Abstract
Hepatic lipogenesis-induced de novo by glucocorticoids (GCs) is associated with the development of obesity and diabetes mellitus. The interaction of GCs and insulin in the regulation of hepatic lipogenesis remains unclear. The effect of exogenous GC administration on hepatic lipogenesis and fat deposition was studied in broiler chickens (Gallus gallus domesticus), and the role of insulin in the effect of GCs on hepatic lipogenesis was evaluated. Dexamethasone (DEX, 2 mg/kg body mass (BM)) administration for 3-d resulted in BM loss and increased liver and cervical adipose tissue mass compared to control and pair-fed counterparts. DEX treatment significantly (P < 0.05) increased plasma level of insulin in either the fed or fasting state, whereas plasma glucose level was only increased in the fed state. In fasted chickens, DEX treatment significantly (P < 0.01) upregulated the hepatic mRNA levels of acetyl-CoA carboxylase (ACC) and fatty acid synthase (FAS). In the fed state, the mRNA levels of ACC and FAS were not significantly influenced by DEX treatment, nor was FAS activity. In cultured primary hepatocytes, combined DEX and insulin significantly upregulated the transcription of the genes for FAS (1.34-fold) and malic enzyme (1.72-fold). By contrast, the expression of sterol response element-binding protein-1 (SREBP-1) was significantly upregulated by insulin (1.67-fold) regardless of DEX. In abdominal adipose tissue, DEX treatment had no significant (P>0.05) effect on the activities and transcription of FAS. The expressions of lipoprotein lipase and peroxisome proliferator-activated receptor-γ were not significantly (P>0.05) affected by DEX treatment in either the fasting or fed state. The results indicate that DEX increased hepatic de novo lipogenesis via the increased activity and expression of lipogenic enzymes. Insulin-activated gene expression for SREBP-1 is suggested to be involved in stress-augmented hepatic lipogenesis.
Introduction
Glucocorticoids (GCs), as the final effectors of the hypothalamic–pituitary–adrenal axis, participate in the control of whole body homeostasis and in the arousing of stress responses. Physiological levels of GCs are required for adequate metabolic control, while excessive GC action has been tied to a variety of pandemic metabolic diseases in humans, such as type II diabetes and obesity (Vegiopoulos and Herzig Citation2007).
Cushing's patients with a pronounced elevation of circulating GC levels display central obesity, fatty liver development, and insulin resistance (Shibli-Rahhal et al. Citation2006). In mammals, GCs and insulin play complex roles in the utilization and deposition of energy both independently and interactively (Dallman et al. Citation2005). GCs inhibit while insulin stimulates energy storage and together these hormones mediate long-term energy balance. The upregulated circulating corticosterone together with the altered insulin sensitivity results in enhanced fat deposition (Geraert et al. Citation1996). The development of obesity is attenuated by adrenalectomy and reversed by the administration of GCs (Freedman et al. Citation1986).
Birds have higher plasma glucose concentrations compared to mammals with similar body mass (BM). In contrast, the circulating insulin levels are lower in chickens, and only about one tenth those of rats (Dupont et al. Citation2004). The relative greater resistance to the effect of insulin than that of mammals makes the chicken an interesting model in the study of insulin resistance. De novo lipogenesis in birds, as well as in humans, mainly, if not exclusively, occurs in liver, whereas in rodents both liver and adipose tissues are lipogenic (Bedu et al. Citation2002). Although the insulin cascade appears to be refractory in chicken muscle compared with mammals, the insulin-signaling pathway in the liver of chickens is similar to that in mammals (Dupont et al. Citation2004, Citation2008). In chickens, it was proposed that corticosterone largely impaired insulin signaling in liver, and the expressions of insulin receptor substance and phospho-inositide 3 kinase were all decreased by corticosterone treatment (Dupont et al. Citation1999). Recently, it was shown that GCs enhanced peripheral and abdominal fat deposition in broilers mainly by increasing hepatic de novo lipogenesis (Cai et al. Citation2009). The role of insulin in the regulation of GCs on hepatic de novo lipogenesis remains unclear.
Sterol response element-binding proteins (SREBPs) are a family of membrane-bound transcription factors containing a helix-loop-helix-leucine zipper, and play a unique and fundamental role in both cholesterol and fatty acid metabolism (McPherson and Gauthier Citation2004). In mammals, there are three SREBPs, SREBP-1a, SREBP-1c, and SREBP-2, transcribed from two different genes. SREBP-1 plays a crucial role in the development of fatty liver, and the disruption of SREBP-1 caused a significant reduction in hepatic expression of a battery of lipogenic genes (Yahagi et al. Citation2002). The resultant SREBP-1c protein activates transcription of the genes required for fatty acid synthesis (FAS). Liver X receptors (LXRs), nuclear hormone receptors that form active heterodimers with retinoid X receptors, are known to regulate gene expression of SREBP-1c (Li and Glass Citation2004). LXR null mice are defective in hepatic lipid metabolism (Kalaany et al. Citation2005). The transcription of genes encoding SREBP-1c and LXRs is activated by insulin in liver.
