Abstract
Negative social experiences such as social stressors and isolation influence mental and physical illnesses, including affective disorders and heart disease. Studies focused on socially monogamous prairie voles can provide insight into neurobiological systems that underlie the consequences of negative social interactions. Female prairie voles were exposed to 28 days of social isolation or pairing with a female sibling (control). Voles were administered daily oxytocin [20 μg/50μl, subcutaneous (sc)] or saline vehicle (50 μl, sc) for 14 days and exposed to two behavioral stressors [elevated plus maze (EPM) and resident–intruder test]. Brain tissue was collected for analysis of central peptide levels in the hypothalamic paraventricular nucleus (PVN). Isolation produced autonomic changes [increased heart rate (HR) and decreased HR variability) during both acute stressors and increased anxiety behaviors in the EPM. Oxytocin injection prevented the autonomic consequences of the acute stressors in isolated prairie voles, but did not affect the behaviors tested under the present conditions. Oxytocin had no effect on the behavioral or autonomic responsiveness in paired prairie voles. Oxytocin injection may exert a beneficial effect on autonomic responses to stressors in isolated animals through increasing the number of oxytocin-containing neurons and decreasing the number of corticotropin-releasing hormone-containing neurons in the PVN. Oxytocinergic mechanisms may serve to compensate for autonomic responses associated with chronic isolation and exposure to both social and non-social acute stressors.
Introduction
Social stressors and the absence of positive social interactions have been associated with several detrimental consequences, including disrupted mood and emotion, increased reactivity of the hypothalamic–pituitary–adrenal (HPA) axis, autonomic imbalance, and central nervous system dysfunction (Anisman and Zacharko Citation1982; Kim and Kirkpatrick Citation1996; Blanchard et al. Citation2001; McCabe et al. Citation2002; Ditzen et al. Citation2007; Grant et al. Citation2009; Norman et al. Citation2010). Previous evidence suggests that negative social experiences may mediate the development and maintenance of affective disorders and cardiovascular diseases (Kalinichev et al. Citation2002; Steptoe et al. Citation2004; Grippo Citation2009; Schwerdtfeger and Friedrich-Mai Citation2009). For instance, in humans, feelings of loneliness are associated with behavioral and cardiovascular alterations including increased ratings of hopelessness, reduced self-esteem, and increased diastolic blood pressure (Steptoe et al. Citation2004). In studies with socially monogamous rodents for which the social structure mirrors that of humans (e.g. prairie vole; Microtus ochrogaster), long-term social isolation produces several negative behavioral and physiological alterations, including depression- and anxiety-relevant behaviors, autonomic and cardiac dysfunction, and increased reactivity to novel social stressors (Grippo et al. Citation2007d; Bosch et al. Citation2009).
The processes that underlie reactions to the social context have been receiving increased attention among researchers (Sgoifo et al. Citation2001; Cacioppo Citation2002; McGraw and Young Citation2009) and the general public (Dingfelder Citation2006; DeAngelis Citation2010); however, our understanding of the neurobiological mechanisms involved in the consequences of negative (or the lack of positive) social experiences is limited. Evidence from several species, including humans and rodents, has led to the hypothesis that neuropeptides and neurohormones including oxytocin, arginine vasopressin (AVP), and corticotropin-releasing hormone (CRH) play an important role in these associations (Taylor et al. Citation2006; Bosch et al. Citation2009; Pierrehumbert et al. Citation2010). For example, high plasma oxytocin levels in humans have been associated with the absence of positive relationships and lower quality social interactions (Taylor et al. Citation2006). Mice that are genetically deficient of oxytocin or its receptor have been shown to be hyper-reactive to psychogenic stressors, compared with control groups (Mantella et al. Citation2004; Takayanagi et al. Citation2005). In prairie voles, several neuroendocrine disruptions have been observed following short- or long-term social stressors, including increased CRH levels in the hypothalamic paraventricular nucleus (PVN), increased circulating corticosterone levels, increased AVP levels in the hypothalamic supraoptic nucleus, and increased PVN and circulating oxytocin levels (Grippo et al. Citation2007b; Ruscio et al. Citation2007).
Studies that focus on the integration of behavioral, cardiovascular, and neural consequences of social stressors using valid animal models promote a greater understanding of these mechanisms. The prairie vole can be a useful rodent model for investigating mechanisms of sociality and the contribution of the social environment to the regulation of emotion, behavior, and cardiovascular function (Carter et al. Citation2008; Grippo Citation2009). Unlike most rodent species, the prairie vole displays an active engagement in the surrounding social context and forms long-term social bonds similar to humans (Carter et al. Citation1995; Getz and Carter Citation1996; Carter and Keverne Citation2002). Prairie voles also exhibit high levels of parasympathetic (vagal) input to the cardiovascular system at rest, similar to humans and larger mammals, but unlike other laboratory rodents, such as rats and mice (Grippo et al. Citation2007c).
Given these distinctive characteristics, the prairie vole has been utilized to investigate hypotheses related to the interactions of social behavior, stress, and cardiovascular function. Previous research from our laboratory and others has demonstrated that prairie voles are highly sensitive to social isolation from family members or opposite-sex partners, displaying several depression-relevant behaviors, altered social behaviors, and physiological consequences including altered heart rate (HR) and HR variability, cardiac arrhythmias, sympathovagal imbalance, hyperactivation of the HPA axis, and altered neuropeptide function (Grippo et al. Citation2007d, Citation2008, Citation2010; Ruscio et al. Citation2007; Bosch et al. Citation2009).
The prairie vole may be a useful model for investigating the role of oxytocin in mediating responses to social isolation. Exogenous oxytocin administration to isolated prairie voles prevented behavioral changes associated with depression as well as several detrimental cardiac consequences including altered HR and HR variability, and autonomic imbalance (Grippo et al. Citation2009). Oxytocin receptors have been identified in cardiac tissue (Jankowski et al. Citation2004), allowing a direct site of action for this peptide to regulate cardiac function. However, oxytocin also may have actions in the central nervous system which influence both behavioral and physiological responses to stress. For instance, the oxytocin molecule or biologically active fragments of the peptide molecule may gain access to the brain via the circumventricular organs or influence central nervous system functions through indirect mechanisms (De Wied et al. Citation1993). To gain a better understanding of the neuropeptide mechanisms that may underlie responses to negative social experiences, this study examined the influence of oxytocin in the regulation of behavioral, physiological, and neural responses to acute stressors in socially isolated prairie voles. Specifically, we investigated the hypotheses that exogenous peripherally administered oxytocin would prevent behavioral and autonomic responses to both a social and a non-social stressor in prairie voles previously exposed to social isolation, and that oxytocin would also influence the levels of neuropeptides in the hypothalamic PVN.
Materials and methods
Animals
The subjects consisted of 26 adult (60–90 days of age) female prairie voles, descendants of a wild stock caught near Champaign, IL, with a mean ± standard error of the mean (SEM) body weight of 44 ± 0.9 g. All voles were maintained on a 14 h/10 h light/dark cycle (lights on at 06:30 h), with a room temperature of 25 ± 1°C and a relative humidity of 21 ± 4 g/m3. Food (Purina rabbit chow) and water were available ad libitum. Offspring were removed from breeding pairs at 21 days of age and housed in same-sex sibling pairs; only one sibling from each pair was used for the experimental procedures described here. All procedures were conducted according to the National Institutes of Health Guide for the Care and Use of Laboratory Animals and approved by the local University Institutional Animal Care and Use Committees.
