Abstract
During the perinatal period, the developing brain is sensitive to environmental events. Deleterious programing resulting from infection, dietary restriction, or psychological stress has been observed and affects adult immune and endocrine systems as well as behavior. In this study, we determined whether neonatal infection permanently alters immune and glucocorticoid receptor signaling pathways in the adult hippocampus. A Chlamydia muridarum respiratory infection was induced in male and female mice at birth. Mice were allowed to recover and microarray analysis was conducted on RNA from adult hippocampal tissue. In males, neonatal infection induced an up-regulation of genes associated with cellular development, nervous system development and function, such as cyclin-dependent kinase inhibitor 1A. After neonatal infection, adult females exhibited a T-helper 2 immune bias with genes such as major histocompatibility complex, class II, DQ beta 1 up-regulated. Expression of prolactin, vasopressin, hypocretin, corticotrophin-releasing hormone-binding protein, and oxytocin were confirmed by quantitative real-time polymerase chain reaction. This study shows that neonatal infection differentially alters the gene expression profiles of both female and male mice along immune and neuroendocrine pathways.
Introduction
The impact of early life insults on adult physiology is well established (Strachan Citation1989; McMillen and Robinson Citation2005). Events such as infection, dietary restriction, or psychological stress have the capacity to detrimentally affect adult immune and endocrine systems as well as behavior (Strachan Citation2000; Buitelaar et al. Citation2003; McGowan et al. Citation2008). The mechanisms underlying these long-term effects of early life programing have been investigated using a variety of paradigms including neonatal exposure to pathogens. Up to one-third of pregnancies suffer complications involving infections or trauma of the fetus or newborn (Garnier et al. Citation2003) and an increasing body of evidence indicates that perinatal events involving the immune system may contribute to the development of adult disease (Shanks et al. Citation1995, Citation2000; Hall and Peckham Citation1997). Furthermore, the immune system in the neonatal period is not fully developed and consequently the neonate is more at risk of infection than an adult, with newborns particularly susceptible to bacterial and fungal infections (Garvy Citation2003). Nevertheless, the long-term impact of early life infection and its effect on adult health remains incompletely understood. Therefore, it is important to examine the impact of pre- and post-natal infection on adult physiology and programing to determine the mechanisms leading to disease and to explore potential interventions.
Chlamydiae are common human pathogens in all age groups (Hammerschlag Citation2000) and frequently cause trachoma, sexually transmitted diseases, and pneumonia (Stephens Citation2003). We have established a mouse model of subacute respiratory infection using Chlamydia muridarum, which naturally infects mice and is an appropriate choice for investigating natural host–pathogen interactions. The temporal, histological, and immunological progression of disease is typical of the immunopathological reactions that are representative of human infection and disease caused by chlamydiae (Adkins et al. Citation1993). We have used this model to show that C. muridarum infection during the neonatal period has long-term effects on the immune and respiratory systems. Infection affects the development of allergic airways disease and is associated with altered T helper 1 (Th1) and T helper 2 (Th2) responses in the adult (Horvat et al. Citation2007, Citation2010). C. muridarum infection activates the stress response as seen by excess glucocorticoids (GCs) circulating in the developing neonate (Ruzek et al. Citation1999). However, whether infection in early life can induce alterations in the stress response and immunological programing in the brain later in life remains unknown. In this study, we have examined the effects of neonatal respiratory C. muridarum infection on hippocampal gene expression. This was achieved by microarray profiling of adult hippocampal tissue and the assessment of infection-induced changes to GC receptor (GR) signaling pathways and immune response indicators.
Methods
Animals
All animals were housed in an accredited animal care facility under specific pathogen-free conditions. Pregnant female BALB/c mice were obtained from the central animal house and used with approval from the animal ethics committee, University of Newcastle (NSW, Australia). Dams were left undisturbed until birth of the young. Offspring were weaned and sexed at 21 days of age. Animals were given access to food and water ad libitum, and housed either per litter (mothers with young) or in groups not larger than six (adult and weaned young) under controlled environmental conditions with a 12:12 h light:dark cycle. Samples were taken from an experimental pool of 64 mice born to 11 mothers. Litter sizes were between 2 and 10 mice; resulting in 31 male and 33 female mice from five infected litters and six uninfected litters. Individual litters were not kept separate. The experimental pool consisted of a mix of mice from different litters of different sizes. Non-infected mice were not litter mates of infected mice. The microarray study was conducted using pools of six mice from each treatment condition and sex (i.e. male control, male infected, female control, and female infected). The follow-up reverse transcription (RT) of complementary DNA (cDNA) and quantitative real-time polymerase chain reaction (qRT PCR) was completed using RNA from 10 mice in each treatment condition and sex.
Infection protocol and tissue collection
The C. muridarum strain ATCC VR-123 (formerly known as the mouse pneumonitis biovar of C. trachomatis) was obtained from the American Type Culture Collection (ATCC, Manassas, VA, USA). Stocks were stored at − 80°C in sucrose–phosphate–glutamate buffer (SPG). Within 24 h of birth, neonatal mice were infected intranasally with C. muridarum without anesthesia. Mice were held in the upright position and the inoculum (400 inclusion-forming units; ifu) in 5 μl of SPG was gently pipetted onto the nares until the whole inoculum was inhaled (Horvat et al. Citation2007, Citation2010). Control groups were sham-inoculated with 5 μl of SPG. We have previously shown that infection peaks between 10 and 15 days after inoculation, bacterial clearance occurs by day 21, and pulmonary inflammation is largely resolved by day 45 (Horvat et al. Citation2007). Mice were allowed to recover from the infection and mature into adults. At 63 days of age, mice were euthanized by sodium pentobarbital overdose (Abbott Australasia, Kurnell, Australia) and the brain was removed and placed in RNAlater (Applied Biosystems/Ambion, Austin, TX, USA) solution and stored at − 80°C.
