Abstract
Effective coping strategies and adaptive behavioral training build resilience against stress-induced pathology. Both predisposed and acquired coping strategies were investigated in rats to determine their impact on stress responsiveness and emotional resilience. Male Long-Evans rats were assigned to one of the three coping groups: passive, active, or variable copers. Rats were then randomly assigned to either an effort-based reward (EBR) contingent training group or a non-contingent training group. Following EBR training, rats were tested in appetitive and stressful challenge tasks. Physiological responses included changes in fecal corticosterone and dehydroepiandrosterone (DHEA) metabolites as well as neuropeptide Y (NPY)-immunoreactivity in the hippocampus and amygdala. Regardless of a rat's predisposed coping strategy, EBR rats persisted longer than non-contingent rats in the appetitive problem-solving task. Furthermore, training and coping styles interacted to yield the seemingly most adaptive DHEA/corticosterone ratios in the EBR-trained variable copers. Regardless of training group, variable copers exhibited increased NPY-immunoreactivity in the CA1 region.
Introduction
Mood disorders (MDs) such as depression and bipolar disorder are among the most common neuropsychiatric illnesses: almost 44 million Americans are affected by MDs, with twice as many women than men affected (Zender and Olshansky Citation2009; Gelenberg Citation2010; Horwitz Citation2010). Focusing specifically on major depression disorder (MDD), lifetime prevalence rates of approximately 17% have recently been reported (Kessler et al. Citation2010). MDD rates remain high despite the increased use of antidepressant medication treatments, increasing nearly twofold from 1996 to 2005 in the USA, from 5.8% to 10% (Olfson and Marcus Citation2009). Additionally, increased antidepressant prescriptions have been accompanied by a decrease in patients undergoing psychotherapy (from 32 to 20%) (Olfson and Marcus Citation2009). Thus, it appears that the emphasis on psychological dimensions of antidepressant therapy has diminished in the context of increased reliance on antidepressant prescriptions (Kirsch Citation2009).
Psychotherapies such as behavioral activation therapy, emphasizing techniques including activity monitoring, skills training, and contingency management, have been successful in the treatment of depression (Kanter et al. Citation2010), possibly due to altered activation in prefrontal brain structures (Dichter et al. Citation2010). A challenge with this therapy, however, has been the lack of animal models to examine critical neurobiological changes accompanying symptom improvement or enhanced resilience against emerging or chronic stress responses that often accompany depressive symptoms (Duman Citation2009). The effort-based reward (EBR) model, a semi-naturalistic behavioral training model requiring the animal to adjust foraging strategies daily to maximize rewards, has been proposed as a training technique that enhances resilience against the onset of depressive symptoms (Lambert Citation2006). This model requires rats to exert physical effort to obtain rewards each day, potentially activating brain areas typically implicated in depression such as the prefrontal cortex (PFC), nucleus accumbens, and striatum (Dichter et al. Citation2010). Interestingly, the striatum, involved in movement control, has close neurobiological ties to the nucleus accumbens (Kelley Citation1999; Zahm Citation1999). Unmedicated patients diagnosed with depression exhibit altered fMRI activation levels in the striatum and PFC, as well as altered responsivity to an amphetamine challenge, implicating the nucleus accumbens in addition to the striatum and PFC as key neuroanatomical areas associated with depression (Tremblay et al. Citation2002; Amat et al. Citation2005).