In chickens, SREBP-1 and SREBP-2 genes are located at two separate chromosomes (Assaf et al. Citation2003). Although both of them are expressed in a wide variety of tissues, SREBP-1 is expressed preferentially in the liver and the uropygial gland (Assaf et al. Citation2003). SREBP-1 is an accessory transcription factor that plays an active and central role in the hormonal and nutritional regulation of acetyl-CoA carboxylase α (ACCα) transcription in hepatocytes (Yin et al. Citation2002; Zhang et al. Citation2003). LXRα is involved in the induction of the lipogenic pathway through the activation of SREBP-1 and its target genes in goose primary hepatocytes (Joseph et al. Citation2002; Han et al. Citation2009). Therefore, we hypothesized that LXR and SREBP-1 are associated with the augmented hepatic lipogenesis induced by GCs in broiler chickens with the involvement of insulin.
The objective of the present study was to investigate the effect of GCs on gene expressions of lipogenic enzymes and to evaluate the role of insulin in stress-induced hepatic lipogenesis in broiler chickens (Gallus gallus domesticus). Dexamethasone (DEX), a synthetic GC, was employed to induce the hyper-GC status in the present study (Cai et al. Citation2009). Lipid metabolism in abdominal adipose tissues was investigated as well.
Materials and methods
Animals and diets
Male broiler chicks (Arbor Acres, G. g. domesticus) were obtained from a local hatchery (Taiyu Broiler Co., Taian, Shandong, P. R. China) at 1-d of age and reared in an environmentally controlled room. The brooding temperature was maintained at 35°C (50% RH) for the first 2 d, and then decreased gradually to 21°C (45% RH) until 28 days of age and maintained as such until the end of the experiment. The light regime was 23 h illumination (from 01:00 to 24:00 h) and 1 h dark (from 24:00 to 01:00 h). All chicks were provided ad libitum water and a starter diet (21.5% CP, 3000 kcal malic enzyme (ME)/kg) from day 1 to 21 and a grower diet (19.5% CP, 3100 kcal ME/kg) thereafter. The experiments were conducted in accordance with the “Guidelines for Experimental Animal” of Ministry of Science and Technology, P. R. China and approved by Shandong Agricultural University.
At 35 d of age, 108 birds near the mean BM (1.73 ± 0.02, SEM, kg) were selected and randomly assigned into nine pens of 12 chickens. The nine pens of chickens were randomly subjected to one of the three following treatments, and each treatment was applied to three pens of chickens: subcutaneous injection of DEX (1 mg/ml, dissolved in 0.9% saline) at 08:00 h for 3 d in a dose of 2.0 mg/kg body weight, sham-treated control (injection of saline), and sham-treated and pair-fed treatment (maintaining the same feed consumption of DEX-chickens, pair-fed control). Feed intake and BM gain were recorded daily.
At 38 d of age, 24 chickens were, respectively, selected from each treatment. Eight chickens were, respectively, sampled in a fed or fasting (12-h feed withdrawal) state. A blood sample was obtained with a heparinized syringe within 30 s from a wing vein and collected in iced tubes. Plasma was obtained after centrifugation at 400g for 10 min at 4°C and was stored at − 20°C for further analysis. After bleeding, the chickens were killed by cervical dislocation and then exsanguinated (Cai et al. Citation2009). Tissue samples were obtained from liver and abdominal adipose tissues. The tissue samples were washed with ice-cold sterilized saline, frozen in liquid nitrogen, and stored at − 80°C for further analysis of lipogenic gene transcription. Thereafter, liver, abdominal fat, cervical fat, and thigh fat were harvested, weighed, and expressed as the percentage of BM (%). The remaining eight chickens were bled from a wing vein at 15 min after an injection of heparin (100 U/kg BM) in the fasting state, and the blood was used for the measurement of heparin-releasable lipoprotein lipase (LPL) activity.
Plasma metabolite and insulin analysis
The concentrations of glucose (no. F006), urate (no. C012), non-esterified fatty acids (NEFA, no. A042), triglyceride (TG, no. F001), and LPL (no. A067) were measured spectrophotometrically with colorimetric enzymatic methods by using commercial diagnostic kits (Jiancheng Bioengineering Institute, Nanjing, P. R. China). The concentrations of very low-density lipoprotein (VLDL) were determined as previously described (Griffin and Whitehead Citation1982). Plasma insulin was measured by radioimmunoassay with a guinea pig anti-porcine insulin serum (3V Biochem Engineering Co., Weifang, P. R. China). A large cross-reaction has been observed between chicken insulin and anti-serum (porcine) (Simon et al. Citation1974). The insulin in this study is referred to as immunoreactive insulin. The sensitivity of the assay was 6.9 pmol/l, and all samples were included in the same assay to avoid interassay variability. The intraassay coefficient of variation was 6.9%.
Enzyme activity in hepatic and adipose tissues
The activities of FAS and ME in liver and adipose tissues were measured. The liver and adipose tissue samples were homogenized in ice-cold 0.25 mol/l sucrose, 1 mmol/l dithiothreitol, and 1 mmol/l EDTA at pH 7.4. The cytosolic fractions were obtained by centrifugation at 100,000g for 1 h at 4°C and used for the enzyme assays. The activity of FAS was measured according to the method of Halestrap and Denton (Citation1973). One unit of FAS is defined as 1 nmol reduced nicotinamide adenine dinucleotide phosphate (NADPH) consumed by reacting in 1 min by 1 mg protein. ME activity was determined by a modified method from Hsu and Lardy (Citation1969). One unit of ME is defined as 1 nmol NADPH produced by reacting in 1 min by 1 mg protein.