Parallel RNA/DNA extractions and purification
Parallel RNA/DNA extractions were carried out on the whole hippocampal tissue. The hippocampus was identified by referring to the Allen Mouse Brain Atlas (www.brain-map.org, Lein et al. Citation2007). For the microarray portion of the study, RNA from six mice was pooled per treatment group (infection and control) and sex (male and female). For the follow-up qRT PCR, 10 mice per treatment group and sex were used. RNA was extracted using RNA/DNA mini kit according to the manufacturer's instruction. Purification of RNA was carried out using an RNeasy mini kit (Qiagen, Hilden, Germany) with DNase treatment using an RNase-free DNase set (Qiagen). RNA concentration was determined by spectrophotometer (NanoDrop 3300, Thermo Scientific, Wilmington, DE, USA) and the quality was assessed by agarose gel electrophoresis.
Microarray profiling
For the microarray portion of the study, the RNA was sent for gene profiling at an external facility [Australian Genome Research Facility (AGRF), St Lucia, QLD, Australia]. Samples were pooled by sex and treatment with six mice in each treatment/sex condition. Microarray profiling was performed using the Illumina MouseWG-6 v1.1 Expression BeadChip, one chip per pooled sample, which then was analyzed using an Illumina BeadArray Reader. The multi-sample format of the chip allows profiling of more than 45,200 transcripts and six samples simultaneously on a single BeadChip. The resulting data were normalized by quantile normalization after variance-stabilizing transformation, using Lumi package (http://www.basic.northwestern.edu/projects/lumi/) in the R statistical software (Du et al. Citation2008) and were subsequently imported into GeneSpring GX 7 (Agilent Technologies, VIC, Australia) for further analysis. Comparisons were of infected vs. control groups for the same sex; male infected vs. male control and female infected vs. female control. Differentially expressed genes were identified as those showing a ≥ 50% difference in mean expression levels between groups. There were no replicates; therefore, no statistical test (such as t-test) was possible.
To identify gene networks and signaling pathways containing differentially expressed genes between conditions, we used ingenuity pathway analysis (IPA; Ingenuity® Systems, Redwood City, CA, USA, www.ingenuity.com/). IPA is a web-based software which organizes differentially expressed genes into molecular pathways and functional groupings based on the published literature, and the software enables examination of affected canonical signaling pathways. Functional analysis in IPA identifies the published biological functions that were most significantly associated with the genes in the network. Genes are represented as nodes, and the biological relationship between two nodes is represented by as a line. All lines are supported by at least one reference in the literature, textbook, or from canonical information stored in the ingenuity pathway's knowledge database. IPA also allows the overlay of specific signaling pathways on identified networks. In this study, we overlaid the GR signaling pathway onto the top gene networks identified by IPA.
Rt Of Cdna And Qrt-pcr
RT was carried out following the manufacturer's instructions using Superscript First-Strand Synthesis System III for RT-PCR kit (Invitrogen, Carlsbad, CA, USA). qRT-PCR was used to determine mRNA abundance of several genes, which were compared to the levels of the constitutively expressed gene, β-actin. Primer sequences were designed with the Primer Express program (Applied Biosystems, Warrington, UK: ) and products were sequenced at an external facility (AGRF). qRT PCR was performed using the supplied program on a PCR 7700 sequence detector (Applied biosystems) in duplicate or triplicate under the following reaction conditions: 2 min at 50°C, 10 min at 95°C, and 40 cycles of 15 s at 95°C and 1 min at 60°C. A single or duplicate sample that had not been reverse transcribed was included as a control for each sample, along with single or duplicate controls without template for each primer. Data were analyzed using the ABI Prism Sequence Detection Systems software version 1.9 (Applied Biosystems). The comparative CT method (where CT is the threshold cycle) was used to derive a relative quantitative measure of the target gene expression compared with β-actin mRNA.
Table I. Primer details for target and reference genes.
Data analysis
qRT PCR data were analyzed by between subjects two-factor full factorial ANOVA using the PASW software (SPSS Inc., Chicago, IL, USA), with the factors being treatment (control and post-natal infection) and sex (male and female), the significance level was set at p ≤ 0.05. Data were log transformed before the analysis. Post hoc analysis was performed within each sex if the two-way ANOVA showed a significant interaction between sex and treatment. Individual data were removed from the analysis if individual samples returned gene expression below detectable levels after qRT PCR.
Results
Overall gene changes
In male hippocampus, 392 (100%) genes were differentially expressed after neonatal infection with C. muridarum; of those genes, 134 (34%) were increased in expression by more than 1.5-fold and 258 (66%) decreased in expression by 0.5-fold. In female adult hippocampus, 541 genes were differentially expressed, of which 330 (61%) were increased in expression by more than 1.5-fold and 211 (39%) were decreased in expression by 0.5-fold. Of the genes differentially expressed, 90 were commonly expressed in males and females; 276 were specific to males and 409 were specific to females.
Genes differentially expressed in male adult mice after neonatal infection
The IPA analysis was conducted on all genes that were differentially expressed in the adult hippocampus after neonatal C. muridarum infection. The five most substantially altered gene networks in the adult male hippocampus are shown in . The specific networks identified were associated with cellular development, nervous system development and function, neurological disease, cellular compromise, and tissue morphology. In network 1, 14 genes were differentially expressed and associated with tissue morphology, cellular development, and connective tissue development and function (). For example, hippocampal 11 β-hydroxysteroid dehydrogenase 1 (11β HSD1) gene expression was increased by 1.63-fold following male neonatal infection and was identified as being indirectly regulated by Interleukin 4 (IL-4; ).
Table II. Networks identified of genes differentially expressed in male adult mice after neonatal infection (bold indicates up-regulation).
Figure 1. Network 1 identified by IPA showing differentially expressed genes in male adult hippocampus after neonatal respiratory C. muridarum infection. Red shading indicates up-regulation of the gene. No color indicates genes that form the network and have no change in expression in the present study. Fourteen genes were differentially expressed and associated with tissue morphology, cellular development, and connective tissue development and function. For example, 11β-HSD1 gene expression was altered following male neonatal infection and was indirectly regulated by IL-4. For full names of genes involved in the network, see .
