-558. In: Proceedings of Society for Neuroscience, Washington DC, USA. Abstract#560.18/FF6.
- Gallo-Payet N, Guillon G. Regulation of adrenocortical function by vasopressin. Horm Metab Res. 30:360–367.
- Gillies GE, Linton EA, Lowry PJ. 1982. Corticotropin releasing activity of the new CRF is potentiated several times by vasopressin. Nature. 299:355–357.
- Grazzini E, Lodboerer AM, Perez-Martin A, Joubert D, Guillon G. 1996. Molecular and functional characterization of V1b vasopressin receptor in rat adrenal medulla. Endocrinology. 137:3906–3914.
- Grazzini E, Boccara G, Joubert D, Trueba M, Durroux T, Guillon G, Gallo-Payet N, Chouinard L, Payet MD, Serradeil Le Gal C. 1998. Vasopressin regulates adrenal functions by acting through different vasopressin receptor subtypes. Adv Exp Med Biol. 449:325–334.
- Grazzini E, Breton C, Derick S, Andres M, Raufaste D, Rickwaert F, Boccara G, Colson P, Guérineau NC, Serradeil-Le Gal C, Guillon G. 1999. Vasopressin receptors in human adrenal medulla and pheochromocytoma. J Clin Endocrinol Metab. 84:2195–2203.
- Griebel G, Simiand J, Serradeil-Le Gal C, Wagnon J, Pascal M, Scatton B, Maffrand J-P, Soubrie P. 2002. Anxiolytic- and antidepressant-like effects of the non-peptide vasopressin V1b receptor antagonist, SSR149415, suggest an innovative approach for the treatment of stress-related disorders. Proc Natl Acad Sci USA. 99:6370–6375.
- Griebel G, Stemmelin J, Gal CS-L, Soubrié P. 2005. Non-peptide vasopressin V1b receptor antagonists as potential drugs for the treatment of stress-related disorders. Curr Pharm Des. 11:1549–1559.
- Griffante C, Green A, Curcuruto O, Haslam CP, Dickinson BA, Arban R. 2005. Selectivity of d[Cha4]AVP and SSR149415 at human vasopressin and oxytocin receptors: Evidence that SSR149415 is a mixed vasopressin V1b/oxytocin receptor antagonist. Br J Pharmacol. 146:744–751.
- Guillon G, Trueba M, Joubert D, Grazzini E, Chouinard L, Côté M, Payet MD, Manzoni O, Barberis C, Robert M. 1995. Vasopressin stimulates steroid secretion in human adrenal glands: Comparison with angiotensin-II effect. Endocrinology. 136:1285–1295.
- Hammock EAD, Young LJ. 2002. Variation in the vasopressin V1a receptor promoter and expression: Implications for inter- and intraspecific variation in social behaviour. Eur J Neurosci. 16:399–402.
- Hammock EAD, Young LJ. 2005. Microsatellite instability generates diversity in brain and sociobehavioral traits. Science. 308:1630–1634.
- Harbuz MS, Lightman SL. 1992. Stress and the hypothalamo–pituitary–adrenal axis: Acute, chronic and immunological activation. J Endocrinol. 134:327–339.
- Herman JP, Figueiredo H, Mueller NK, Ulrich-Lai Y, Ostrander MM, Choi DC, Cullinan WE. 2003. Central mechanisms of stress integration: Hierarchical circuitry controlling hypothalamo–pituitary–adrenocortical responsiveness. Front Neuroendocrinol. 24:151–180.
- Hernando F, Schoots O, Lolait SJ, Burbach JP. 2001. Immunohistochemical localization of the vasopressin V1b receptor in the rat brain and pituitary gland: Anatomical support for its involvement in the central effects of vasopressin. Endocrinology. 142:1659–1668.
- Hodgson RA, Higgins GA, Guthrie DH, Lu SX, Pond AJ, Mullins DE, Guzzi MF, Parker EM, Varty GB. 2007. Comparison of the V1b antagonist, SSR149415, and the CRF1 antagonist, CP-154,526, in rodent models of anxiety and depression. Pharmacol Biochem Behav. 86:431–440.