Females were chosen for these experiments for several reasons. First, female prairie voles may be especially sensitive to the effects of social stressors (Cushing and Carter Citation2000; Grippo et al. Citation2007b). In addition, female prairie voles do not show a spontaneous puberty or estrous cycle; the ovaries remain inactive until the female has physical contact with a male, allowing for the use of reproductively intact animals without the need for artificially controlling the estrous cycle (Carter et al. Citation1987). Finally, female rodents are an understudied group in both behavioral and physiological investigations relating to affective regulation and cardiovascular disorders (Konkle et al. Citation2003).
General experimental design
displays the timeline of procedures employed during this study. Specific experimental procedures are outlined in the following sections.
Table I. Timeline of procedures in this study.
Telemetric transmitter implantation
Wireless radiofrequency transmitters [Data Sciences International (DSI), St. Paul, MN] were implanted for continuous electrocardiogram (ECG) recordings using procedures similar to those described previously (Sgoifo et al. Citation1996). Briefly, transmitters were implanted intraperitoneally under aseptic conditions in anesthetized voles during the light period [ketamine 67 mg/kg, subcutaneous (sc); xylazine 13.33 mg/kg, sc; NLS Animal Health, Owings Mills, MD], and the leads were anchored in place on either side of the heart with permanent sutures. Skin incisions were sutured closed, and sc fluids were administered as necessary.
All animals were housed for 5 days in custom-designed cages (Grippo et al. Citation2007c) which allowed the instrumented vole to interact with its sibling through a divider, but also permitted adequate healing of suture wounds in the instrumented vole. Previous studies (Grippo et al. Citation2007c,Citationd) involving surgical implantation of these transmitters in prairie voles have demonstrated that 5 days of recovery in the divided cage is necessary to facilitate adequate healing of suture wounds, but does not significantly affect the vole's ability to re-establish social bonds following this period. Care was taken to allow voles to interact on a limited basis via small holes in the divider. Following 5 days of recovery in the divided cages, voles were then returned to the standard home cages (with the sibling) for an additional 7–10 days before the onset of experimentation. Voles display signs of social bonding (e.g. sitting in side-by-side contact) within the first few hours after being reunited in the standard home cage.
Social isolation
Following recovery from the surgical procedures, voles were randomly divided into paired (control; n = 13) or isolated (n = 13) conditions. Isolated voles were separated from the sibling and housed individually (without sensory cues from the sibling or other conspecifics) for 4 weeks; paired voles were continually housed with the siblings during this period. Handling, cage changing, and measuring of body weight were matched between the groups.
Oxytocin administration
During weeks 3 and 4 of the isolation/pairing period, voles received one daily sc injection of either oxytocin (20 μg/50 μl/vole; n = 6 paired, n = 6 isolated) or sterile saline vehicle (50 μl/vole; n = 7 paired, n = 7 isolated) for a total of 14 injections. Previous evidence demonstrates that multiple peripheral injections of oxytocin in prairie voles at the dose used here are necessary and sufficient to alter both behavior and autonomic function in a predictable manner. For example, repeated peripheral injections (but not a single injection) of 20 μg oxytocin increase positive social behaviors in adult female prairie voles, including formation of a partner preference (Cushing and Carter Citation2000). Preliminary studies and published results from our laboratory also indicate that a sc dose of 20 μg of oxytocin (but not a lower dose) and a dosing schedule of a once-daily injection during the final 2 weeks of a 4-week isolation period (but not a single injection) prevent long-term behavioral and autonomic consequences of social isolation in isolated female prairie voles, including depressive behaviors, increased HR, decreased HR variability, and sympathovagal imbalance (but do not alter the behavior or autonomic function of paired prairie voles; Grippo et al. Citation2009). Injections were administered during the light period between 09:00 and 11:00 h in a counterbalanced fashion. Immediately following each injection, voles were replaced in the home cages and left undisturbed for approximately 24 h, until the next scheduled injection time or until the beginning of behavioral tests (described below).
Elevated plus maze
Forty-eight hours following the end of the 4-week isolation period and the final oxytocin injection, anxiogenic behaviors were measured in the elevated plus maze (EPM) during the light period, using procedures described elsewhere (Pellow et al. Citation1985). The maze (57 cm height) consisted of two open arms of clear Plexiglas opposite to each other (49.5 × 10 cm), two closed arms of black Plexiglas with an open roof (49.5 × 10 × 30.5 cm), and a center section of clear Plexiglas (10 × 10 cm). Telemetry receivers were placed under the center and end of each arm, approximately 15 cm below the maze, to allow for a continuous ECG and activity signal for each vole during the entire test. The vole was placed in the center section and allowed to explore freely for 5 min. Voles were returned to the home cage immediately following the test. ECG and generalized activity parameters were recorded continuously before, during, and following the test. Behaviors were video-recorded during the test, and were subsequently scored by two trained observers blinded to the experiment. Voles were coded as entering a section of the maze when all four paws crossed into the respective section.
Resident–intruder test
Forty-eight hours after the completion of the EPM test (96 h following the end of the 4-week isolation period and the final oxytocin injection), a resident–intruder (RI) test was conducted during the light period, according to procedures described previously (Bosch et al. Citation2004; Grippo et al. Citation2007a). The paired or isolated vole (intruder) was placed into the cage of an unrelated and unfamiliar vole of the same sex, age, and approximate size (resident) for 5 min. ECG and generalized activity parameters were recorded continuously before, during, and following the test. Each vole was returned to its home cage immediately following the test. Aggressive behaviors of each resident and intruder (aggressive grooming or posture, swatting, biting, thrusting, pulling, and/or attack behavior; Mitchell et al. Citation2003) were video-recorded and subsequently scored by two trained observers blinded to the experiment.
Quantification of radiotelemetric recordings
Quantification of telemetric variables
ECG and activity signals were recorded with a radiotelemetry receiver (DSI; sampling rate 5 kHz for ECG and 256 Hz for activity, 12-bit precision digitizing). These parameters were recorded continuously during all experimental procedures, and were processed and quantified using the vendor software (Dataquest ART Acquisition software version 4.1), according to the methods described previously (Grippo et al. Citation2007c). Briefly, multiple segments of 1–5 min of stable, continuous data were used to evaluate HR, HR variability, and activity before, during, and following the behavioral stressors (48–96 h following the end of the isolation period and the final oxytocin injection). All R–R intervals were manually inspected using custom-designed software (Brain-Body Center, University of Illinois at Chicago, Chicago, IL; Porges Citation1985; Porges and Bohrer Citation1990), and care was taken to exclude the segments of ECG which were confounded by animal movement artifact. HR was evaluated by quantifying the number of beats per unit time (beats per minute, bpm).
The R–R intervals were analyzed for variations to provide two measures of HR variability, including SD of all R–R (normal-to-normal; N–N) intervals (SDNN Index; Task Force of the European Society of Cardiology and North American Society of Pacing and Electrophysiology Citation1996) and amplitude of respiratory sinus arrhythmia (RSA; Yongue et al. Citation1982; Grippo et al. Citation2007c). The SDNN Index was evaluated according to the procedures described previously (Task Force of the European Society of Cardiology and North American Society of Pacing and Electrophysiology Citation1996), and is hypothesized to represent the convergence of both sympathetic and parasympathetic innervation to the sino-atrial node.