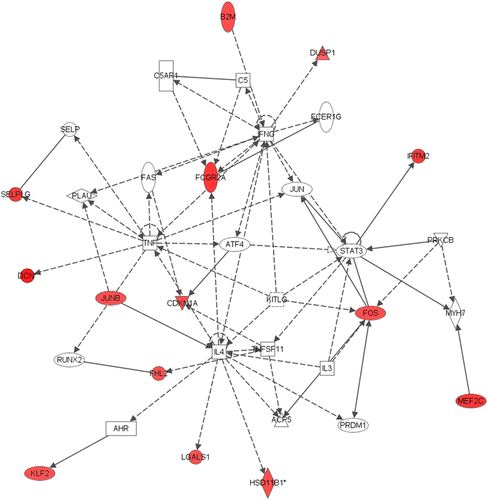
Genes differentially expressed in female adult mice after neonatal infection
The five most substantially altered gene networks in females are shown in . The female-specific networks identified were associated with cell-to-cell signaling, immune cell trafficking, cell death, cellular movement, cancer, as well as cellular growth and proliferation. In network 1, 13 genes were differentially expressed after infection and were identified as indirectly involved with cytokine regulation as shown in . For example, major histocompatibility complex, class II, DQ beta 2 (HLA-DQβ2) was increased by 1.68-fold following infection and identified to indirectly regulate IL-6 and IL-10 (). In network 4 (), 10 genes were differentially expressed including tyrosine hydroxylase (1.60-fold increase), a rate-limiting enzyme in catecholamine synthesis.
Table III. Networks identified of genes differentially expressed in female adult mice after neonatal infection (bold indicates up-regulation; italic indicates down-regulation).
Table IV. Definitions of abbreviations used in figures and tables.
Figure 2. Network 1 identified by IPA showing differentially expressed genes in female adult hippocampus after neonatal respiratory C. muridarum infection. Red shading indicates up-regulation of the gene. Gray shading indicates down-regulation. No color indicates genes that form the network and have no change in expression in the present study. Thirteen genes were differentially expressed after infection and were indirectly involved with cytokine regulation. For example, HLA DQB2 was increased following infection and identified to indirectly regulate IL-6 and IL-10. For full names of genes involved in the network, see .
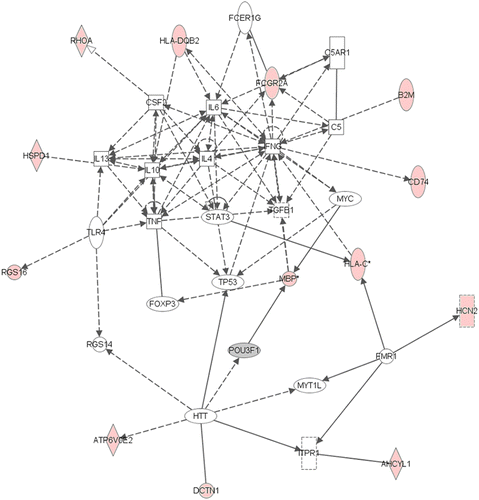
Differential expression of genes related to GR signaling pathway
Using IPA, the relationship between the GR signaling pathway and the networks listed for male adult hippocampus after neonatal C. muridarum infection was examined and two networks were related to the GR pathway. Network 1 ( and ) showed eight genes regulated by the GR pathway. The genes were related to inflammatory response and cell regulation. Two implicated genes were up-regulated after infection, FOS (v-fos FBJ murine osteosarcoma viral oncogene homolog; 1.60-fold) and cyclin-dependent kinase inhibitor 1A (CDKN1A; 1.605-fold).
The relationship between the GR signaling pathway and the networks listed for female adult hippocampus after neonatal C. muridarum infection was examined. In female adult hippocampus after infection, within network 1 ( and ), the GR signaling pathway interacted with nine genes including IL-4, IL-6, IL-10, tumor necrosis factor α (TNFα), interferon (IFN)-γ, and signal transducer and activator of transcription (STAT)-3 which are immune-related genes. In network 2 (), small ubiquitin-like modifier-1 was up-regulated (1.61-fold) and this gene interacts directly with the GR signaling pathway as defined by the IPA. In network 3, prolactin was increased (7.51-fold) and identified as directly regulated by GR signaling pathway (). Other components of this network; STAT-1, STAT-3, and CCAAT/enhancer-binding protein (C/EBP) were also identified as part of the GR signaling pathway.
Confirmation of gene changes in individual hippocampal mRNA
Confirmation of the microarray results by qRT PCR was performed on a number of genes. Candidate genes were chosen a priori according to the following specifications, if the microarray data analysis showed high fold changes; if the genes were altered in each treatment/sex condition; and if the genes were linked to GR/HPA functioning. The genes selected were prolactin, vasopressin, hypocretin, corticotrophin-releasing hormone-binding protein (CRH BP), and oxytocin. After neonatal infection, males had an increase in CRH BP (1.78-fold); and decreases in vasopressin (0.44-fold), hypocretin (0.30-fold), and oxytocin (0.24-fold). Females had increases in prolactin (7.51-fold), oxytocin (4.92-fold), hypocretin (9.51-fold), and vasopressin (13.07-fold) after neonatal infection. compares gene expression changes found by microarray to gene expression changes found by qRT PCR and shows the results of the pairwise comparison by treatment conducted after ANOVA.
Table V. Direction of change in mRNA in microarray (fold change) and qRT PCR (relative abundance) with pairwise comparison across treatment for qRT PCR.
The two-way ANOVA showed that prolactin mRNA abundance was unchanged by neonatal infection for both sexes (). For vasopressin mRNA, the ANOVA revealed main effects of sex, F(1,35) = 90.1, p < 0.01, and treatment, F(1,36) = 48.89, p < 0.01, with a significant interaction, F(1,35) = 96.24, p < 0.01. Males had significantly less vasopressin mRNA after infection when compared to same sex controls, p < 0.01 (). Females had significantly more vasopressin mRNA after infection when compared to same sex controls, p = 0.05.