Past research in our laboratory has indicated that, when faced with a challenge task, EBR contingently trained animals persist longer than control, non-contingent animals (Lambert et al. Citation2006). There is ample evidence in rats indicating that task contingency training, resulting in increased associations between effort/responses and consequences, enhances prefrontal executive and cognitive functions, likely influenced by dopaminergic activity (Neill et al. Citation2002; Dalley et al. Citation2004; Ishiwari et al. Citation2004; Salamone et al. Citation2009). We also found that variable copers, defined as animals that demonstrated a variable as opposed to a rigid response to stress, exhibited more seemingly adaptive responsiveness in three successive forced swim tasks (FSTs) than the more consistently responding passive and active copers. This behavioral flexibility was accompanied by increased neuropeptide Y (NPY)-immunoreactivity in the bed nucleus of the stria terminalis and the amygdala (Hawley et al. Citation2010). NPY has received extensive and consistent support as one of the most prominent peptides implicated in the brain's resilience systems (Mathew et al. Citation2008; Rotzinger et al. Citation2010; Thorsell Citation2010). Specifically, anti-anxiety effects of NPY have been demonstrated in several human (Sah et al. Citation2009) and animal models (primates: Lindell et al. Citation2010; rodents: Gutman et al. Citation2008; invertebrates: Xu et al. Citation2010). Evidence suggests that the NPY-induced long-term behavioral resilience to stress may occur via mechanisms involving neuronal plasticity (Sajdyk et al. Citation2008).
In our laboratory, we have also found evidence for the role of dehydroepiandrosterone (DHEA) in mediating stress and coping responses (Hawley et al. Citation2010; Bardi et al. Citation2011a). DHEA is released concurrently with cortisol during physical stress (Charney Citation2004; Izawa et al. Citation2008), protecting the body against the negative effects of prolonged exposure to glucocorticoids (Morgan et al. Citation2004). Moreover, DHEA can act centrally to decrease glucocorticoid-induced neuronal death in the hippocampus and to promote neurogenesis in the dentate gyrus (DG) of the hippocampus and in sensory dorsal root ganglion neurons (Maninger et al. Citation2009; Pinnock et al. Citation2009; Ulmann et al. Citation2009). Furthermore, the ratio between DHEA and cortisol has been found to be a reliable index of neuroprotection (Maninger et al. Citation2009). Specifically, higher corticosteroid levels can be adaptive in some situations: corticosteroids, for example, facilitate both the storage of novel information and the extinction of behavior that is no longer adaptive or relevant in a particular situation (Oitzl et al. Citation2010). At the same time, the organism needs to be protected by the negative effects of high corticosterone levels. Consequently, the emerging theory from recent stress research suggests that higher DHEA concentrations (among other chemicals able to counteract stress), as compared to corticosterone concentrations, can indeed confer an advantage in demanding environments (Wemm et al. Citation2010; Bardi et al. Citation2011b).
Given previous findings that an animal's coping profile influences responsiveness to stress (Oortmerssen and Busser Citation1989; Koolhaas et al. Citation1999; Van Erp-an der Kooij et al. Citation2000; Hawley et al. Citation2010), the goal of the current study was to investigate the impact of EBR training on each of the coping profiles used previously in our laboratory (i.e. passive, active, and variable) to determine whether these predisposed responses to stress can be altered by EBR training. Specifically, following profiling with the back-test, rats were exposed to either EBR contingent training or non-contingent training, and the following neurobiological measures of resilience were subsequently observed: behavioral persistence in a behavioral challenge task, response choice in repeated forced swim tasks (FSTs), stress hormone (e.g. glucocorticoid and DHEA) levels, and NPY immunoreactivity in brain areas associated with stress and anxiety. Past research in our laboratory revealed enhanced NPY activation in the brains of variable copers, as well as more adaptive stress responses (Hawley et al. Citation2010). In the current study, it was hypothesized that contingent EBR training would enhance neurobiological resilience; however, due to lack of prior research in the area, no directional hypothesis was generated for the potential differential impact on animals exhibiting various coping strategies.
Methods and materials
Subjects
Forty male Long-Evans rats (Rattus norvegicus), 20 days old, originally purchased from Harlan (Indianapolis, IN, USA), were employed in this study. Upon arrival at the laboratory, rats were housed in 48 cm × 26 cm × 21 cm wire-lidded cages to habituate to laboratory conditions prior to the commencement of the experiments. Following the coping profile assessment, rats with the most extreme scores were selected, with 28 animals in total. The rats were pair-housed for the remainder of the study. Cages were lined with aspen bedding; additionally, food and water were provided ad libitum until the first week of training was completed. A 12:12 h light–dark cycle began with lights on at 06:00 h; ambient temperature was maintained at 24°C. All rats were maintained in accordance with the guidelines of the Randolph-Macon College Institutional Animal Care and Use Committee.