Hepatocyte culture and in vitro treatments
Primary cultures of chicken hepatocytes were prepared with a modified method described previously by Hahn et al. (Citation1997). In brief, 6-day-old male chickens (Arbor Acres, G. g. domesticus) were killed by decapitation, and livers were immediately removed and placed in Hanks' balanced salt solution without calcium (pH 7.4). After three consecutive washes with fresh Hanks' solution, the liver was minced into small pieces and dissociated by enzymatic treatment with 5 ml 0.1% collagenase II at 37°C for 26 min. During this period, the digested liver was pipetted up and down four times to produce a single-cell suspension. The cell suspension was filtered through a 200-mesh screen, and washed twice with RPMI 1640 by gentle centrifugation (300g, 5 min). Cell viability was estimated by Trypan blue exclusion, and viable hepatocytes at the density of 1 × 105 − 1 × 106 cells/ml were seeded in 6-well plates and cultured in Williams E medium with 10% fetal bovine serum, 0.3 μg/ml DEX, 100 U/ml penicillin, and 100 μg/ml streptomycin. Culture was maintained in the medium at 37°C in a humidified atmosphere containing 5% CO2. After the hepatocytes adhered to the culture plates, and the cell debris and blood cells were removed, the plates were washed with RPMI 1640. The hepatocytes were incubated for 15 h in Williams E medium with 5% fetal bovine serum, followed by serum-free media. Thereafter, the pre-cultured hepatocytes were exposed to one of the four following treatments, the basal serum-free medium (control), or basal medium with added DEX (200 nmol/l) or insulin (100 nmol/l) or both DEX (200 nmol/l) and insulin (100 nmol/l). After 24-h of exposure, total RNA was isolated and transcription of lipogenic genes was measured by real-time PCR.
RNA isolation and analysis
The expression of genes in liver and adipose tissue was measured using quantitative real-time PCR with SYBR Green I labeling.
Total RNA from liver and adipose tissue was isolated using Trizol Reagent (Invitrogen, San Diego, CA, USA). The quality of the RNA was tested by electrophoresis on an agarose-gel, and the quantity of the RNA was determined with a biophotometer (Eppendorf, Germany).
RT reactions (10 μl) consisted of 500 ng total RNA, 5 mmol/l MgCl2, 1 μl RT buffer, 1 mmol/l dNTP, 2.5 U AMV, 0.7 nmol/l oligod (T), and 10 U ribonuclease inhibitor. Real-time PCR analysis was conducted using the Applied Biosystems 7500 Real time PCR System (Applied Biosystems, Foster, CA, USA). Each RT-reaction served as a template in a 20 μl PCR containing 0.2 μmol/l of each primer and SYBR green master mix (Takara, Dalian, Liaoning, P.R. China). Primer-set sequences are described in . Real-time PCRs were performed at 95°C for 10 s, followed by 40 cycles at 95°C for 5 s and 60°C for 34 s. SYBR green fluorescence was detected at the end of each cycle to monitor the amount of PCR product. When calculating the efficiency of qPCR primers, a standard curve was made in 5-fold dilutions, and its slope was used to calculate efficiency.
Table I. Gene-specific primers for the lipid metabolism enzyme of mRNAs.
The relative amount of mRNA was calculated according to the method of Livak and Schmitten (Citation2001). The mRNA levels of these genes were normalized to mRNA for glyceraldehyde 3-phosphate dehydrogenase (GAPDH, △CT). The △CT was calibrated against an average from the control chickens. The linear amount of target molecules relative to the calibrator was calculated by 2− △△CT. Therefore, all gene transcription results are reported as the n-fold difference relative to the calibrator. Specificity of the amplification product was verified by electrophoresis on a 0.8% agarose-gel and by DNA sequencing.
Statistical analysis
Data are presented as means ± SEM. Homogeneity of variances among groups was confirmed using Bartlett's test (SAS Institute). All data were subjected to one-way ANOVA to evaluate the main effect of the DEX treatment. When the main effect of treatment was significant, differences between means were assessed by Duncan's multiple range analysis. P < 0.05 was considered significant.
Results
Chickens treated with DEX significantly (P < 0.0001, ) decreased their BM, while the control and the pair-fed chickens gained BM during the 3-d DEX treatment (). Compared to the control group, DEX-treated chickens consumed less feed (P < 0.01,
). DEX treatment significantly increased liver mass (P < 0.0001,
) and cervical adipose tissue mass (P < 0.05,
). In contrast, the effect of DEX treatment on abdominal and thigh fat masses was not significant (P>0.05).
Table II. Effect of 3-d DEX treatment (DEX, 2 mg/kg BM) on BM gain (n = 3), feed intake (n = 3), and organ index (% of BM, n = 8) of broiler chickens.