Figure 3. Expression of target mRNA relative to β-actin (log) in adult male and female hippocampus after neonatal respiratory infection with C. muridarum compared to sham-infected controls. The expression of (a) prolactin, (b) vasopressin, (c) hypocretin, (d) CRH-BP, and (e) oxytocin.
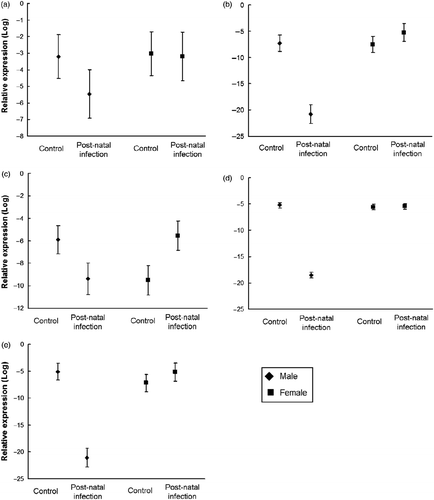
A two-way ANOVA revealed a significant interaction of sex and treatment for hypocretin mRNA expression after neonatal infection, F(1,32) = 33.53, p < 0.01. Infected males had a significant reduction in hypocretin, p < 0.01, and females had significant increase compared with uninfected controls, p < 0.01 (). There was significant main effects of sex, F(1,36) = 562.14, p < 0.01 and treatment, F(1,36) = 602.45, p < 0.01 and the interaction was significant, F(1,36) = 624.91, p < 0.01 for CRH BP. In males, infection resulted in significantly less CRH BP than controls, p < 0.01. However, there was no significant difference in CRH BP abundance due to treatment in females, p = 0.75 (). The between-subjects ANOVA for oxytocin mRNA revealed a main effect of sex, F(1,35) = 72.74, p < 0.01, and treatment, F(1,35) = 73.44, p < 0.01 with the interaction also significant, F(1,35) = 122.88, p < 0.01. In males, oxytocin mRNA was significantly reduced following neonatal infection relative to the controls, p < 0.01, whereas expression was increased in females, but not significantly, p = 0.09 ().
Discussion
The rate of infection is high in newborns due to their relatively immature, naive immune system (Garvy Citation2003) and our results demonstrate that early life infection significantly alters adult hippocampal gene expression in a sex-specific manner. The data indicate that adult gene expression profiles were altered with males that were exposed to infection early in life, exhibiting gene changes associated with tissue morphology or cellular development such as CDKN1A, a regulator of cell cycle progression, when compared to male controls. In contrast, females exposed to infection in early life exhibited a Th2 cytokine bias with an up-regulation of genes related to humoral immunity such as IL-6 and IL-10, when compared to females in the control condition.
Perinatal stress can modify the hippocampal gene expression. Previous studies have examined the effects of pre- and post-natal stress, including psychological (Vallee et al. Citation1999; Szuran et al. Citation2000; Tuchscherer et al. Citation2004; Kapoor et al. Citation2008) and physiological stress (Sutanto et al. Citation1996; Liu et al. Citation1997; Dodic et al. Citation2002; Walker et al. Citation2004b, Citation2009; Shoener et al. Citation2006); and have found that early life stressors permanently alter hippocampal corticosteroid receptor gene expression. These studies report that early life stress leads to a reduction in GR and mineralocorticoid receptor (MR) expression (Sutanto et al. Citation1996; Dodic et al. Citation2002; Kapoor et al. Citation2008), resulting in anxiety-like behavior (Walker et al. Citation2004b, Citation2009), learning deficits (Vallee et al. Citation1999; Szuran et al. Citation2000), and altered stress responsivity in adulthood (Liu et al. Citation2001; Walker et al. Citation2009). This suggests that the GR/MR signaling pathway may be permanently altered by early life stress. The present study aimed to expand on previous research by examining potential global gene changes and exploring the relationship of these gene alterations to the GR signaling pathway.
The IPA analysis of the adult male hippocampus data indicated that the genes differentially expressed compared to male controls had limited interaction with the GR signaling pathway with only 10 genes in all the networks identified as related to GR signaling, suggesting that in the males, GR-related genes were relatively unaffected by neonatal infection. One exception was 11β-HSD1 which was shown to be up-regulated after neonatal infection. In the hippocampus, 11β-HSD1 converts the inactive 11-dehydrocorticosterone to the active corticosterone (Rajan et al. Citation1996). 11β-HSD1 was up-regulated after infection which would increase the amount of corticosterone in the hippocampus, thereby decreasing the amount of GR and MR. This suggests that in the males, hippocampal function may be altered through genes not directly related to the GR signaling pathway.
Of the networks altered in female adult hippocampi after infection, the GR signaling pathway interacted with many genes including IL-6, IL-10, TNFα, IFN-γ, and STAT-3, with those Th2 factors associated with up-regulated genes. Prolactin was up-regulated and identified as being directly regulated by GRs. Other genes such as STAT-1, STAT-3, and C/EBP were also identified as part of the GR signaling pathway by regulating or being regulated by GRs (Zhang et al. Citation1997; Boruk et al. Citation1998). This suggests a pervasive up-regulation of genes linked to the GR signaling pathway after neonatal infection in the female rodent.
Generally, with age comes a shift from an IL-10 immune dominance to an IL-4 immune dominance (Pettiford et al. Citation2002), and in the present study, the males show evidence of this shift, whereas the females show evidence of IL-10 and IL-6 dominance. This suggests that for male mice, immune organization was not changed by neonatal infection, whereas females had their immune profile altered. Clinically, many diseases and disorders occur in sex-specific patterns, for example women are two to three times more likely than men to present with affective disorders, autism affects four times as many boys as girls, and multiple sclerosis and lupus erythematosus are more prevalent in women (Bale Citation2009). Although it is well established that early life events program the functioning of physiological systems (Hales et al. Citation1991; Meaney et al. Citation1996; Hodgson et al. Citation2001; Welberg et al. Citation2001; Walker et al. Citation2009; Horvat et al. Citation2010), there is a gap in the knowledge of the role sex plays in determining adult outcomes after perinatal immune activation.