- Iijima M, Chaki S. 2007. An arginine vasopressin V1b antagonist, SSR149415 elicits antidepressant-like effects in an olfactory bulbectomy model. Prog Neuropsychopharmacol Biol Psychiatry. 31:622–627.
- Ishizuka Y, Abe H, Tanoue A, Kannan H, Ishida Y. 2010. Involvement of vasopressin V1b receptor in anti-anxiety action of SSRI and SNRI in mice. Neurosci Res. 66:233–237.
- Itoh S, Yamada S, Mori T, Miwa T, Tottori K, Uwahodo Y, Yamamura Y, Fukuda M, Yamamoto K, Tanoue A, Tsujimoto G. 2006. Attenuated stress-induced catecholamine release in mice lacking the vasopressin V1b receptor. Am J Physiol Endocrinol Metab. 291:E147–E151.
- Jard S, Gaillard RC, Guillon G, Marie J, Schoenenberg P, Muller AF, Manning M, Sawyer WH. 1986. Vasopressin antagonists allow demonstration of a novel type of vasopressin receptor in the rat adenohypophysis. Mol Pharmacol. 30:171–177.
- Jard S, Barberis C, Audigier S, Tribollet E. 1987. Neurohypophyseal hormone receptor systems in brain and periphery. Prog Brain Res. 72:173–187.
- Jeng YJ, Lolait SJ, Strakova Z, Chen C, Copland JA, Mellman D, Hellmich MR, Soloff MS. 1996. Molecular cloning and functional characterization of the oxytocin receptor from a rat pancreatic cell line (RINm5F). Neuropeptides. 30:557–565.
- Kalueff AV, Wheaton M, Murphy DL. 2007. What's wrong with my mouse model? Advances and strategies in animal modeling of anxiety and depression. Behav Brain Res. 179:1–18.
- Kirchhoff VD, Nguyen HTT, Soczynska JK, Woldeyohannes HO, McIntyre RS. 2009. Discontinued psychiatric drugs in 2008. Expert Opin Investig Drugs. 18:1431–1443.
- Kirk CJ, Rodrigues LM, Hems DA. 1979. The influence of vasopressin and related peptides on glycogen phosphorylase activity and phosphatidylinositol metabolism in hepatocytes. Biochem J. 178:493–496.
- Knepper MA. 1997. Molecular physiology of urinary concentrating mechanism: Regulation of aquaporin water channels by vasopressin. Am J Physiol. 272:F3–F12.
- Koshimizu T-A, Nasa Y, Tanoue A, Oikawa R, Kawahara Y, Kiyono Y, Adachi T, Tanaka T, Kuwaki T, Mori T, Takeo S, Okamura H, Tsujimoto G. 2006. V1a vasopressin receptors maintain normal blood pressure by regulating circulating blood volume and baroreflex sensitivity. Proc Natl Acad Sci USA. 103:7807–7812.
- Lemmens-Gruber R, Kamyar M. 2006. Vasopressin antagonists. Cell Mol Life Sci. 63:1766–1779.
- Lolait SJ, O'Carroll A-M, Mahan LC, Felder CC, Button DC, Young WS, Mezey E, Brownstein MJ. 1995. Extrapituitary expression of the rat V1b vasopressin receptor gene. Proc Natl Acad Sci USA. 92:6783–6787.
- Lolait SJ, Stewart LQ, Jessop DS, Young WS, O'Carroll A-M. The hypothalamic–pituitary–adrenal axis response to stress in mice lacking functional vasopressin V1b receptors. Endocrinology. 2007a; 148:849–856.
- Lolait SJ, Stewart LQ, Roper JA, Harrison G, Jessop DS, Young WS, O'Carroll A-M. Attenuated stress response to acute lipopolysaccharide challenge and ethanol administration in vasopressin V1b receptor knockout mice. J Neuroendocrinol. 2007b; 19:543–551.