RSA was assessed with time-frequency procedures (Porges Citation1985; Porges and Bohrer Citation1990) that have been validated with humans (CitationPorges 2007), applied to small mammals (Yongue et al. Citation1982), and modified for the prairie vole (Grippo et al. Citation2007c). The RSA amplitude represents the functional vagal impact on the sino-atrial node of myelinated vagal efferent pathways originating in the nucleus ambiguus (CitationPorges 2007). To deal with the possibility that violating the assumption of stationarity can distort time series analyses of RSA, the following procedures were implemented: (a) the R–R intervals (heart period) were time sampled into equal time intervals with a sampling rate of 20 Hz and (b) the time series were detrended with a moving polynomial filter (Porges and Byrne Citation1992) that removed variance in the series below 1.0 Hz for RSA (i.e. 21-point cubic polynomial). The residuals of these procedures were free of aperiodic and slow periodic processes that may violate the assumption of stationarity. A bandpass filter was applied to define RSA by extracting only the variance in the HR spectrum between the frequencies of 1.0 and 4.0 Hz, which has been confirmed through spectral analyses to be the frequency band in which breathing is observed in mammals of size similar to prairie voles (Ishii et al. Citation1996; Gehrmann et al. Citation2000; Grippo et al. Citation2007c).
Behavioral tests
Cardiac and activity parameters were evaluated using the continuous data recorded during the EPM and RI test, using the segments of data that were not confounded by movement artifact. Pre-stressor cardiac parameters were derived from ECG data sampled during a period of at least 1 h of minimal activity [5 counts per minute (cpm) or lower], recorded approximately 2–4 h before the behavioral stressors. Post-stressor parameters were derived from ECG and activity sampled during a period of at least 15 min of minimal activity (5 cpm or lower), recorded approximately 3 h after each behavioral stressor.
Collection of tissue and immunohistochemical analysis
Forty-eight hours following the final behavioral test (144 h following the end of the 4-week isolation period and the final oxytocin injection), all voles were anesthetized with a mixture of ketamine (67 mg/kg, sc) and xylazine (13.33 mg/kg, sc) during the light period. Voles were anesthetized within 1 min of being removed from the housing room, and euthanized via cervical dislocation. Brains were carefully removed and processed with a passive perfusion technique described previously (Cushing et al. Citation2001; Grippo et al. Citation2007a). Briefly, brains were immersed in a fixative solution consisting of 4% paraformaldehyde containing 5% acrolein (pH 8.6) for a total of 4 h. Brains were postfixed for 24 h in 4% paraformaldehyde, and sunk in 25% sucrose. Tissue was stored in 25% sucrose at 4°C until it was sectioned at 40 μm on a freezing sliding microtome. Sliced serial brain sections were stored in wells at − 20°C, in cryoprotectant antifreeze solution, until analyzed for peptide immunoreactivity in the PVN.
Serial brain slices (40 μm) from the PVN were assayed for oxytocin, AVP, and CRH using standard avidin:biotinylated enzyme complex immunocytochemistry, according to the procedures described previously (Grippo et al. Citation2007b). Anti-CRH (generously provided by Dr Ann-Judith Silverman) was used at a concentration of 1:50,000; anti-oxytocin (generously provided by Dr Mariana Morris) was used at a concentration of 1:300,000; and anti-AVP (MP Biomedicals, Solon, OH) was used at a concentration of 1:200,000. All antibodies were generated in rabbit.
Stained sections were mounted on electrostatically charged slides, air-dried, dehydrated in a series of ethanol dilutions, cleared with Histoclear (National Diagnostics, Atlanta, GA), and then protected with coverslips using Histomount mounting medium (National Diagnostics, Atlanta, GA).
Images were captured using an Olympus BH-2 microscope (Lombard, IL) equipped with a Pixera Penguin 600CL camera and Pixera Studio 3.0 imaging software (San Jose, CA). The density of oxytocin-, AVP-, and CRH-immunoreactive cell bodies was determined visually in the PVN using a 20X objective according to procedures described previously (Grippo et al. Citation2007b; Ruscio et al. Citation2007). Briefly, measurements within the PVN were taken from a section of the nucleus approximate to that shown in Figure 49 of Paxinos and Watson (Citation2005), in which the stained cell bodies take a characteristic shape demonstrated in previous studies (Wang et al. Citation1996; Ruscio et al. Citation2007). A standardized sampling area was defined in sections that were matched in rostral–caudal orientation to minimize variability. The total number of immunoreactive cell bodies was counted to define a measurement of cell body density within the standardized sampling area. Two to three sections were analyzed from each subject, and results for each vole were averaged across sections. Counts were performed separately for each hemisphere, and the results were averaged between the hemispheres. For all subjects, measurements of immunoreactive cell body density were conducted by two trained raters blinded to treatment, and the results were averaged between the raters. Density measures were therefore averaged across multiple hemispheres, brain slices, and raters to provide an estimate of immunoreactive cell body density each for oxytocin, AVP, and CRH in the PVN.
Data analyses
The data are presented as means ± (or+)SEM for all analyses, tables, and figures. A probability value of P < 0.05 was considered to be statistically significant. As described in the following sections: (a) for the behavioral measures, data from voles that did not complete the full behavioral tests were excluded from the analyses; (b) for autonomic measures, periods of ECG involving vole movement artifact were excluded from the analyses; and (c) for central nervous system measures, damaged brain sections were excluded from the analyses.
For repeated measures, data were analyzed with three-factor mixed-design analyses of variance (ANOVA), with group (paired or isolated) and exogenous treatment (oxytocin or vehicle) as independent variables and the following as repeated dependent variables: (a) HR; (b) SDNN Index; and (c) RSA amplitude before, during, and following the behavioral stressors. Single measures, including (a) behaviors during the stressors; (b) density of PVN oxytocin-immunoreactive cell bodies; (c) density of PVN AVP-immunoreactive cell bodies; and (d) density of PVN CRH-immunoreactive cell bodies, were analyzed using two-way independent groups ANOVA, with housing group (paired or isolated) and treatment (oxytocin or vehicle) as independent variables. Multiple comparisons were conducted using Fisher's Protected Least Significant Difference post-hoc analyses or Student's t-test with a Bonferroni correction (hypothesis-driven, statistically justified comparisons).
For ease of interpreting the results, the following four group names are used herein: Paired+oxytocin (OT), Paired+vehicle (V), Isolated+OT, and Isolated+V. Results are presented first with a general description of each major finding, followed by a specific description of the statistical test results and associated probability values.
Results
Responses to the EPM
Autonomic data associated with the EPM were excluded for two voles that did not have complete pre-EPM, EPM, and post-EPM ECG recordings. All data (behavioral and ECG) were excluded for two additional voles that fell off the maze (these tests were immediately aborted). Isolation was associated with anxiogenic behavior during the EPM, with no corresponding change in generalized activity level. However, the anxiogenic behavior was not prevented with oxytocin administration in the isolated group. Isolation was also associated with increases in HR and reductions in SDNN Index (but not RSA) during the EPM; these cardiac alterations were prevented with oxytocin administration in the isolated group. Oxytocin administration had no significant effect on the behavioral or autonomic responses of paired voles before, during, or following exposure to the EPM.
Behavioral data during the EPM test are shown in . The ANOVA for open arm time yielded a main effect of group [F(1,20) = 13.48, P < 0.002], but did not yield an interaction. A t-test confirmed that, irrespective of peptide administration, isolated voles spent significantly less time in the open arms of the EPM than the paired voles [t(22) = 3.86, P < 0.0004]. Also, although 92% of the paired voles entered the open arm at least once during the 5-min test, only 50% of the isolated voles entered the open arm (z = 2.26, P < 0.05).