The present study shows that it is necessary to examine both males and females, due to their physiological differences it is unsuitable to extrapolate the results of one to the other. Primary targets that may account for such differences are, most obviously, the sex hormones. Human studies have shown that in response to stress, (i.e. the trier social stress test), men have a higher adrenocorticotropic hormone (ACTH) response to the stressor compared with women, but the plasma cortisol response is the same in both men and women (Kirschbaum et al. Citation1999; Young and Korszun Citation2010). However, the female cortisol response is dependent on the menstrual cycle phase (Young et al. Citation1991; Young and Korszun Citation2010). This response appears to be due to more than just the influence of estrogens. The differences are related to levels of progesterone, with decreases in progesterone resulting in cortisol suppression (Young et al. Citation1991). Estradiol influences hypothalamic–pituitary–adrenal (HPA) axis functioning and has been shown to decrease stress responsiveness in rodents (Young et al. Citation2001), sheep (Komesaroff et al. Citation1998), and humans (Komesaroff et al. Citation1999). Human studies suggest that estradiol acts as a ‘brake’ on the HPA axis response and that progesterone impairs GC negative feedback (Young and Korszun Citation2010).
In the present study, confirmation by qRT-PCR of target genes found that for the males, the microarray data were supported for all genes tested except CRH-BP and prolactin. For the females, the findings of the microarray data were supported with a significant increases in vasopressin, hypocretin, and oxytocin mRNA. Overall, this study provides evidence of the effects of early life infection on adult gene expression and provides important information of potential gene and network targets, as seen in , that may be investigated to further understand the impact of perinatal infection on adult immune functioning and stress responsivity.
The focus genes for follow-up qRT PCR were chosen due to the high fold changes seen in the microarray and their proposed role in the GR/HPA functioning. Hypocretin is involved in the biostability of arousal states and hippocampal plasticity (Selbach et al. Citation2010), and neurons expressing hypocretin have been shown to be directly and indirectly regulated by catecholamines (Yamanaka et al. Citation2006). Vasopressin, along with CRH is one of the principal effectors of the stress system (Charmandari et al. Citation2003) and has been extensively studied in the hypothalamus, but not in the hippocampus. Females in our study showed an increase in vasopressin mRNA after infection, whereas the males showed a significant decrease. CRH has been shown to be neuroprotective in the hippocampus (Pedersen et al. Citation2001; Elliott-Hunt et al. Citation2002) and we found that males had less CRH BP after infection, thereby increasing the availability of CRH in the hippocampus. This suggests that males may be more protected from stress-related excitotoxicity. Oxytocin has been shown to be regulated by GCs with decreases in GCs resulting in decreases of oxytocin in the hippocampus (Liberzon et al. Citation1994). Also, oxytocin may override the stress response by eliminating the hypothalamic response to stress (Lightman Citation1992). More recent data support this theory, as oxytocin has been shown to regulate GR and MR in the hippocampus with increases in oxytocin resulting in decreased GR and MR (Petersson and Uvnas-Moberg Citation2003). Our follow-up qRT PCR showed after infection that females had higher oxytocin mRNA, whereas males had significantly less oxytocin. Overall, the data are further evidence of the complex influence of sex on potential health outcomes. The gene expression profiles of both female and male mice were altered by neonatal infection, but along differing immune and neuroendocrine pathways.
There are a number of possible alternate explanations for our results; one factor not accounted for is the influence of maternal behavior on adult outcomes. Any alterations to endocrine activity may be in part mediated by the impact of infection on maternal behavior. In rodent studies, an attentive dam results in offspring with reduced plasma ACTH and corticosterone, increased hippocampal GR, and enhanced negative feedback sensitivity when compared to less attentive dams (Liu et al. Citation1997; Francis et al. Citation1999). Although there has been much research on the level of maternal care and the response to adult stressors, few studies have assessed maternal care after neonatal infection. Dams of pups exposed to endotoxin have been found to display significantly less arched-back nursing with significantly more time spent off nest (Walker et al. Citation2004a). Others have found endotoxin exposure to have no effect on maternal behavior (Spencer et al. Citation2006). During the present study, maternal behavior was not assessed, as such we cannot rule out maternal care as a contributing factor. Therefore, future studies should assess maternal behavior in parallel with the offspring's functional and physiological parameters.
The use of microarray in the present study was largely exploratory with a focus on potential changes to GR-related genes; as such the results highlight many areas for further study. For example, given both males and females showed changes to dopamine-related genes after infection, and a closer examination of dopamine-responsive hormones/neuropeptides is warranted. The results of the microarray analysis also showed changes to 11β-HSD1 and genes related to immune function which were not directly measured by qRT PCR. In addition, the analysis of transcription factors that could mediate the impact of infection on hippocampal gene expression may help to elucidate mechanisms behind the changes seen in the present study. Given the limited number of genes confirmed by qRT PCR, the present findings should be regarded as a preliminary indication of adult outcomes after neonatal infection.
Much of the previous work that has investigated immune factors and the hippocampus has focused on the role of the immune response in the development of Alzheimer's disease, specifically the potential preventative effects of a Th2 bias. It has been found that the administration of a Th2-biased Amyloid β-specific T-cell vaccine leads to a significant decrease in plaque-associated microglia and less vascular amyloidosis (Cao et al. Citation2009). The beneficial effects are only observed with the induction of a Th2 immune response. This is evidenced by the original human trials being halted due to the adverse effects of inflammatory Th1 responses caused by components in the formula of the vaccine (Gilman et al. Citation2005); subsequent trials with an altered formula for the vaccine did not result in any Th1 immune response (Bayer et al. Citation2005). In the present study, both sexes show a pervasive up-regulation of immune-related genes with males having more genes related to gene transcription and cellular development, and females showing a higher number of altered immune-related genes with a Th2 bias. The study provides evidence that the altered immune profile after neonatal infection persists and may indicate future directions for preventative intervention of neurodegenerative disease.