DEX treatment significantly increased plasma glucose concentration in fed chickens (P < 0.0001, ), whereas there was no significant effect in the fasting state (). However, plasma concentrations of VLDL (P < 0.01, feeding:
, fasting:
), TG (feeding: P < 0.05,
, fasting: P < 0.01,
), urate (P < 0.001, feeding:
, fasting:
), and insulin (P < 0.05, feeding:
, fasting:
) were all significantly increased by DEX treatment regardless of feeding state, compared to either control or pair-fed chickens. In contrast, DEX treatment had no significant (P>0.05) influence on NEFA concentrations regardless of feeding states. Under the fasting state, heparin-releasable LPL was significantly (P < 0.001,
) increased by DEX treatment compared to either control or pair-fed chickens. There was no significant (P>0.05) difference between control and the pair-fed groups for any of the measured plasma variables.
Table III. Effect of 3-d DEX treatment (DEX, 2 mg/kg BM) on plasma metabolite and insulin concentrations of broiler chickens in fasting and fed states.
In the fasting state, DEX treatment tended to increase hepatic FAS activity (by 89.8%; DEX, 4.08 vs. pair-fed, 2.15 nmol/min/mg protein, P = 0.097, ) and ME activity (by 43.3%; DEX, 36.7 vs. pair-fed, 25.6 nmol/min/mg protein, P = 0.065,
) compared to the pair-fed chickens. In contrast, there was no significant influence in the fed state. The FAS and ME activities in abdominal adipose tissues were not affected by DEX treatment in either fed or fasted chickens (data not shown).
In the fasting state, DEX treatment significantly upregulated the hepatic mRNA levels for ACC (5.35 or 4.86-fold, P < 0.01, ) and FAS (2.48 or 3.26-fold, P < 0.01,
) compared to control or pair-fed chickens, while the ME mRNA level was not significantly influenced (). In contrast, the ME mRNA levels were upregulated (4.76-fold, P < 0.05,
) by DEX treatment in the fed state compared to the pair-fed groups (). No significant (P>0.05) change in the expression of FAS or ACC was detected after DEX in the fed state.
Figure 1. Effects of 3-d DEX treatment (DEX, 2 mg/kg BM) on the hepatic expression of mRNAs for ACC, FAS, and ME in broiler chickens in fasting (A) and fed states (B) Values were determined in duplicate for each sample; values are means ± SEM (n = 8); a–bmeans with different letters differ significantly, P < 0.05, by ANOVA.
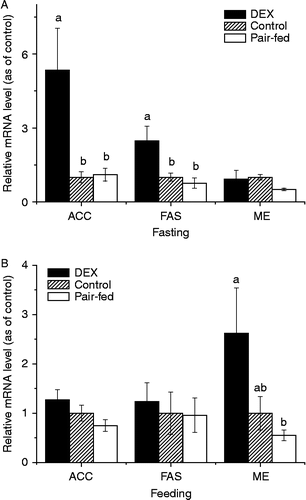
In the abdominal adipose tissue, the expression of mRNAs for FAS, ME, LPL, adipose triglyceride lipase (ATGL) and peroxisome proliferator-activated receptor-γ (PPARγ) was not significantly (P>0.05) affected by DEX treatment at either in the fasting or in the fed state, except that the expression of LPL mRNA was significantly (P < 0.05, ) upregulated by DEX treatment (3.87-fold, data not shown) in fed chickens.
In the in vitro cultured hepatocytes, insulin, DEX, or their combination had no significant influence on the expression of ACC and LXR-α mRNAs (), compared to the control group. In contrast, the administration of DEX together with insulin significantly (P < 0.05) upregulated the expression of mRNAs for FAS (1.34-fold, P = 0.017, ) and ME (1.72-fold, P = 0.0104,
) compared with control treatment, which was not detected (P>0.05) when DEX or insulin was present separately (). The mRNA level for SREBP-1 was significantly upregulated by insulin (1.67-fold, P = 0.0258,
) or insulin with DEX (1.89-fold, P = 0.022,
) treatment compared to the control group.
Figure 2. Effects of DEX and insulin treatment on the expression of mRNAs for ACC, FAS, ME, LXR, and SREBP-1 in hepatocytes cultured in vitro. Values were determined in duplicate for each sample; values are means ± SEM (n = 8); a–bmeans with different letters differ significantly, P < 0.05, by ANOVA.
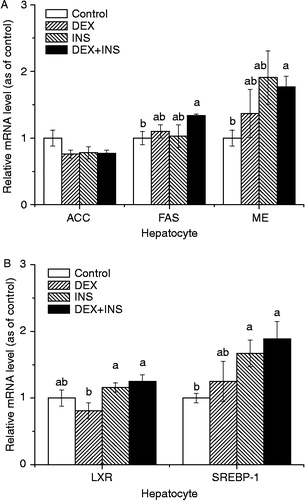
Discussion
The primary objective of the present study was to investigate the effect of GCs on the gene expressions of lipogenic enzymes and to evaluate the role of insulin in stress-induced hepatic lipogenesis of broiler chickens. Exogenous GC administration in broiler chickens and the primary cultured hepatocytes were employed in this study. DEX, a synthetic GC exhibiting a high affinity for GC receptors and a delayed plasma clearance that enhances tissue exposure (Foucaud et al. Citation1998), was employed to induce a hyper-GC status in the present study. The significantly suppressed BM gain and the elevated plasma level of urate in DEX chickens indicated the retarded growth and enhanced proteolysis (), in accordance with the previous reports in chickens challenged with exogenous corticosterone (Lin et al. Citation2004, Citation2006) and in rats subjected to DEX exposure (Dimitriadis et al. Citation1997).