- Louis C, Cohen C, Depoortere R, Griebel G. 2006. Antidepressant-like effects of the corticotropin-releasing factor I receptor antagonist, SSR125543, and the vasopressin Ib receptor antagonist, SSR149415, in a DRL-72s schedule in the rat. Neuropsychopharmacology. 31:2180–2187.
- Manning M, Stoev S, Chini B, Durroux T, Mouillac B, Guillon G. 2008. Peptide and non-peptide agonists and antagonists for the vasopressin and oxytocin V1a, V1b, V2 and OT receptors: Research tools and potential therapeutic agents. Prog Brain Res. 170:473–512.
- Marti O, Armario A. 1998. Anterior pituitary response to stress: Time-related changes and adaptation. Int J Dev Neurosci. 16:241–260.
- Ma X-M, Levy A, Lightman SL. 1997. Emergence of an isolated arginine vasopressin (AVP) response to stress after repeated restraint: A study of both AVP and corticotropin-releasing hormone messenger ribonucleic acid (RNA) and heteronuclear RNA. Endocrinology. 138:4351–4357.
- Ma X-M, Lightman SL, Aguilera G. 1999. Vasopressin and corticotropin-releasing hormone gene responses to novel stress in rats adapted to repeated restraint. Endocrinology. 140:3623–3632.
- Mlynarik M, Zelena D, Bagdy G, Makara GB, Jezova D. 2007. Signs of attenuated depression-like behaviour in vasopressin deficient Brattleboro rats. Horm Behav. 51:395–405.
- Morel A, O'Carroll A-M, Brownstein MJ, Lolait SJ. 1992. Molecular cloning and expression of a rat V1a arginine vasopressin receptor. Nature. 356:523–526.
- Nakamura K, Fujiwara Y, Mizutani R, Sanbe A, Miyauchi N, Hiroyama M, Yamauchi J, Yamashita T, Nakamura S, Mori T, Tsujimoto G, Tanoue A. 2008. Effects of vasopressin V1b receptor deficiency on adrenocorticotropin release from anterior pituitary cells in response to oxytocin stimulation. Endocrinology. 149:4883–4891.
- O'Carroll A-M, Howell GM, Roberts EM, Lolait SJ. 2008. Vasopressin potentiates corticotropin-releasing hormone-induced insulin release from mouse pancreatic beta-cells. J Endocrinol. 197:231–239.
- Oshikawa S, Tanoue A, Koshimizu T-A, Kitagawa Y, Tsujimoto G. 2004. Vasopressin stimulates insulin release from islet cells through V1b receptors: A combined pharmacological/knockout approach. Mol Pharmacol. 65:623–629.
- Ostrowski NL, Lolait SJ, Young WS. 1994. Cellular localization of vasopressin V1a receptor messenger ribonucleic acid in adult male rat brain, pineal, and brain vasculature. Endocrinology. 135:1511–1528.
- Overstreet DH, Griebel G. 2005. Antidepressant-like effects of the vasopressin V1b receptor antagonist SSR149415 in the Flinders Sensitive Line rat. Pharmacol Biochem Behav. 82:223–227.
- Pena A, Murat B, Trueba M, Ventura MA, Wo NC, Szeto HH, Cheng LL, Stoev S, Guillon G, Manning M. Design and synthesis of the first selective agonists for the rat vasopressin V(1b) receptor: Based on modifications of deamino-[Cys1]arginine vasopressin at positions 4 and 8. J Med Chem. 2007a; 50:835–847.
- Pena A, Murat B, Trueba M, Ventura MA, Bertrand G, Cheng LL, Stoev S, Szeto HH, Wo N, Brossard G, Serradeil-Le Gal C, Manning M, Guillon G. Pharmacological and physiological characterization of d[Leu4, Lys8]vasopressin, the first V1b-selective agonist for rat vasopressin/oxytocin receptors. Endocrinology. 2007b; 148:4136–4146.
- Plotsky PM, Bruhn TO, Vale W. 1985. Hypophysiotropic regulation of adrenocorticotropin secretion in response to insulin-induced hypoglycemia. Endocrinology. 117:323–329.