Early life infection has been found to result in altered patterns of neurogenesis in the hippocampus (Sharma et al. Citation2002; Cui et al. Citation2009), deficits in long-term potentiation (Lante et al. Citation2007; Lowe et al. Citation2008), and decreased hippocampal volume (Ikeda et al. Citation2005; Fatemi et al. Citation2009). These physiological outcomes result in increased anxiety-like behavior, and decreased learning and memory. Taking into account, the impact of early life infection on adult health predispositions could facilitate health practitioners in prevention, diagnosis, and treatment of adult disease. The present study shows that neonatal infection has effects on adult hippocampal gene expression that differ between males and females. It is clear that early life experiences influence brain development and physiology, which in turn has fundamental implications for an individual throughout their lifespan.
Acknowledgements
This work was funded by grants from the National Health and Medical Research Council of Australia (401238 and 569219).
Declaration of interest: The authors report no conflicts of interest. The authors alone are responsible for the content and writing of the paper.
References
- Adkins B, Ghanei A, Hamilton K. 1993. Developmental regulation of IL-4, IL-2, and IFN-gamma production by murine peripheral T lymphocytes. J Immunol. 151:6617–6626.
- Bale TL. 2009. Neuroendocrine and immune influences on the CNS: It's a matter of sex. Neuron. 64:13–16.
- Bayer AJ, Bullock R, Jones RW, Wilkinson D, Paterson KR, Jenkins L, Millais SB, Donoghue S. 2005. Evaluation of the safety and immunogenicity of synthetic Abeta42 (AN1792) in patients with AD. Neurology. 64:94–101.
- Boruk M, Savory JG, Hache RJ. 1998. AF-2-dependent potentiation of CCAAT enhancer binding protein beta-mediated transcriptional activation by glucocorticoid receptor. Mol Endocrinol. 12:1749–1763.
- Buitelaar JK, Huizink AC, Mulder EJ, de Medina PG, Visser GH. 2003. Prenatal stress and cognitive development and temperament in infants. Neurobiol Aging. 24 Suppl 1: S53–S60 discussion S67-58.
- Cao C, Arendash GW, Dickson A, Mamcarz MB, Lin X, Ethell DW. 2009. A beta-specific Th2 cells provide cognitive and pathological benefits to Alzheimer's mice without infiltrating the CNS. Neurobiol Dis. 34:63–70.
- Charmandari E, Kino T, Souvatzoglou E, Chrousos GP. 2003. Pediatric stress: Hormonal mediators and human development. Horm Res. 59:161–179.
- Cui K, Ashdown H, Luheshi GN, Boksa P. 2009. Effects of prenatal immune activation on hippocampal neurogenesis in the rat. Schizophr Res. 113:288–297.
- Dodic M, Hantzis V, Duncan J, Rees S, Koukoulas I, Johnson K, Wintour EM, Moritz K. 2002. Programming effects of short prenatal exposure to cortisol. Faseb J. 16:1017–1026.
- Du P, Kibbe WA, Lin SM. 2008. Lumi: A pipeline for processing Illumina microarray. Bioinformatics. 24:1547–1548.
- Elliott-Hunt CR, Kazlauskaite J, Wilde GJ, Grammatopoulos DK, Hillhouse EW. 2002. Potential signalling pathways underlying corticotrophin-releasing hormone-mediated neuroprotection from excitotoxicity in rat hippocampus. J Neurochem. 80:416–425.
- Fatemi SH, Folsom TD, Reutiman TJ, Huang H, Oishi K, Mori S. 2009. Prenatal viral infection of mice at E16 causes changes in gene expression in hippocampi of the offspring. Eur Neuropsychopharmacol. 19:648–653.
- Francis DD, Champagne FA, Liu D, Meaney MJ. 1999. Maternal care, gene expression, and the development of individual differences in stress reactivity. Ann N Y Acad Sci. 896:66–84.
- Garnier Y, Coumans AB, Jensen A, Hasaart TH, Berger R. 2003. Infection-related perinatal brain injury: The pathogenic role of impaired fetal cardiovascular control. J Soc Gynecol Investig. 10:450–459.
- Garvy BA. 2003. Host defense against pulmonary infection in neonates. Clin Appl Immunol Rev. 4:205–223.
- Gilman S, Koller M, Black RS, Jenkins L, Griffith SG, Fox NC, Eisner L, Kirby L, Rovira MB, Forette F, Orgogozo JM. 2005. Clinical effects of Abeta immunization (AN1792) in patients with AD in an interrupted trial. Neurology. 64:1553–1562.
- Hales CN, Barker DJ, Clark PM, Cox LJ, Fall C, Osmond C, Winter PD. 1991. Fetal and infant growth and impaired glucose tolerance at age 64. Br Med J. 303:1019–1022.
- Hall AJ, Peckham CS. 1997. Infections in childhood and pregnancy as a cause of adult disease-methods and examples. Br Med Bull. 53:10–23.
- Hammerschlag MR. 2000. Chlamydia pneumoniae and the lung. Eur Respir J. 16:1001–1007.
- Hodgson DM, Knott B, Walker FR. 2001. Neonatal endotoxin exposure influences HPA responsivity and impairs tumor immunity in Fischer 344 rats in adulthood. Pediatr Res. 50:750–755.
- Horvat JC, Beagley KW, Wade MA, Preston JA, Hansbro NG, Hickey DK, Kaiko GE, Gibson PG, Foster PS, Hansbro PM. 2007. Neonatal chlamydial infection induces mixed T-cell responses that drive allergic airway disease. Am J Respir Crit Care Med. 176:556–564.
- Horvat JC, Starkey MR, Kim RY, Phipps S, Gibson PG, Beagley KW, Foster PS, Hansbro PM. 2010. Early-life chlamydial lung infection enhances allergic airways disease through age-dependent differences in immunopathology. J Allergy Clin Immunol. 125 3: 617–625 625 e1-625 e6.