Effect of DEX on hepatic lipogenesis
In birds, liver is the main site of de novo lipogenesis, as in humans. In the present study, both the relative () and the actual liver mass (data not shown) were all significantly higher in DEX-treated chickens compared to control (+47.9 vs. +30.2%) and the pair-fed counterparts (+41.9 vs. +27.0%). The liver hypertrophy induced by DEX treatment was related to the increased TG content (Cai et al. Citation2009).
In the fasting state, there was only a trend for increased FAS activity (+89.8%, P = 0.1), that was consistent with enhanced hepatic lipogenesis. ACC, a rate limiting enzyme in FAS, catalyzes the conversion of acetyl-CoA to malonyl-CoA (M-CoA), while FAS catalyzes the conversion of M-CoA to palmitate. The significantly upregulated expression of mRNAs for ACC and FAS by DEX treatment during fasting compared to the pair-fed chickens (4.86 and 3.26-fold) or control chickens (5.35 and 2.48-fold, ) further indicated the increased de novo hepatic lipogenesis in DEX-treated chickens. As ACC can be regulated at the levels of mRNA expression, protein phosphorylation, and proteasomal degradation, the contribution of post-transcriptional regulation of ACC on hepatic lipogenesis cannot be excluded. In contrast, FAS is regulated primarily at the transcriptional level in avian species (Back et al. Citation1986) and in mammals (Semenkovich Citation1997). The result suggests that the transcription of hepatic lipogenesis genes is upregulated by DEX treatment. As fasting significantly decreased while re-feeding restored ME, ACC and FAS mRNA levels in liver of broiler chickens (Zhang and Hillgartner Citation2004; Proszkowiec-Weglarz et al. Citation2009), the insignificant difference between DEX and control treatments in fed chickens implied that feeding may conceal the stimulating effect of DEX treatment on hepatic lipogenesis.
In the in vivo study, DEX-induced hyperinsulinemia was observed in this study () and in previous work (Lin et al. Citation2006; Yuan et al. Citation2008). The augmented circulating insulin level is suggested to be associated with the enhanced hepatic de novo lipogenesis in DEX-treated chickens (Cai et al. Citation2009). In the in vitro study with primary cultured hepatocytes, the mRNA levels of FAS and ME were upregulated only when DEX and insulin were present simultaneously rather than presented separately (), indicating that the evoking effect of DEX on expression of lipogenic genes was dependent on the presence of insulin. This result was in line with the studies in mammals. Exposure to DEX with insulin enhances insulin action either by overcoming the insulin resistance induced by DEX alone or by stimulating insulin action induced by insulin alone (Amatruda et al. Citation1983). The result indicates that both GC and insulin are necessary for the augmented hepatic de novo lipogenesis in stressed chickens, suggesting that the enhanced fat accumulation during stress is dependent on the effect of GCs in concert with insulin (Dallman et al. Citation2005).
SREBP-1 plays a crucial role in the regulation of lipogenesis in mammals (Yahagi et al. Citation2002) as well as in avian species (Assaf et al. Citation2003). In mammals, LXRs are essential for hepatic lipid metabolism, and SREBP-1c is a direct target of LXR action (Repa et al. Citation2000; Kalaany et al. Citation2005). In accordance with the increased expression of FAS and ME, SREBP-1 mRNA levels were upregulated (1.89-fold, P < 0.05) in primary hepatocytes by DEX plus insulin (), indicating that SREBP-1 is involved in the enhanced expression of hepatic lipogenic genes. Under normal physiological condition, LXRs regulate FAS expression through direct interaction with the FAS promoter as well as through activation of SREBP-1 expression in mice (Joseph et al. Citation2002), chickens (Demeure et al. Citation2009), and goose (Han et al. Citation2009; Wang et al. Citation2009). The result suggests that SREBP-1 is associated with the enhanced gene expression for ACC and FAS by DEX together with insulin.
The marked potentiated gene expression for SREBP-1 was found when insulin was present (), in line with the previous studies in chickens and in mammals. In mammals, gene transcription for SREBP-1c and LXRs is activated by insulin (Liang et al. Citation2002; Tobin et al. Citation2002). In chickens, the transcription of SREBP-1 in liver is activated by insulin, and insulin deprivation decreased the expression of SREBP-1 (Dupont et al. Citation2008). Insulin activates SREBP-1c promoter primarily by increasing the activity of LXRs, possibly through the production of a ligand that activates LXRs or their heterodimerizing partner, the retinoid X receptor (Chen et al. Citation2004). In rats, GCs are involved in the regulation of the expression of LXRα and SREBP-1c (Paterson et al. Citation2004; Erhuma et al. Citation2009). In contrast, with this result, DEX had no significant effect on the gene expression of LXRα and SREBP-1, indicating the different regulation mechanisms in chickens. However, the exact role of DEX in the regulation of these lipogenesis regulators needs to be investigated further.