- Ramos AT, Troncone LRP, Tufik S. 2006. Suppression of adrenocorticotrophic hormone secretion by simultaneous antagonism of vasopressin 1b and CRH-1 receptors on three different stress models. Neuroendocrinology. 84:309–316.
- Reversi A, Cassoni P, Chini B. 2005. Oxytocin receptor signaling in myoepithelial and cancer cells. J Mammary Gland Biol Neoplasia. 10:221–229.
- Rivier C, Vale W. 1983. Interaction of corticotropin-releasing factor and arginine vasopressin on adrenocorticotropin secretion in vivo. Endocrinology. 113:939–942.
- Saito M, Sugimoto T, Tahara A, Kawashima H. 1995. Molecular cloning and characterization of rat V1b vasopressin receptor: Evidence for its expression in extra-pituitary tissues. Biochem Biophys Res Commun. 212:751–757.
- Salomé N, Stemmelin J, Cohen C, Griebel G. 2006. Differential roles of amygdaloid nuclei in the anxiolytic- and antidepressant-like effects of the V1b receptor antagonist, SSR149415, in rats. Psychopharmacology. 187:237–244.
- Scattoni ML, McFarlane HG, Zhodzishsky V, Caldwell HK, Young WS, Ricceri L, Crawley JN. 2008. Reduced ultrasonic vocalizations in vasopressin 1b knockout mice. Behav Brain Res. 187:371–378.
- Schonberger M, Legget C, Kim SW, Hooker JM. 2010. Synthesis of [11C]SSR149415 and preliminary imaging studies using positron emission topography. Bioorg Med Chem Lett. 20:3103–3106.
- Scott JD, Miller MW, Li SW, Lin S-I, Vaccaro HA, Hong L, Mullins DE, Guzzi M, Wenstein J, Hodgson RA, Varty GB, Stamford AW, Chan T-Y, McKittrick BA, Greenlee WJ, Priestley T, Parker EM. 2009. Tetrahydroquinoline sulfonamides as vasopressin 1b receptor antagonists. Bioorg Med Chem Lett. 19:6018–6022.
- Serradeil-Le Gal C, Wagnon J, Simiand J, Griebel G, Lacour C, Guillon G, Barberis C, Brossard G, Soubrié P, Nisato D, Pascal M, Pruss R, Scatton B, Maffrand J-P, Le Fur G. 2002. Characterization of (2S,4R)-1-[5-chloro-1-[(2,4-dimethoxyphenyl)sulfonyl]-3-(2-methoxy-phenyl)-2-oxo-2,3-dihydro-1H-indol-3-yl]-4-hydroxy-N,N-dimethyl-2-pyrrolidine carboxamide (SSR149415), a selective and orally active vasopressin V1b receptor antagonist. J Pharmacol Exp Ther. 300:1122–1130.
- Serradeil-Le Gal C, Raufaste D, Derick S, Blankenstein J, Allen J, Pouzet B, Pascal M, Wagnon J, Ventura MA. 2007. Biological characterization of rodent and human vasopressin V1b receptors using SSR-149415, a nonpeptide V1b receptor ligand. Am J Physiol Regul Integr Comp Physiol. 293:R938–R949.
- Shimazaki T, Iijima M, Chaki S. 2006. The pituitary mediates the anxiolytic-like effects of the vasopressin V1B receptor antagonist, SSR149415, in a social interaction test in rats. Eur J Pharmacol. 543:63–67.
- Spiga F, Harrison L, Wood S, Knight D, Macsweeney C, Thomson F, Craighead M, Lightman SL. Blockade of the V1b receptor reduces ACTH, but not corticosterone, secretion induced by stress without effecting basal HPA axis activity. J Endocrinol. 2009a; 200:273–283.
- Spiga F, Harrison L, Macsweeney C, Thomson F, Craighead M, Lightman S. Effect of vasopressin 1b receptor blockade on the hypothalamic–pituitary–adrenal response of chronically stressed rats to a heterotypic stressor. J Endocrinol. 2009b; 200:285–291.