Table II. Behavioral responses in the open and closed arms of the 5-min EPM test in prairie voles following 4 weeks of social isolation or pairing plus daily exogenous administration of either oxytocin (20 μg/50 μl/vole, sc) or sterile saline vehicle (50 μl/vole, sc).
The ANOVA for closed arm time yielded a housing group × treatment interaction [F(1,20) = 26.05, P < 0.0001] and main effects of both group [F(1,20) = 31.72, P < 0.0001] and treatment [F(1,20) = 4.50, P < 0.05]. A t-test indicated that both isolated groups spent significantly more time in the closed arms of the EPM than both the paired groups [t(22) = 1.91, P < 0.03]; however, this behavior was not affected by oxytocin administration (P>0.05). The ANOVA for center section duration yielded a main effect of treatment [F(1,20) = 4.96, P < 0.04], but did not yield an interaction. Follow-up tests yielded no significance between-group differences in the time spent in the center section (P>0.05 for all comparisons; data not shown).
The ANOVA for general activity level during the EPM yielded no significant effects (P>0.05 for both main effects and interaction).
Autonomic data during the EPM test are shown in . The ANOVA for HR yielded a main effect of housing group [F(1,18) = 7.22, P < 0.02] and a main effect of treatment [F(1,18) = 11.94, P < 0.003]. Post-hoc analyses, with P < 0.05 defined as the critical probability value, indicated that the Isolated+V group showed a significantly higher HR than the other three groups before exposure to the EPM (P < 0.05 for all comparisons). In addition, all four groups showed a significantly higher HR during the EPM test versus the pre- and post-EPM time points (P < 0.05 for all comparisons). The Isolated+V group displayed a significantly higher HR than the other three groups following the EPM test (P < 0.05 for all comparisons).
Figure 1. Mean ( + SEM) HR (Panel A), SDNN Index (Panel B), and amplitude of RSA (Panel C) before, during, and following the 5-min EPM test in prairie voles following 4 weeks of social isolation or pairing plus daily exogenous administration of either oxytocin (20 μg/50 μl/vole, sc) or sterile saline vehicle (50 μl/vole, sc), in the following groups: Paired+V (‐‐▴‐‐; n = 6), Paired+OT (—▴—; n = 5), Isolated+V (‐‐▪‐‐; n = 6), and Isolated+OT (—▪—; n = 5). Three-factor mixed-design ANOVA with post-hoc multiple comparisons: *P < 0.05 versus Paired+V, Paired+OT, and Isolated+OT groups at the same time point; †P < 0.05 versus two paired groups at the same time point; ‡P < 0.05 versus respective EPM value. Abbreviations: ANOVA, analyses of variance; EPM, elevated plus maze; HR, heart rate; OT, oxytocin; RSA, respiratory sinus arrhythmia; SDNN, SD of normal-to-normal intervals; SEM, standard error of the mean; V, vehicle.
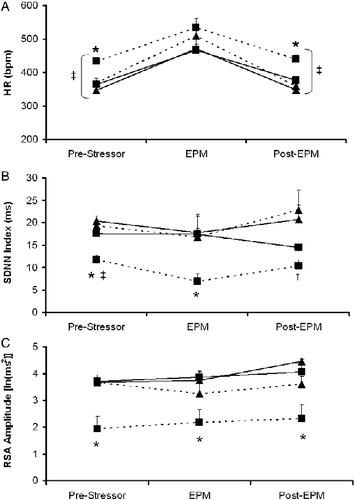
The ANOVA for SDNN Index yielded a group × treatment interaction [F(1,18) = 5.65, P < 0.03], a main effect of group [F(1,18) = 21.27, P < 0.0002], and a main effect of treatment [F(1,18) = 5.44, P < 0.03]. Post-hoc analyses, with P < 0.05 defined as the critical probability value, indicated that the Isolated+V group displayed a significantly lower pre-EPM SDNN Index value than both paired groups and the Isolated+OT group (P < 0.05 for all comparisons). The Isolated+V group displayed a significantly lower SDNN Index during the EPM than this group's respective pre-EPM value (P < 0.05), and the SDNN Index during the EPM in this group was significantly lower than the other three groups (P < 0.05 for all comparisons). There was no change in SDNN Index during the EPM test, compared with pre- or post-EPM time points, in the Paired+V, Paired+OT, or Isolated+OT groups (P>0.05 for all comparisons). Following the EPM test, the Isolated+V group's SDNN Index value did not differ significantly from its EPM SDNN Index value (P>0.05), and it was significantly lower than the SDNN Indices of both paired groups at the same time point (P < 0.05 for both comparisons).
The ANOVA for RSA amplitude yielded a housing group × treatment interaction [F(1,18) = 6.88, P < 0.02], a main effect of group [F(1,18) = 20.39, P < 0.0003], and a main effect of treatment [F(1,18) = 8.75, P < 0.009]. Post-hoc analyses, with P < 0.05 defined as the critical probability value, indicated that the Isolated+V group displayed significantly lower pre-EPM, EPM, and post-EPM RSA amplitudes than the other three groups at the same time points (P < 0.05 for all comparisons). No significant differences in RSA amplitude were observed among the Paired+V, Paired+OT, or Isolated+OT groups at any time point (P>0.05 for all comparisons). None of the groups displayed a change in RSA amplitude during the EPM versus pre- and post-EPM time points (P>0.05 for all comparisons).
Responses to the RI test
Autonomic data during the RI test were excluded for three voles that did not have complete pre-RI test, RI test, and post-RI test ECG recordings. Neither isolation nor oxytocin administration significantly affected the behavior during the RI test. However, isolation was associated with altered cardiac responses during and following the RI test, and these responses were partially (but not completely) prevented by oxytocin administration. Oxytocin administration had no significant effects on the behavioral or autonomic responses of paired voles before, during, or following the RI test.
Behavioral data during the RI test are shown in . The ANOVA for aggressive behaviors during the RI test yielded no significant effects (P>0.05 for both main effects and interaction). Similarly, the ANOVA for general activity level did not yield any significant effects (P>0.05 for both main effects and interaction).
Table III. Behavioral responses in the 5-min RI paradigm in prairie voles following 4 weeks of social isolation or pairing plus daily exogenous administration of either oxytocin (20 μg/50 μl/vole, sc) or sterile saline vehicle (50 μl/vole, sc).
Autonomic data during the RI test are shown in . The ANOVA for HR yielded a housing group × treatment interaction [F(1,19) = 8.71, P < 0.008], a main effect of group [F(1,19) = 33.14, P < 0.0001], and a main effect of treatment [F(1,19) = 7.70, P < 0.01]. Post-hoc analyses, with P < 0.05 defined as the critical probability value, indicated that the Isolated+V group showed a significantly higher HR than the other three groups before and during the RI test (P < 0.05 for all comparisons). The HRs of the other three groups were not significantly different before or during the RI test (P>0.05 for all comparisons). In addition, all the four groups showed a significantly higher HR during the RI test versus the pre- and post-RI test time points (P < 0.05 for all comparisons). Both the isolated groups displayed higher HR versus both paired groups following the RI test (P < 0.05 for all comparisons).