Collectively, the present results indicate that DEX in concert with insulin facilitates gene expression for hepatic lipogenic enzymes such as FAS and ME. Insulin-activated gene expression for SREBP-1 is suggested to be involved in stress-augmented hepatic lipogenesis. As there was evidence to indicate that corticosterone largely impaired insulin signaling in liver of chickens (Dupont et al. Citation1999), the exact effect of GCs on the signaling pathway of lipogenesis needs to be investigated further.
Effect of DEX on fat deposition in adipose tissues
In line with the previous reports (Lin et al. Citation2004, Citation2006), the increased abdominal (29.5%) and cervical (41.9%) adipose tissue mass was observed in DEX-treated chickens compared to pair-fed control chickens (). The negative BM gain together with the augmented adipose depots indicated the altered energy balance to fat deposition in DEX-treated chickens (Jiang et al. Citation2008; Yuan et al. Citation2008).
The activities of FAS and ME and their mRNA levels in abdominal adipose tissue were not significantly affected by DEX treatment in either the fed or fasting state, suggesting that lipogenesis in adipose tissues was not significantly altered by DEX treatment (Cai et al. Citation2009). The increased abdominal adipose tissue mass in DEX chickens may be related to the enhanced hepatic de novo lipogenesis.
The development of adipose tissue depends on the availability of plasma TGs that are hydrolyzed prior to their uptake by adipocytes (Mossab et al. Citation2002). LPL mRNA is most abundant in adipose tissue. and LPL catalyzes the rate-limiting step in the hydrolysis of TG from circulating chylomicrons and VLDL in adipose tissues (Semenkovich et al. Citation1989; Goldberg Citation1996). In the present study, the mRNA level of LPL was related to the feeding status. LPL mRNA level was significantly upregulated in the fed rather than in the fasting state, in line with the previous studies (Jiang et al. Citation2008; Cai et al. Citation2009). However, the circulating LPL activity was increased in DEX chickens. The significantly increased plasma levels of TG and VLDL in both feeding and fasting states indicate increased blood lipid flux (Yuan et al. Citation2008). Therefore, the results indicate that DEX could improve exohepatic fat accumulation via the augmented lipid flux and upregulated LPL activity and transcription.
PPARγ, a member of the nuclear receptor super-family, triggers the expression of terminal differentiation-related genes and adipogenesis (Krempler et al. Citation2000). On the contrary, ATGL catalyzes the initial step in TG hydrolysis (Zimmermann et al. Citation2004). In line with the previous work (Cai et al. Citation2009), the unaltered mRNA level of PPARγ and ATGL in abdominal adipose tissues implies that lipid metabolism was not significantly altered by DEX treatment.
In conclusion, DEX administration resulted in enhanced fat deposition. DEX treatment increased hepatic de novo lipogenesis via the increased activity and gene expression of lipogenic enzymes (FAS and ME). Insulin-activated gene expression for SREBP-1 is suggested to be involved in stress-augmented hepatic lipogenesis.
Acknowledgements
We thank Ms. Shujing Wang for real-time PCR technical assistance. Financial support was provided by grants from Shandong Science Fund for Distinguished Youth Scholars and National Natural Science Foundation of China (30771573).
Declaration of interest: The authors report no conflicts of interest. The authors alone are responsible for the content and writing of the paper.
References
- Amatruda JM, Danahy SA, Chang CL. 1983. The effects of glucocorticoids on insulin-stimulated lipogenesis in primary cultures of rat hepatocytes. Biochem J. 212:135–141.
- Assaf S, Hazard D, Pitel F, Morisson M, Alizadeh M, Gondret F, Diot C, Vignal A, Douaire M, Lagarrigue S. 2003. Cloning of cDNA encoding the nuclear form of chicken sterol response element binding protein-2 (SREBP-2), chromosomal localization, and tissue expression of chicken SREBP-1 and -2 genes. Poult Sci. 82:54–61.
- Back DW, Goldman MJ, Fisch JE, Ochs RS, Goodridge AG. 1986. The fatty acid synthase gene in avian liver. Two mRNAs are expressed and regulated in parallel by feeding, primarily at the level of transcription. J Biol Chem. 261:4190–4197.
- Bedu E, Chainier F, Sibille B, Meister R, Dallevet G, Garin D, Duchamp C. 2002. Increased lipogenesis in isolated hepatocytes from cold-acclimated ducklings. Am J Physiol Regul Integr Comp Physiol. 283:R1245–R1253.
- Cai YL, Song ZG, Zhang XH, Wang XJ, Jiao HC, Lin H. 2009. Increased de novo lipogenesis in liver contributes to the augmented fat deposition in dexamethasone exposed broiler chickens (Gallus gallus domesticus). Comp Biochem Physiol C. 150:164–169.
- Chen G, Liang G, Ou J, Goldstein JL, Brown MS. 2004. Central role for liver X receptor in insulin-mediated activation of SREBP-1c transcription and stimulation of fatty acid synthesis in liver. Proc Natl Acad Sci USA. 101:11245–11250.
- Dallman MF, Pecoraro NC, Ia Fleur SE. 2005. Chronic stress and comfort foods: Self-mediation and abdominal obesity. Brain Behav Immun. 19:275–280.
- Demeure O, Duby C, Desert C, Assaf S, Hazard D, Guillou H, Lagarrigue S. 2009. Liver X receptor α regulates fatty acid synthase expression in chicken. Poult Sci. 88:2628–2635.