- Stemmelin J, Lukovic L, Salomé N, Griebel G. 2005. Evidence that the lateral septum is involved in the antidepressant-like effects of the vasopressin V1b receptor antagonist, SSR149415. Neuropsychopharmacology. 30:35–42.
- Stewart LQ, Roper JA, Young WS, O'Carroll A-M, Lolait SJ. Pituitary–adrenal response to acute and repeated mild restraint, forced swim and change in environment stress in arginine vasopressin receptor 1b knockout mice. J Neuroendocrinol. 2008a; 20:597–605.
- Stewart LQ, Roper JA, Young WS, O'Carroll A-M, Lolait SJ. The role of the arginine vasopressin Avp1b receptor in the acute neuroendocrine action of antidepressants. Psychoneuroendocrinology. 2008b; 33:405–415.
- Subburaju S, Aguilera G. 2007. Vasopressin mediates mitogenic responses to adrenalectomy in the rat anterior pituitary. Endocrinology. 148:3102–3110.
- Tanoue A, Ito S, Honda K, Oshikawa S, Kitagawa Y, Koshimizu T-A, Mori T, Tsujimoto G. 2004. The vasopressin V1b receptor critically regulates hypothalamic–pituitary–adrenal axis activity under both stress and resting conditions. J Clin Invest. 113:302–309.
- Thibonnier M, Coles P, Thibonnier A, Shoham M. 2001. The basic and clinical pharmacology of nonpeptide vasopressin receptor antagonists. Annu Rev Pharmacol Toxicol. 41:175–202.
- Vaccari C, Lolait SJ, Ostrowski NL. 1998. Comparative distribution of vasopressin V1b and oxytocin receptor messenger ribonucleic acids in brain. Endocrinology. 139:5015–5033.
- Ventura MA, René P, de Keyzer Y, Bertagna X, Clauser E. 1999. Gene and cDNA cloning and characterization of the mouse V3/V1b pituitary vasopressin receptor. J Mol Endocrinol. 22:251–260.
- Volpi S, Rabadan-Diehl C, Cawley N, Aguilera G. 2002. Transcriptional regulation of the pituitary vasopressin V1b receptor involves a GAGA-binding protein. J Biol Chem. 277:27829–27838.
- Volpi S, Rabadán-Diehl C, Aguilera G. Regulation of vasopressin V1b receptors and stress adaptation. Ann N Y Acad Sci. 2004a; 1018:293–301.
- Volpi S, Rabadan-Diehl C, Aguilera G. Vasopressinergic regulation of the hypothalamic pituitary adrenal axis and stress adaptation. Stress. 2004b; 7:75–83.
- Wernet W, Hornberger WB, Unger LV, Meyer AH, Netz A, Oost T, Hillen H, Hahn A, Schoemaker H, Backfish G, Beyerbach A, Sullivan JP. 2008. In vitro characterization of the selective vasopressin V1b receptor antagonists ABT-436 and ABT-558. In: Proceedings of Society for Neuroscience, Washington DC, USA. Abstract#560.16/FF4.
- Wersinger SR, Ginns EI, O'Carroll A-M, Lolait SJ, Young WS. Vasopressin V1b receptor knockout reduces aggressive behavior in male mice. Mol Psychiatry. 7:975–984.
- Wersinger SR, Kelliher KR, Zufall F, Lolait SJ, O'Carroll A-M, Young WS. 2004. Social motivation is reduced in vasopressin 1b receptor null mice despite normal performance in an olfactory discrimination task. Horm Behav. 46:638–645.
- Wersinger SR, Caldwell HK, Christiansen M, Young WS. Disruption of the vasopressin 1b receptor gene impairs the attack component of aggressive behavior in mice. Genes Brain Behav. 2007a; 6:653–660.
- Wersinger SR, Caldwell HK, Martinez L, Gold P, Hsu SB, Young WS. Vasopressin 1a receptor knockout mice have a subtle olfactory deficit but normal aggression. Genes Brain Behav. 2007b; 6:540–551.