Figure 2. Mean ( + SEM) HR (Panel A), SDNN Index (Panel B), and amplitude of RSA (Panel C) before, during, and following the 5-min resident–intruder paradigm in prairie voles following 4 weeks of social isolation or pairing plus daily exogenous administration of either oxytocin (20 μg/50 μl/vole, sc) or sterile saline vehicle (50 μl/vole, sc), in the following groups: Paired+V (‐‐▴‐‐; n = 6), Paired+OT (—▴—; n = 5), Isolated+V (‐‐▪‐‐; n = 7), and Isolated+OT (—▪—; n = 5). Three-factor mixed-design ANOVA with post-hoc multiple comparisons: *P < 0.05 versus Paired+V, Paired+OT, and Isolated+OT groups at the same time point; †P < 0.05 versus two paired groups at the same time point; ¶P < 0.05 versus Paired+V and Isolated+OT groups at the same time point; ‡P < 0.05 versus respective RI test value. Abbreviations: ANOVA, analyses of variance; HR, heart rate; OT, oxytocin; RI test, resident–intruder paradigm; RSA, respiratory sinus arrhythmia; SDNN, SD of normal-to-normal intervals; V, vehicle.
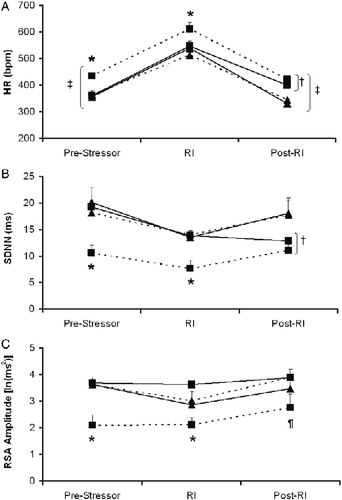
The ANOVA for SDNN Index yielded a group × treatment interaction [F(1,19) = 7.79, P < 0.01], a main effect of group [F(1,19) = 11.10, P < 0.004], and a main effect of treatment [F(1,19) = 4.57, P < 0.05]. Post-hoc analyses, with P < 0.05 defined as the critical probability value, indicated that the Isolated+V group showed a significantly lower SDNN Index than the other three groups before and during the RI test (P < 0.05 for all comparisons). The SDNN Indices of the other three groups were not significantly different before or during the RI test (P>0.05 for all comparisons). Following the RI test, both isolated groups displayed significantly lower SDNN Indices than both the paired groups (P < 0.05 for all comparisons).
The ANOVA for RSA amplitude yielded a housing group × treatment interaction [F(1,19) = 12.97, P < 0.002], a main effect of group [F(1,19) = 8.05, P < 0.01], and a main effect of treatment [F(1,19) = 15.89, P < 0.0008]. Post-hoc analyses, with P < 0.05 defined as the critical probability value, indicated that the Isolated+V group showed a significantly lower RSA amplitude than the other three groups before and during the RI test (P < 0.05 for all comparisons), and a significantly lower RSA amplitude versus the Isolated+OT and Paired+V groups following the RI test (P < 0.05 for both comparisons). There were no significant differences in RSA among the Paired+V, Paired+OT, or Isolated+OT groups at any time point (P>0.05 for all comparisons).
PVN peptide responses
Isolation alone did not alter the density of PVN oxytocin- or AVP-immunoreactive cell bodies, but it significantly increased the density of CRH-immunoreactive cell bodies. Administration of oxytocin led to an increase in density of PVN oxytocin-immunoreactive cell bodies, versus administration of vehicle, in both the paired and isolated groups. Oxytocin administration led to a decrease in the density of CRH-immunoreactive cell bodies in the isolated group, with no detectable difference in CRH in the paired group, for which CRH immunoreactivity was already low. Administration of oxytocin did not affect AVP immunoreactivity in the PVN in either paired or isolated groups.
Central nervous system data for statistically significant effects are shown in . The ANOVA for density of oxytocin-immunoreactive cell bodies yielded a trend toward a housing group × treatment interaction [F(1,22) = 4.28, P = 0.05], and a main effect of treatment [F(1,22) = 26.97, P < 0.05]. T-tests with a Bonferroni correction, with a probability value of P < 0.0125 required, confirmed that both oxytocin-treated groups displayed higher PVN oxytocin cell body levels than both the paired groups [Paired+OT versus Paired+V: t(11) = 4.31, P < 0.0006; Isolated+OT versus Isolated+V: t(11) = 2.88, P < 0.007].
Figure 3. Coronal brain sections (40 μm) from a representative subject in each group showing the density of immunoreactive cell bodies (number of cell bodies per standardized area; oxytocin, Panel A; CRH, Panel B), and mean ( + SEM) group data (oxytocin, Panel C; CRH, Panel D), in the PVN of the hypothalamus of paired and isolated prairie voles following 4 weeks of social isolation or pairing plus daily exogenous administration of either oxytocin (20 μg/50 μl/vole, sc) or sterile saline vehicle (50 μl/vole, sc), in the following groups: Paired+V (n = 7), Paired+OT (n = 6), Isolated+V (n = 7), and Isolated+OT (n = 6). Scale bars = 100 μm. Two-way independent-groups ANOVA with Bonferroni-corrected t-tests for multiple comparisons: *P < 0.05 versus Paired (Pair) + V, Paired+OT, and Isolated (Isol) + OT groups; †P < 0.05 versus two vehicle-treated groups. Abbreviations: ANOVA, analyses of variance; CRH, corticotropin-releasing hormone; ir, immunoreactive; OT, oxytocin; V, vehicle.
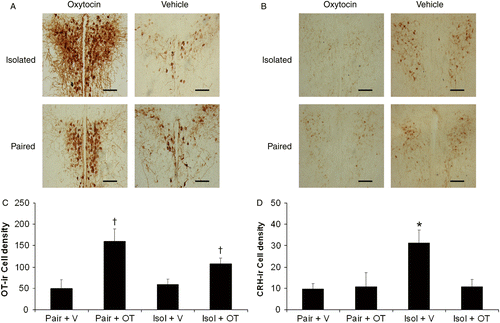
The ANOVA for density of CRH-immunoreactive cell bodies yielded a housing group × treatment interaction [F(1,22) = 5.71, P < 0.03], and main effects of group [F(1,22) = 5.78, P < 0.03] and treatment [F(1,22) = 4.79, P < 0.04]. T-test with a Bonferroni correction, with a probability value of P < 0.01 required, indicated that the Isolated+V group had higher PVN CRH levels than the Isolated+OT [t(11) = 2.84, P < 0.008], Paired+OT [t(11) = 2.45, P < 0.01], and Paired+V groups [t(12) = 3.35, P < 0.003]. No significant differences were found in PVN CRH levels among the Isolated+OT, Paired+OT, or Paired+V groups (P>0.05 for all comparisons).
The ANOVA for density of AVP-immunnoreactive cell bodies did not yield any significant main effects or interactions (P>0.05; data not shown).
Discussion
This study was designed to examine the hypothesis that in female prairie voles, peripheral oxytocin administration would modulate behavioral and autonomic responses to acute stressors, and that these effects would vary as a function of the social conditions in which these voles were living. We have demonstrated that chronic isolation in prairie voles serves as a chronic stressor, similar to previous studies (Grippo et al. Citation2007d, Citation2011). These findings also support the hypothesis that exogenous oxytocin can be protective and is capable of reducing some measures of autonomic reactivity to both social and non-social acute stressors. However, oxytocin treatment did not have a detectable beneficial effect on the behavioral responsiveness to acute stressors in the context of social isolation at the dose and route of administration employed here. Furthermore, the present data indicate that exogenous oxytocin may interact with oxytocin- and CRH-producing cells in the PVN.