- Dimitriadis G, Leighton B, Parry-Billings M, Sasson S, Young M, Krause U, Bevan S, Piva T, Wegener G. 1997. Effects of glucocorticoid excess on the sensitivity of glucose transport and metabolism to insulin in a rat skeletal muscle. Biochem J. 321:707–712.
- Dupont J, Derouet M, Simon J, Taouis M. 1999. Corticosterone alters insulin signaling in chicken muscle and liver at different steps. J Endocrinol. 162:67–76.
- Dupont J, Dagou C, Derouet M, Simon J, Taouis M. 2004. Early steps of insulin receptor signaling in chicken and rat: Apparent refractoriness in chicken muscle. Domest Anim Endocrinol. 26:127–142.
- Dupont J, Tesseraud S, Derouet M, Collin A, Rideau N, Crochet S, Godet E, Cailleau-Audouin E, Métayer-Coustard S, Duclos MJ, Gespach C, Porter TE, Cogburn LA, Simon J. 2008. Insulin immuno-neutralization in chicken: Effects on insulin signaling and gene expression in liver and muscle. J Endocrinol. 197:531–542.
- Erhuma A, McMullen S, Langley-Evans SC, Bennett AJ. 2009. Feeding pregnant rats a low-protein diet alters the hepatic expression of SREBP-1c in their offspring via a glucocorticoid-related mechanism. Endocrine. 36:333–338.
- Foucaud L, Niot I, Kanda T, Besnard P. 1998. Indirect dexamethasone down-regulation of the liver fatty acid-binding protein expression in rat liver. Biochim Biophys Acta. 1391:204–212.
- Freedman MR, Horwitz BA, Stern JS. 1986. Effect of adrenalectomy and glucocorticoid replacement on development of obesity. Am J Physiol. 250:R595–R607.
- Geraert PA, Padilha JC, Guillaumin S. 1996. Metabolic and endocrine changes induced by chronic heat exposure in broiler chickens: Growth performance, body composition and energy retention. Br J Nutr. 75:195–204.
- Goldberg IJ. 1996. Lipoprotein lipase and lipolysis: Central roles in lipoprotein metabolism and atherogenesis. J Lipid Res. 37:693–707.
- Griffin HD, Whitehead CC. 1982. Plasma lipoprotein concentration as an indicator of fatness in broilers: Development and use of a simple assay for plasma very low density lipoproteins. Br Poult Sci. 23:307–313.
- Hahn M, Gildemeister OS, Krauss GL, Pepe JA, Lambrecht RW, Donohue S, Bonkovsky HL. 1997. Effects of new anticonvulsant medications on porphyrin synthesis in cultured liver cells: Potential implications for patients with acute porphyria. Neurology. 49:97–106.
- Halestrap AP, Denton RM. 1973. Insulin and the regulation of adipose tissue acetyl-coenzyme: A carboxylase. Biochem J. 132:509–517.
- Han CC, Wang JW, Li L, Wang L, Zhang ZX. 2009. The role of LXRa in goose primary hepatocyte lipogenesis. Mol Cell Biochem. 322:37–42.
- Hsu RY, Lardy HA. 1969. Malic enzyme. In: Lowenstein JM. editors. Methods in enzymology. New York: Academic230–235.
- Jiang KJ, Jiao HC, Song ZG, Yuan L, Zhao JP, Lin H. 2008. Corticosterone administration and dietary glucose supplementation enhance fat accumulation in broiler chickens. Br Poult Sci. 49:625–631.
- Joseph SB, Laffitte BA, Patel PH, Watson MA, Matsukuma KE, Walczak R, Collins JL, Osborne TF, Tontonoz P. 2002. Direct and indirect mechanisms for regulation of fatty acid synthase gene expression by liver X receptors. J Biol Chem. 277:11019–11025.
- Kalaany NY, Gauthier KC, Zavacki AM, Mammen PP, Kitazume T, Peterson JA, Horton JD, Garry DJ, Bianco AC, Mangelsdorf DJ. 2005. LXRs regulate the balance between fat storage and oxidation. Cell Metab. 1:231–244.
- Krempler F, Breban D, Oberkofler H, Esterbauer H, Hell E, Paulweber B, Patsch W. 2000. Leptin, peroxisome proliferator-activated receptor-gamma, and CCAAT/enhancer binding protein-alpha mRNA expression in adipose tissue of humans and their relation to cardiovascular risk factors. Arterioscler Thromb Vasc Biol. 20:443–449.
- Li AC, Glass CK. 2004. PPAR- and LXR-dependent pathways controlling lipid metabolism and the development of atherosclerosis. J Lipid Res. 45:2161–2173.
- Liang G, Yang J, Horton JD, Hammer RE, Goldstein JL, Brown MS. 2002. Diminished hepatic response to fasting/refeeding and liver X receptor agonists in mice with selective deficiency of sterol regulatory element-binding protein-1c. J Biol Chem. 277:9520–9528.
- Lin H, Decuypere E, Buyse J. 2004. Oxidative stress induced by corticosterone administration in broiler chickens (Gallus gallus domesticus) 1 domesticus) 1. Chronic exposure. Comp Biochem Physiol B. 139:737–744.