- Wersinger SR, Temple JL, Caldwell HK, Young WS. 2008. Inactivation of the oxytocin and the vasopressin (Avp) 1b receptor genes, but not the Avp 1a receptor gene, differentially impairs the Bruce effect in laboratory mice (Mus musculus). Endocrinology. 149:116–121.
- van West D, Del-Favero J, Aulchenko Y, Oswald P, Souery D, Forsgren T, Sluijs S, Bel-Kacem S, Adolfsson R, Mendlewicz J, van Duijn C, Deboutte D, van Broeckhoven C, Claes S. 2004. A major SNP haplotype of the arginine vasopressin 1B receptor protects against recurrent major depression. Mol Psychiatry. 9:287–292.
- van West D, Del-Favero J, Deboutte D, van Broeckhoven C, Claes S. 2009. Arginine vasopressin receptor-gene based single-nucleotide polymorphism analysis in attention deficit hyperactivity disorder. Psychiatr Genet. 19:102–103.
- de Wied D, Diamant M, Fodor M. 1993. Central nervous system effects of the neurohypophyseal hormones and related peptides. Front Neuroendocrinol. 14:251–302.
- Winslow JT, Hearn EF, Ferguson J, Young LJ, Matzuk MM, Insel TR. 2000. Infant vocalization, adult aggression, and fear behaviour of an oxytocin null mutant mouse. Horm Behav. 37:145–155.
- Yang M, Scattoni ML, Zhodzishsky V, Chen T, Caldwell H, Young WS, McFarlane HG, Crawley JN. 2007. Social Approach Behaviors are Similar on Conventional Versus Reverse Lighting Cycles, and in Replications Across Cohorts, in BTBR T+ tf/J, C57BL/6J, and Vasopressin Receptor 1B Mutant Mice. Front Behav Neurosci. 1:1–9.
- Yibchok-anun S, Hsu WH. 1998. Effects of arginine vasopressin and oxytocin on glucagon release from clonal alpha-cell line In-R1-G9: Involvement of V1b receptors. Life Sci. 63:1871–1878.
- Yibchok-anun S, Cheng H, Heine PA, Hsu WH. 1999. Characterization of receptors mediating AVP- and OT-induced glucagon release from the rat pancreas. Am J Physiol. 277:E56–E62.
- Yibchok-anun S, Abu-Basha EA, Yao C-Y, Panichkriangkrai W, Hsu WH. 2004. The role of arginine vasopressin in diabetes-associated increase in glucagon secretion. Regul Pept. 122:157–162.
- Young WS, Li J, Wersinger SR, Palkovits M. 2006. The vasopressin 1b receptor is prominent in the hippocampal area CA2 where it is unaffected by restraint stress or adrenalectomy. Neuroscience. 143:1031–1039.
- Young SF, Griffante C, Aguilera G. 2007. Dimerization between vasopressin V1b and corticotropin releasing hormone type 1 receptors. Cell Mol Neurobiol. 27:439–461.
- Zelena D, Foldes A, Mergl Z, Barna I, Kovacs KJ, Makara GB. 2004. Effects of repeated restraint stress on hypothalamo–pituitary–adrenocortical function in vasopressin deficient Brattleboro rats. Brain Res Bull. 63:521–530.
- Zelena D, Domokos A, Barna I, Mergl Z, Haller J, Makara GB. 2008. Control of the hypothalamo–pituitary–adrenal axis in the neonatal period: Adrenocorticotropin and corticosterone stress responses dissociate in vasopressin-deficient Brattleboro rats. Endocrinology. 149:2576–2583.
- Zelena D, Domokos A, Jain S, Jankord R, Filaretova L. 2009. The stimuli-specific role of vasopressin in the hypothalamo–pituitary–adrenal axis response to stress. J Endocrinol. 202:263–278.
- Zhou Y, Leri F, Cummins E, Hoeschele M, Kreek MJ. 2008. Involvement of arginine vasopressin and V1b receptor in heroin withdrawal and heroin seeking precipitated by stress and by heroin. Neuropsychopharmacology. 33:226–236.