The present results indicate that there may be a dissociation between the behavioral and autonomic responses during the EPM in voles that were pre-treated with oxytocin. The autonomic responses of isolated prairie voles differed from those of paired prairie voles before and during this stressor; and also did not recover at the same rate following this stressor. The autonomic changes associated with exposure to the EPM were reduced in isolated voles that received oxytocin, as shown by cardiac rate and rhythm responses comparable to the paired groups before, during, and following this stressor.
Similar to previous findings (Grippo et al. Citation2007d), prairie voles exposed to social isolation displayed an anxiogenic response during the EPM test relative to paired voles. However, contrary to our initial predictions, oxytocin administration did not prevent anxiogenic behaviors during the EPM test at the dose and route of administration employed here. This observation differs from some previous human (Heinrichs et al. Citation2003) and rodent studies (Uvnäs-Moberg et al. Citation1994; Windle et al. Citation1997, Citation2006; Slattery and Neumann Citation2010), which suggests that oxytocin has anxiolytic properties. The experimental protocol employed here differs from that employed in previous studies on several key variables. First, given that oxytocin was administered peripherally in this study, it is possible that the lack of behavioral anxiolytic effects was due to its inability to access the central nervous system. Second, oxytocin treatments were terminated 48–96 h before behavioral testing, which may have reduced the capacity of the treatment to influence behaviors, although autonomic and neuroanatomical effects of oxytocin were still detected. Third, the effects of oxytocin in the current study may have been partially dependent on the species, reproductive state of the voles, and basal hormone levels. These findings represent the behaviors of reproductively naïve (virgin) female voles, which differ from some previous studies that have reported anxiolytic effects of oxytocin in men (Heinrichs et al. Citation2003), male rats (Uvnäs-Moberg et al. Citation1994), or female ovariectomied rats supplemented with either 17β-estradiol benzoate (Windle et al. Citation1997) or with a combination of estradiol and progesterone to mimic the hormonal profile during late pregnancy (Windle et al. Citation2006). The current results may be supported by findings from Neumann et al. (Citation2000), who showed an anxiolytic effect of oxytocin in the EPM in pregnant and lactating female rats, but not in virgin rats.
However, in contrast to the present results are those from Slattery and Neumann (Citation2010), showing that chronic intracerebroventricular infusion of oxytocin attenuated anxiogenic behaviors in Wistar female rats selectively bred for high anxiety-related behavior. Given the differences in species, strain, sex, and reproductive status of the subjects (Slattery and Neumann Citation2010), as well as the doses and routes of administration of oxytocin, further investigation of the precise consequences of peripherally versus centrally administered oxytocin in both humans and animal models will be necessary to determine the mechanism(s) of its beneficial effects on anxiogenic behaviors and autonomic responses to acute environmental stressors.
During the RI test, neither isolation nor oxytocin administration altered behavior, including aggressive behavior and generalized activity level. The lack of behavioral changes during this test is consistent with previous studies showing that neither 4 nor 8 weeks of social isolation was associated with altered aggression during this stressor in female prairie voles, despite significant neuroendocrine activation in response to the stressor (versus paired control conditions; Grippo et al. Citation2007a,Citationb). The autonomic profile observed here in isolated voles mirrors the neuroendocrine profile demonstrated previously (Grippo et al. Citation2007a,Citationb). At all three time points (before, during, and following the RI test), HR was highest in the Isolated+V group, whereas SDNN Index and RSA amplitude were lowest in this group. Oxytocin partially prevented these autonomic reactions in isolated voles (). Pre-treatment with oxytocin in isolated prairie voles may be associated with a different time course of recovery of autonomic parameters following various stressors, possibly dependent on differential sympathetic and parasympathetic regulation of the heart (Norman et al. Citation2011).
In addition to preventing autonomic responses to the EPM and RI test, oxytocin administration was also associated with changes in the PVN of the hypothalamus. Administration of this peptide was associated with an increase in the density of oxytocin-immunoreactive neurons in the PVN, which may indicate increased synthesis of endogenous oxytocin. Alternatively, this cytochemical change could be the result of decreased release of the peptide. Increases in the density of oxytocin-immunoreactive cell bodies were evident in both paired and isolated voles, indicating that circulating oxytocin may regulate central processes via a feed-forward mechanism, as has been suggested previously (Falke Citation1989; CitationYee et al. 2010). Alterations of PVN levels of oxytocin-containing cell bodies may affect a variety of central target tissues including brainstem regions that regulate the autonomic nervous system, as well as hypothalamic–amygdala targets that may be of particular importance for anxiogenic behaviors.
It is notable that, although oxytocin administration was associated with increased density of oxytocin-immunoreactive cell bodies in the PVN in paired prairie voles, administration of the exogenous peptide did not significantly affect the behavioral or autonomic responses during the two behavioral stressors, nor did it alter CRH levels in the PVN in the paired group. In contrast, administration of exogenous oxytocin was associated with a reduced density of CRH-immunoreactive cell bodies in the PVN in the isolated group. These findings indicate that exogenous oxytocin may have the capacity to block the increase in CRH synthesis that typically accompanies a chronic stressor (e.g. in the absence of supportive social stimuli).
The current results may also be informed by the finding that oxytocin administered intranasally interacts with perceived social support to down-regulate the HPA axis and HR responses in humans (Heinrichs et al. Citation2003). Additional studies are required to fully understand the release patterns of both oxytocin and CRH from the PVN following social isolation and oxytocin administration. A limitation of this study is that the central nervous system changes were investigated several days following the final oxytocin injection, with exposure to two behavioral stressors during the intervening period. Although care was taken to ensure that the voles had a sufficient amount of time to recover from the disruption of the behavioral stressors before the collection of brain tissue, some of the changes observed in the PVN are due to complex interactions among social isolation, oxytocin administration, and exposure to acute behavioral stressors.
The precise pathways by which peripherally administered oxytocin may exert a protective effect on autonomic responses to stressors require further investigation. As noted above, oxytocin receptors are present in cardiac tissue (Jankowski et al. Citation2004), which is a potential site of action for the exogenously administered oxytocin in this study. However, peripherally administered oxytocin may also influence central oxytocin function via several mechanisms. The oxytocin molecule or biologically active fragments of the peptide molecule may access the brain through the circumventricular organs (De Wied et al. Citation1993). Peripheral treatments with this peptide might also act indirectly on the brain via the afferent vagus. Oxytocin receptors have been identified in several central autonomic nuclei, including the dorsal motor nucleus of the vagus, nucleus amgibuus, and nucleus tractus solitarius (Higa et al. Citation2002). These are likely sites of action for central oxytocin to influence autonomic regulation of the heart during periods of social isolation and exposure to acute stressors.
The present findings provide evidence that oxytocin may serve to modulate autonomic responses to social isolation, especially within the context of acute stressors. It is possible that peripherally administered oxytocin influences the central nervous system, which can affect the levels or release of oxytocin and CRH within the PVN. Future studies will benefit from an investigation of the specific processes through which this and other neuropeptides influence the integration of behavioral and physiological responses to stressors, including a comparison of peripheral and central actions of these substances. Additional mechanistic studies with translational animal models will improve our understanding of neurobiological and psychological processes that underlie reactions to environmental and social stressors in humans.