- Horvat JC, Starkey MR, Kim RY, Phipps S, Gibson PG, Beagley KW, Foster PS, Hansbro PM. 2010. Early-life chlamydial lung infection enhances allergic airways disease through age-dependent differences in immunopathology. J Allergy Clin Immunol. 125:617–625 e611-625, e616.
- Ikeda T, Mishima K, Aoo N, Liu AX, Egashira N, Iwasaki K, Fujiwara M, Ikenoue T. 2005. Dexamethasone prevents long-lasting learning impairment following a combination of lipopolysaccharide and hypoxia-ischemia in neonatal rats. Am J Obstet Gynecol. 192:719–726.
- Kapoor A, Leen J, Matthews SG. 2008. Molecular regulation of the hypothalamic–pituitary–adrenal axis in adult male guinea pigs after prenatal stress at different stages of gestation. J Physiol. 586:4317–4326.
- Kirschbaum C, Kudielka BM, Gaab J, Schommer NC, Hellhammer DH. 1999. Impact of gender, menstrual cycle phase, and oral contraceptives on the activity of the hypothalamus-pituitary-adrenal axis. Psychosom Med. 61:154–162.
- Komesaroff PA, Esler M, Clarke IJ, Fullerton MJ, Funder JW. 1998. Effects of estrogen and estrous cycle on glucocorticoid and catecholamine responses to stress in sheep. Am J Physiol. 275:E671–E678.
- Komesaroff PA, Esler MD, Sudhir K. 1999. Estrogen supplementation attenuates glucocorticoid and catecholamine responses to mental stress in perimenopausal women. J Clin Endocrinol Metab. 84:606–610.
- Lante F, Meunier J, Guiramand J, Maurice T, Cavalier M, de Jesus Ferreira MC, Aimar R, Cohen-Solal C, Vignes M, Barbanel G. 2007. Neurodevelopmental damage after prenatal infection: Role of oxidative stress in the fetal brain. Free Radic Biol Med. 42:1231–1245.
- Lein ES, Hawrylycz MJ, Ao N, Ayres M, Bensinger A, Bernard A, Boe AF, Boguski MS, Brockway KS, Byrnes EJ, Chen L, Chen L, Chen TM, Chin MC, Chong J, Crook BE, Czaplinska A, Dang CN, Datta S, Dee NR, Desaki AL, Desta T, Diep E, Dolbeare TA, Donelan MJ, Dong HW, Dougherty JG, Duncan BJ, Ebbert AJ, Eichele G, Estin LK, Faber C, Facer BA, Fields R, Fischer SR, Fliss TP, Frensley C, Gates SN, Glattfelder KJ, Halverson KR, Hart MR, Hohmann JG, Howell MP, Jeung DP, Johnson RA, Karr PT, Kawal R, Kidney JM, Knapik RH, Kuan CL, Lake JH, Laramee AR, Larsen KD, Lau C, Lemon TA, Liang AJ, Liu Y, Luong LT, Michaels J, Morgan JJ, Morgan RJ, Mortrud MT, Mosqueda NF, Ng LL, Ng R, Orta GJ, Overly CC, Pak TH, Parry SE, Pathak SD, Pearson OC, Puchalski RB, Riley ZL, Rockett HR, Rowland SA, Royall JJ, Ruiz MJ, Sarno NR, Schaffnit K, Shapovalova NV, Sivisay T, Slaughterbeck CR, Smith SC, Smith KA, Smith BI, Sodt AJ, Stewart NN, Stumpf KR, Sunkin SM, Sutram M, Tam A, Teemer CD, Thaller C, Thompson CL, Varnam LR, Visel A, Whitlock RM, Wohnoutka PE, Wolkey CK, Wong VY, Wood M, Yaylaoglu MB, Young RC, Youngstrom BL, Yuan XF, Zhang B, Zwingman TA, Jones AR. 2007. Genome-wide atlas of gene expression in the adult mouse brain. Nature. 445:168–176.
- Liberzon I, Chalmers DT, Mansour A, Lopez JF, Watson SJ, Young EA. 1994. Glucocorticoid regulation of hippocampal oxytocin receptor binding. Brain Res. 650:317–322.
- Lightman SL. 1992. Alterations in hypothalamic–pituitary responsiveness during lactation. Ann N Y Acad Sci. 652:340–346.
- Liu D, Diorio J, Tannenbaum B, Caldji C, Francis D, Freedman A, Sharma S, Pearson D, Plotsky PM, Meaney MJ. 1997. Maternal care, hippocampal glucocorticoid receptors, and hypothalamic–pituitary–adrenal responses to stress. Science. 277:1659–1662.
- Liu L, Li A, Matthews SG. 2001. Maternal glucocorticoid treatment programs HPA regulation in adult offspring: Sex-specific effects. Am J Physiol Endocrinol Metab. 280:E729–E739.
- Lowe GC, Luheshi GN, Williams S. 2008. Maternal infection and fever during late gestation are associated with altered synaptic transmission in the hippocampus of juvenile offspring rats. Am J Physiol Regul Integr Comp Physiol. 295:R1563–R1571.
- McGowan PO, Meaney MJ, Szyf M. 2008. Diet and the epigenetic (re)programming of phenotypic differences in behavior. Brain Res. 1237:12–24.
- McMillen IC, Robinson JS. 2005. Developmental origins of the metabolic syndrome: Prediction, plasticity, and programming. Physiol Rev. 85:571–633.
- Meaney MJ, Diorio J, Francis D, Widdowson J, LaPlante P, Caldji C, Sharma S, Seckl JR, Plotsky PM. 1996. Early environmental regulation of forebrain glucocorticoid receptor gene expression: Implications for adrenocortical responses to stress. Dev Neurosci. 18:49–72.
- Pedersen WA, McCullers D, Culmsee C, Haughey NJ, Herman JP, Mattson MP. 2001. Corticotropin-releasing hormone protects neurons against insults relevant to the pathogenesis of Alzheimer's disease. Neurobiol Dis. 8:492–503.