- Lin H, Sui SJ, Jiao HC, Buyse J, Decuypere E. 2006. Impaired development of broiler chickens by stress mimicked by corticosterone exposure. Comp Biochem Physiol A. 143:400–405.
- Livak KJ, Schmittgen TD. 2001. Analysis of relative gene expression data using real-time quantitative PCR and the 2(-Delta Delta C(T)) method. Methods. 25:402–408.
- McPherson R, Gauthier A. 2004. Molecular regulation of SREBP function: The Insig-SCAP connection and isoform-specific modulation of lipid synthesis. Biochem Cell Biol. 82:201–211.
- Mossab A, Lessire M, Guillaumin S, Kouba M, Mourot J, Peiniau P, Hermier D. 2002. Effect of dietary fats on hepatic lipid metabolism in the growing turkey. Comp Biochem Physiol B. 132:473–483.
- Paterson JM, Morton NM, Fievet C, Kenyon CJ, Holmes MC, Staels B, Seckl JR, Mullins JJ. 2004. Metabolic syndrome without obesity: Hepatic overexpression of 11-hydroxysteroid dehydrogenase type 1 in transgenic mice. Proc Natl Acad Sci USA. 101:7088–7093.
- Proszkowiec-Weglarz M, Richards MP, Humphrey BD, Rosebrough RW, McMurtry JP. 2009. AMP-activated protein kinase and carbohydrate response element binding protein: A study of two potential regulatory factors in the hepatic lipogenic program of broiler chickens. Comp Biochem Physiol B. 154:68–79.
- Repa JJ, Liang G, Ou J, Bashmakov Y, Lobaccaro JM, Shimomura I, Shan B, Brown MS, Goldstein JL, Mangelsdorf DJ. 2000. Regulation of mouse sterol regulatory elementbinding protein-1c (SREBP-1c) by oxysterol receptors LXRα and LXRβ. Genes Dev. 14:2819–2830.
- Semenkovich CF. 1997. Regulation of fatty acid synthase (FAS). Prog Lipid Res. 36:43–53.
- Semenkovich CF, Wims M, Noe L, Etienne J, Chan L. 1989. Insulin regulation of lipoprotein lipase activity in 3T3-L1 adipocytes is mediated at posttranscriptional and posttranslational levels. J Biol Chem. 264:9030–9038.
- Shibli-Rahhal A, Van Beek M, Schlechte JA. 2006. Cushing's syndrome. Clin Dermatol. 24:260–265.
- Simon J, Freychet P, Rosselin G. 1974. Chicken insulin: Radioimmunological characterization and enhanced activity in rat fat cells and liver plasma membranes. Endocrinology. 95:1439–1449.
- Tobin KA, Ulven SM, Schuster GU, Steineger HH, Andresen SM, Gustafsson JA, Nebb HI. 2002. Liver X receptors as insulin-mediating factors in fatty acid and cholesterol biosynthesis. J Biol Chem. 277:10691–10697.
- Vegiopoulos A, Herzig S. 2007. Glucocorticoids, metabolism and metabolic diseases. Mol Cell Endocrinol. 275:43–61.
- Wang PH, Ko YH, Chin HJ, Hsu C, Ding ST, Chen CY. 2009. The effect of feed restriction on expression of hepatic lipogenic genes in broiler chickens and the function of SREBP1. Comp Biochem Physiol B. 153:327–331.
- Yahagi N, Shimano H, Hasty AH, Matsuzaka T, Ide T, Yoshikawa T, Amemiya-Kudo M, Tomita S, Okazaki H, Tamura Y, Iizuka Y, Ohashi K, Osuga J, Harada K, Gotoda T, Nagai R, Ishibashi S, Yamada N. 2002. Absence of sterol regulatory element-binding protein-1 (SREBP-1) ameliorates fatty livers but not obesity or insulin resistance in Lep(ob)/Lep(ob) mice. J Biol Chem. 277:19353–19357.
- Yin L, Zhang Y, Hillgartner FB. 2002. Sterol regulatory element-binding protein-1 interacts with the nuclear thyroid hormone receptor to enhance acetyl-CoA carboxylase-α transcription in hepatocytes. J Biol Chem. 277:19554–19565.
- Yuan L, Lin H, Jiang KJ, Jiao HC, Song ZG. 2008. Corticosterone administration and high-energy feed results in enhanced fat accumulation and insulin resistance in broiler chickens. Br Poult Sci. 49:487–495.
- Zhang Y, Hillgartner FB. 2004. Starvation and feeding a high-carbohydrate, low-fat diet regulate the expression sterol regulatory element-binding protein-1 in chickens. J Nutr. 134:2205–2210.
- Zhang Y, Yin L, Hillgartner FB. 2003. SREBP-1 integrates the actions of thyroid hormone, insulin, cAMP, and medium-chain fatty acids on ACCα transcription in hepatocytes. J Lipid Res. 44:356–368.
- Zimmermann R, Strauss JG, Haemmerle G, Schoiswohl G, Birner-Gruenberger R, Riederer M, Lass A, Neuberger G, Eisenhaber F, Hermetter A, Zechner R. 2004. Fat mobilization in adipose tissue is promoted by adipose triglyceride lipase. Science. 306:1383–1386.