Acknowledgments
The authors are grateful to Dr Ann-Judith Silverman for supplying the CRH antibody and to Dr Mariana Morris for supplying the oxytocin antibody. The investigators also thank Iman Hassan, William Homan, Damon Lamb, Meagan LaRocca, Gregory Lewis, Maulin Shah, and Robert Zimmerman II for technical assistance. This research was funded by the following National Institutes of Health grants: MH73233 (AJG), MH77581 (AJG), MH67446 (SWP), and MH72935 (CSC); and the following Northern Illinois University units: College of Liberal Arts and Sciences, Division of Research and Graduate Studies, and Center for Biochemical and Biophysical Studies.
Declaration of interest: The authors report no conflicts of interest. The authors alone are responsible for the content and writing of the paper.
References
- Anisman H, Zacharko RM. 1982. Depression: The predisposing influence of stress. Behav Brain Sci. 5:89–137.
- Blanchard RJ, McKittrick CR, Blanchard DC. 2001. Animal models of social stress: Effects on behavior and brain neurochemical systems. Physiol Behav. 73:261–271.
- Bosch OJ, Krömer SA, Brunton PJ, Neumann ID. 2004. Release of oxytocin in the hypothalamic paraventricular nucleus, but not central amygdala or lateral septum in lactating residents and virgin intruders during maternal defence. Neuroscience. 124:439–448.
- Bosch OJ, Nair HP, Ahern TH, Neumann ID, Young LJ. 2009. The CRF system mediates increased passive stress-coping behavior following the loss of a bonded partner in a monogamous rodent. Neuropsychopharmacology. 34:1406–1415.
- Cacioppo JT. 2002. Social neuroscience: Understanding the pieces fosters understanding the whole and vice versa. Am Psychologist. 57:819–831.
- Carter CS, DeVries AC, Getz LL. 1995. Physiological substrates of mammalian monogamy: The prairie vole model. Neurosci Biobehav Rev. 19:303–314.
- Carter CS, Grippo AJ, Pournajafi-Nazarloo H, Ruscio MG, Porges SW. 2008. Oxytocin, vasopressin and sociality. Prog Brain Res. 170:331–336.
- Carter CS, Keverne EB. 2002. The neurobiology of social affiliation and pair bonding. Horm Brain Behav. 1:299–337.
- Carter CS, Witt DM, Schneider J, Harris ZL, Volkening D. 1987. Male stimuli are necessary for female sexual behavior and uterine growth in prairie voles (Microtus ochrogaster). Horm Behav. 21:74–82.
- Cushing BS, Carter CS. 2000. Peripheral pulses of oxytocin increase partner preferences in female, but not male, prairie voles. Horm Behav. 37:49–56.
- Cushing BS, Klein D, Hoffman GE, Carter CS, Le WW, De Vries GJ. 2001. Comparison of fixation techniques: Immersion versus perfusion. Horm Behav. 39:329.
- De Wied D, Diamant M, Fodor M. 1993. Central nervous system effects of the neurohypophyseal hormones and related peptides. Front Neuroendocrinol. 14:251–302.
- DeAngelis T. 2010. Heartfelt interventions. Am Psychologist. 41:48–49.
- Dingfelder SF. 2006. Socially isolated and sick. Monitor Psy. 37:18–19.
- Ditzen B, Neumann ID, Bodenmann G, von Dawans B, Turner RA, Ehlert U, Heinrichs M. 2007. Effects of different kinds of couple interaction on cortisol and heart rate responses to stress in women. Psychoneuroendocrinology. 32:565–574.
- Falke N. 1989. Oxytocin stimulates oxytocin release from isolated nerve terminals of rat neural lobes. Neuropeptides. 14:269–274.
- Gehrmann J, Hammer PE, Maguire CT, Wakimoto H, Triedman JK, Berul CI. 2000. Phenotypic screening for heart rate variability in the mouse. Am J Physiol Heart Circ Physiol. 279:H733–H740.
- Getz LL, Carter CS. 1996. Prairie-vole partnerships. Am Sci. 84:56–62.
- Grant N, Hamer M, Steptoe A. 2009. Social isolation and stress-related cardiovascular, lipid, and cortisol responses. Ann Behav Med. 37:29–37.
- Grippo AJ. 2009. Mechanisms underlying altered mood and cardiovascular dysfunction: The value of neurobiological and behavioral research with animal models. Neurosci Biobehav Rev. 33:171–180.
- Grippo AJ, Carter CS, McNeal N, Chandler DL, LaRocca MA, Bates SL, Porges SW. 2011. 24-Hour autonomic dysfunction and depressive behaviors in an animal model of social isolation: Implications for the study of depression and cardiovascular disease. Psychosom Med. 73:59–66.
- Grippo AJ, Cushing BS, Carter CS. Depression-like behavior and stressor-induced neuroendocrine activation in female prairie voles exposed to chronic social isolation. Psychosom Med. 2007a; 69:149–157.
- Grippo AJ, Gerena D, Huang J, Kumar N, Shah M, Ughreja R, Carter CS. Social isolation induces behavioral and neuroendocrine disturbances relevant to depression in female and male prairie voles. Psychoneuroendocrinology. 2007b; 32:966–980.
- Grippo AJ, Lamb DG, Carter CS, Porges SW. Cardiac regulation in the socially monogamous prairie vole. Physiol Behav. 2007c; 90:386–393.
- Grippo AJ, Lamb DG, Carter CS, Porges SW. Social isolation disrupts autonomic regulation of the heart and influences negative affective behaviors. Biol Psychiatry. 2007d; 62:1162–1170.
- Grippo AJ, Sgoifo A, Mastorci F, McNeal N, Trahanas DM. 2010. Cardiac dysfunction and hypothalamic activation during a social crowding stressor in prairie voles. Autonom Neurosci Basic Clin. 156:44–50.
- Grippo AJ, Trahanas DM, Zimmerman PorgesSWII RR, Carter CS. 2009. Oxytocin protects against negative behavioral and autonomic consequences of long-term social isolation. Psychoneuroendocrinology. 34:1542–1553.
- Grippo AJ, Wu KD, Hassan I, Carter CS. 2008. Social isolation in prairie voles induces behaviors relevant to negative affect: Toward the development of a rodent model focused on co-occurring depression and anxiety. Depress Anxiety. 25:E17–E26.
- Heinrichs M, Baumgartner T, Kirschbaum C, Ehlert U. 2003. Social support and oxytocin interact to suppress cortisol and subjective responses to psychosocial stress. Biol Psychiatry. 54:1389–1398.
- Higa KT, Mori E, Viani FF, Morris M, Michelini LC. 2002. Baroreflex control of heart rate by oxytocin in the solitary-vagal complex. Am J Physiol Regul Integr Comp Physiol. 282:R537–R545.
- Ishii K, Kuwahara M, Tsubone H, Sugano S. 1996. Autonomic nervous function in mice and voles (Microtus arvalis): Investigation by power spectral analysis of heart rate variability. Lab Animals. 30:359–364.
- Jankowski M, Danalache B, Wang D, Bhat P, Hajjar F, Marcinkiewicz M, Paquin J, McCann SM, Gutkowska J. 2004. Oxytocin in cardiac ontogeny. Proc Natl Acad Sci. 31:13074–13079.
- Kalinichev M, Easterling KW, Plotsky PM, Holtzman SG. 2002. Long-lasting changes in stress-induced corticosterone response and anxiety-like behaviors as a consequence of neonatal maternal separation in Long-Evans rats. Pharmacol Biochem Behav. 73:131–140.