Following the coping profile assessment and habituation, the rats were food deprived for approximately 12 h prior to the commencement of cognitive testing. Upon the completion of the first day of testing, food was returned and the rats were once again kept on an ad libitum diet. At the beginning of the second week of training, rats were placed on a continuous restricted diet to maintain motivation to work in the task.
Materials
The training apparatus used for EBR training was 122 cm × 9 cm × 5 cm and was constructed of wood with the floor covered in gray linoleum. A plastic cat toy ball was used during the persistence task (PT) (). The Froot Loop® (Kellogg's®; Battle Creek, MI, USA) reward was also placed in the ball's interior.
The FST was used to assess the rats' stress responses to an inescapable paradigm. Rats were placed in 60 cm × 31 cm × 53 cm glass aquarium for 3 min, with water depth of 30 cm, maintained at room temperature (24°C).
Coping strategy assessment
To determine each rat's coping strategy, a back-test was conducted as described in Hawley et al. (Citation2010). Briefly, during the back-test, the rats were gently restrained in a supine position by holding them at the nape of the neck as well as the base of the tail. To maintain consistency in these tests, the same trained observers were used for all back-test assessments. During this 1-min test, the number of attempts to escape (defined as “wiggling” or movement of the limbs) was recorded for 60 s. When a rat paused during the escape attempt, a new bout was recorded (Geverink et al. Citation2004). Previous research in our laboratory indicated that the escape responses appear in bouts rather than one continuous attempt to escape; therefore, the number of escape attempt bouts was recorded in the present study (Hawley et al. Citation2010). Following the test, rats were returned to their cages. Seven days later, a second back-test was conducted in exactly the same manner as the initial test so that the scores from the first and second assessments could be compared. The number of escape attempts in each test ranged from 1 to 12 responses. Three categories were subsequently created to categorize the coping strategies: active, passive, and variable. Rats were categorized as active copers if they exhibited seven or more escape attempts in both back-test assessments (n = 9). Rats were considered passive if they exhibited six or fewer escape attempts during both assessments (n = 10). Rats that were categorized as active in one trial and passive on the other trial were categorized as variable copers (n = 9).
Behavioral assessments
Half the rats assigned to each coping style were randomly assigned to one of the two treatment conditions in the EBR paradigm: contingent (n = 14) and non-contingent training groups (n = 14). Consequently, we had five contingent and four non-contingent active rats; five contingent and five non-contingent passive rats; and four contingent and five non-contingent variable coping rats. Contingent rats had Froot Loop® pieces placed on top of the four mounds of bedding created within the digging apparatus. Non-contingent rats were placed in the same apparatus daily but in this condition the Froot Loops® were placed in the designated starting corner of the apparatus (no responses were necessary for rewards).
Upon placement into the apparatus each rat had up to 5 min to gather all four halves of the Froot Loop® rewards; the position of the mounds varied daily. Contingent rats were removed from the apparatus immediately after retrieving all four rewards or, if all rewards were not retrieved, after 5 min had passed. There was no significant difference in the average amount of time spent in the apparatus between the two groups. The training continued 5 days per week for 4 weeks and was conducted approximately 3 h after lights on every day, starting at 09:00 h.
After training was completed, the rats were exposed to a PT during the next day of testing. A Froot Loop® was placed inside a plastic cat toy ball that also had a bell inside it. The rats could see, smell, and touch the cereal reward but could not retrieve it from the container (). Each rat was placed in the apparatus in the same location they had been placed in for the previous 4 weeks. Following a 5-min habituation period, the ball was placed in the center of the apparatus and the following behaviors were observed: latency to contact the ball, number of contacts, duration of contact, duration of contact bout, grooming, freezing, digging, and number of ball pick-ups. The PT was the same for both the contingent and non-contingent rats.
Starting on the same day of the PT, each rat was exposed to a 3-min FST at 18:00 h for 3 consecutive days. After being placed in the tank, the following behaviors were observed: (1) number and duration of floating and swimming bouts, and (2) number of the following events: diving, wet shakes, and fecal boli excreted. Upon completion of the first and third swim tasks, fecal samples were collected at 06:00 h the following morning in order to assess the swim-induced alterations in corticosterone and DHEA levels at the appropriate time delay for fecal detection of metabolites (Bardi et al. Citation2010).