- Petersson M, Uvnas-Moberg K. 2003. Systemic oxytocin treatment modulates glucocorticoid and mineralocorticoid receptor mRNA in the rat hippocampus. Neurosci Lett. 343:97–100.
- Pettiford JN, Jason J, Nwanyanwu OC, Archibald LK, Kazembe PN, Dobbie H, Jarvis WR. 2002. Age-related differences in cell-specific cytokine production by acutely ill Malawian patients. Clin Exp Immunol. 128:110–117.
- Rajan V, Edwards CR, Seckl JR. 1996. 11 beta-Hydroxysteroid dehydrogenase in cultured hippocampal cells reactivates inert 11-dehydrocorticosterone, potentiating neurotoxicity. J Neurosci. 16:65–70.
- Ruzek MC, Pearce BD, Miller AH, Biron CA. 1999. Endogenous glucocorticoids protect against cytokine-mediated lethality during viral infection. J Immunol. 162:3527–3533.
- Selbach O, Bohla C, Barbara A, Doreulee N, Eriksson KS, Sergeeva OA, Haas HL. 2010. Orexins/hypocretins control bistability of hippocampal long-term synaptic plasticity through co-activation of multiple kinases. Acta Physiol(Oxf). 198 3: 277–285.
- Shanks N, Larocque S, Meaney MJ. 1995. Neonatal endotoxin exposure alters the development of the hypothalamic–pituitary–adrenal axis: Early illness and later responsivity to stress. J Neurosci. 15:376–384.
- Shanks N, Windle RJ, Perks PA, Harbuz MS, Jessop DS, Ingram CD, Lightman SL. 2000. Early-life exposure to endotoxin alters hypothalamic–pituitary–adrenal function and predisposition to inflammation. Proc Natl Acad Sci USA. 97:5645–5650.
- Sharma A, Valadi N, Miller AH, Pearce BD. 2002. Neonatal viral infection decreases neuronal progenitors and impairs adult neurogenesis in the hippocampus. Neurobiol Dis. 11:246–256.
- Shoener JA, Baig R, Page KC. 2006. Prenatal exposure to dexamethasone alters hippocampal drive on hypothalamic–pituitary–adrenal axis activity in adult male rats. Am J Physiol Regul Integr Comp Physiol. 290:R1366–R1373.
- Spencer SJ, Martin S, Mouihate A, Pittman QJ. 2006. Early-life immune challenge: Defining a critical window for effects on adult responses to immune challenge. Neuropsychopharmacology. 31:1910–1918.
- Stephens RS. 2003. The cellular paradigm of chlamydial pathogenesis. Trends Microbiol. 11:44–51.
- Strachan DP. 1989. Hay fever, hygiene, and household size. Br Med J. 299:1259–1260.
- Strachan DP. 2000. Family size, infection and atopy: The first decade of the ‘hygiene hypothesis’. Thorax. 55 Suppl 1: S2–S10.
- Sutanto W, Rosenfeld P, de Kloet ER, Levine S. 1996. Long-term effects of neonatal maternal deprivation and ACTH on hippocampal mineralocorticoid and glucocorticoid receptors. Brain Res Dev Brain Res. 92:156–163.
- Szuran TF, Pliska V, Pokorny J, Welzl H. 2000. Prenatal stress in rats: Effects on plasma corticosterone, hippocampal glucocorticoid receptors, and maze performance. Physiol Behav. 71:353–362.
- Tuchscherer M, Kanitz E, Puppe B, Tuchscherer A, Stabenow B. 2004. Effects of postnatal social isolation on hormonal and immune responses of pigs to an acute endotoxin challenge. Physiol Behav. 82:503–511.
- Vallee M, MacCari S, Dellu F, Simon H, Le Moal M, Mayo W. 1999. Long-term effects of prenatal stress and postnatal handling on age-related glucocorticoid secretion and cognitive performance: A longitudinal study in the rat. Eur J Neurosci. 11:2906–2916.
- Walker FR, Brogan A, Smith R, Hodgson DM. A profile of the immediate endocrine, metabolic and behavioural responses following a dual exposure to endotoxin in early life. Physiol Behav. 2004a; 83:495–504.
- Walker FR, March J, Hodgson DM. Endotoxin exposure in early life alters the development of anxiety-like behaviour in the Fischer 344 rat. Behav Brain Res. 2004b; 154:63–69.
- Walker AK, Nakamura T, Byrne RJ, Naicker S, Tynan RJ, Hunter M, Hodgson DM. 2009. Neonatal lipopolysaccharide and adult stress exposure predisposes rats to anxiety-like behaviour and blunted corticosterone responses: Implications for the double-hit hypothesis. Psychoneuroendocrinology. 34:1515–1525.
- Welberg LA, Seckl JR, Holmes MC. 2001. Prenatal glucocorticoid programming of brain corticosteroid receptors and corticotrophin-releasing hormone: Possible implications for behaviour. Neuroscience. 104:71–79.
- Yamanaka A, Muraki Y, Ichiki K, Tsujino N, Kilduff TS, Goto K, Sakurai T. 2006. Orexin neurons are directly and indirectly regulated by catecholamines in a complex manner. J Neurophysiol. 96:284–298.
- Young E, Korszun A. 2010. Sex, trauma, stress hormones and depression. Mol Psych. 15:23–28.
- Young EA, Haskett RF, Murphy-Weinberg V, Watson SJ, Akil H. 1991. Loss of glucocorticoid fast feedback in depression. Arch Gen Psych. 48:693–699.
- Young EA, Altemus M, Parkison V, Shastry S. 2001. Effects of estrogen antagonists and agonists on the ACTH response to restraint stress in female rats. Neuropsychopharmacology. 25:881–891.
- Zhang Z, Jones S, Hagood JS, Fuentes NL, Fuller GM. 1997. STAT3 acts as a co-activator of glucocorticoid receptor signaling. J Biol Chem. 272:30607–30610.