- Kim JW, Kirkpatrick B. 1996. Social isolation in animal models of relevance to neuropsychiatric disorders. Biol Psychiatry. 40:918–922.
- Konkle AT, Baker SL, Kentner AC, Barbagallo LSM, Merali Z, Bielajew C. 2003. Evaluation of the effects of chronic mild stressors on hedonic and physiological responses: Sex and strain compared. Brain Res. 992:227–238.
- Mantella RC, Vollmer RR, Rinaman L, Li X, Amico JA. 2004. Enhanced corticosterone concentrations and attenuated Fos expression in the medial amygdala of female oxytocin knockout mice exposed to psychogenic stress. Am J Physiol Regul Integr Comp Physiol. 287:1495–1504.
- McCabe PM, Gonzales JA, Zaias J, Szeto A, Kumar M, Herron AJ, Schneiderman N. 2002. Social environment influences the progression of atherosclerosis in the Watanabe heritable hyperlipidemic rabbit. Circulation. 105:354–359.
- McGraw LA, Young LJ. 2009. The prairie vole: An emerging model organism for understanding the social brain. Trends Neurosci. 33:103–109.
- Mitchell PJ, Fairhall SJ, Fletcher A, Redfern PH. 2003. Effects of single and repeated electroconvulsive shock on the social and agonistic behaviour of resident rats. Neuropharmacology. 44:911–925.
- Neumann ID, Torner L, Wigger A. 2000. Brain oxytocin: Differential inhibition of neuroendocrine stress responses and anxiety-related behaviour in virgin, pregnant and lactating rats. Neuroscience. 95:567–575.
- Norman GJ, Cacioppo JT, Morris JS, Malarkey WB, Berntson GG, DeVries AC. 2011. Oxytocin increases autonomic cardiac control: Moderation by loneliness. Biol Psychol. 86:174–180.
- Norman GJ, Zhang N, Morris JS, Karelina K, Berntson GG, DeVries AC. 2010. Social interaction modulates autonomic, inflammatory, and depressive-like responses to cardiac arrest and cardiopulmonary resuscitation. Proc Natl Acad Sci. 107:16342–16347.
- Paxinos G, Watson C. 2005. The rat brain in stereotaxic coordinates. New York: Elsevier.
- Pellow S, Chopin P, File SE, Briley M. 1985. Validation of open: closed arm entries in an elevated plus-maze as a measure of anxiety in the rat. J Neurosci Methods. 14:149–167.
- Pierrehumbert B, Torrisi R, Laufer D, Halfon O, Ansermet F, Beck Popovic M. 2010. Oxytocin response to an experimental psychosocial challenge in adults exposed to traumatic experiences during childhood or adolescence. Neuroscience. 166:168–177.
- Porges SW. 1985. Method and apparatus for evaluating rhythmic oscillations in aperiodic physiological response systems Patent Number 4510944: April 16. Alexandria, VA: United States Patent and Trademark Office.
- Porges SW. The polyvagal perspective. Biol Psychol. 74:116–143.
- Porges SW, Bohrer RE. 1990. Analyses of periodic processes in psychophysiological research. In: Cacioppo JT, Tassinary LG. editors. Principles of psychophysiology: Physical, social, and inferential elements. New York: Cambridge University Press708–753.
- Porges SW, Byrne EA. 1992. Research methods for measurement of heart rate and respiration. Biol Psychol. 324:93–130.
- Ruscio MG, Sweeny T, Hazelton J, Suppatkul P, Carter CS. 2007. Social environment regulates corticotropin releasing factor, corticosterone and vasopressin in juvenile prairie voles. Horm Behav. 51:54–61.
- Schwerdtfeger A, Friedrich-Mai P. 2009. Social interaction moderates the relationship between depressive mood and heart rate variability: Evidence from an ambulatory monitoring study. Health Psychol. 28:501–509.
- Sgoifo A, Koolhaas J, Alleva E, Musso E, Parmigiani S. 2001. Social stress: Acute and long-term effects on physiology and behavior. Physiol Behav. 73:253–254.
- Sgoifo A, Stilli D, Medici D, Gallo P, Aimi B, Musso E. 1996. Electrode positioning for reliable telemetry ECG recordings during social stress in unrestrained rats. Physiol Behav. 60:1397–1401.
- Slattery DA, Neumann ID. 2010. Chronic icv oxytocin attenuates the pathological high anxiety state of selectively bred Wistar rats. Neuropharmacology. 58:56–61.
- Steptoe A, Owen N, Kunz-Ebrecht SR, Brydon L. 2004. Loneliness and neuroendocrine, cardiovascular, and inflammatory stress responses in middle-aged men and women. Psychoneuroendocrinology. 29:593–611.
- Takayanagi Y, Yoshida M, Bielsky IF, Ross HE, Kawamata M, Onaka T, Yanagisawa T, Kimura T, Matzuk MM, Young LJ, Nishimori K. 2005. Pervasive social deficits, but normal parturition, in oxytocin receptor-deficient mice. Proc Natl Acad Sci. 102:16096–16101.
- Task Force of the European Society of Cardiology, North American Society of Pacing and Electrophysiology. 1996. Heart rate variability: Standards of measurement, physiological interpretation, and clinical use. Circulation. 93:1043–1065.
- Taylor SE, Gonzaga GC, Klein LC, Hu P, Greendale GA, Seeman TE. 2006. Relation of oxytocin to psychological stress responses and hypothalamic-pituitary-adrenocortical axis activity in older women. Psychosom Med. 68:238–245.
- Uvnäs-Moberg K, Ahlenius S, Hillegaart V, Alster P. 1994. High doses of oxytocin cause sedation and low doses cause an anxiolytic-like effect in male rats. Pharmacol Biochem Behav. 49:101–106.
- Wang ZX, Zhuo L, Hulihan TJ, Insel TR. 1996. Immunoreactivity of central vasopressin and oxytocin pathways in microtine rodents: A quantitative comparative study. J Comp Neurol. 366:726–737.
- Windle RJ, Gamble LE, Kershaw YM, Wood SA, Lightman SL, Ingram CD. 2006. Gonadal steroid modulation of stress-induced hypothalamo-pituitary-adrenal activity and anxiety behavior: Role of central oxytocin. Endocrinology. 147:2423–2431.
- Windle RJ, Shanks N, Lightman SL, Ingram CD. 1997. Central oxytocin administration reduces stress-induced corticosterone release and anxiety behavior in rats. Endocrinology. 138:2829–2834.
- Yee JR, Frijling J, Saber M, Sterlinski A, Tovar S, Barlas L, Pournajafi-Nazarloo H, Lewis G, Stevenson J, Kenkel W, Porges SW, Carter CS. 2010. Oxytocin alters the behavioral, cardiovascular, and hormonal responses to a mild daily stressor Soc Neurosci Abstr, Available at http://www.abstractsonline.com/Plan/ViewAbstract.aspx?sKey = c5ef6681-4c75-4ab4-9188-bde89eb16999&cKey = 70011bb2-e182-4ad4-bd3c-2e1d01ea1e6d&mKey = %7bE5D5C83F-CE2D-4D71-9DD6-FC7231E090FB%7d.
- Yongue BG, McCabe PM, Porges SW, Rivera M, Kelley SL, Ackles PK. 1982. The effects of pharmacological manipulations that influence vagal control of the heart on heart period, heart-period variability and respiration in rats. Psychophysiology. 19:426–432.