Physiological responses
Fresh fecal samples were collected at the baseline (prior to EBR training) and after days 1 and 3 during the FST. All fecal samples were stored at − 70°C and assayed with standard methods (Bardi et al. Citation2010). Briefly, to extract corticosterone and DHEA from the samples, wet samples were weighed so that 0.1 g could be transferred to individually labeled test tubes. The fecal samples were added to 1 ml of 100% methanol in a glass test tube and were homogenized with the large end of a glass Pasteur pipette. Each sample was then vortexed for 30 s and centrifuged for 10 min at 1500 g. The supernatant was transferred to a 13 × 10 mm glass test tube with a transfer pipette. Samples were diluted at a ratio of 1:20 for corticosterone and 1:10 for DHEA with the buffers from the ImmunoAssay kit (AssayDesigns, Ann Arbor, MI, USA). Assays were processed on an ELx8000 microplate reader interfaced with KC Junior software (Bio Tek, Winooski, VT, USA). Samples were analyzed in duplicate. The cross-reactivity of the DHEA kit was 100% with DHEA, 30% with DHEA sulfate, and under 1% with other steroids. The cross-reactivity of the corticosterone kit was 100% with corticosterone, 8.6% with deoxycorticosterone, and below 1% with other steroids. Hence, the assay measurements include DHEA and corticosterone metabolites. Dose–response curves for the assays, assessed by spiking sample extracts with a known amount of DHEA and corticosterone standard in increasing amounts, generated a curve with an average slope of 0.93 and a correlation coefficient of 0.943. Intra-assay precision for our samples was in the range of the kit standards (DHEA average CV = 9%; corticosterone average CV = 8%). Inter-assay average coefficient of variations were 13% for DHEA and 11% for corticosterone.
Histological assessments
Following the forced swim assessment, rats were individually placed into an airtight chamber with 1 ml of Halothane liquid (Sigma-Aldrich, St Louis, MO, USA) until respiratory rate slowed and rats were non-responsive. Rats were then given an intraperitoneal injection of 0.2 ml sodium pentobarbitol at an overdose of >40 mg/kg. They were transcardially perfused at 40 ml/min using a MasterFlex L/S perfusion pump with 100 ml phosphate-buffered saline (PBS) solution followed by 100 ml 4% paraformaldehyde solution. Brains were extracted and post-fixed in 4% paraformaldehyde overnight at 4°C, then transferred to a 10% sucrose solution for 24 h at 4°C, and were then stored in 20% sucrose solution at 4°C until sectioning (at least 24 h later). Brains were sectioned coronally at − 25°C using a HM525 Microm cryostat. Thirty-six sections were cut at 40 μm thickness in order to capture sections throughout the hippocampus, basolateral amygdala, and DG. Every sixth section was kept for NPY ICC and sections were stored in PBS at 4°C in 24-well plates.
Six free-floating sections from each brain were assessed for NPY immunoreactivity. After a 10-min wash in 0.3% hydrogen peroxide to quench endogenous peroxidase activity, sections were blocked for 1 h in 10% normal goat serum in PBST (0.3% Triton-X, Spectrum Chemical, Gardena, CA, USA). Sections were then incubated overnight at 4°C in a primary antibody for NPY (1:4000; ImmunoStar Inc., Hudson, WI, USA), exposed for 1 h to goat anti-rabbit secondary antibody (Vector, Burlingame, CA, USA) at 1:200, processed with a standard Vectastain ABC kit (Vector) and visualized with a DAB peroxidase substrate kit (Vector). Stained sections were mounted onto gelatinized slides, cleared through a series of 70, 95, and 100% EtOH and Citrisolv washes, and coverslipped using Permount.
Histomorphometry
A Zeiss Axioskop light microscope was used to identify the CA1, CA3, and DG in the hippocampus and the basolateral amygdala. For the NPY-immunoreactivity analysis, light-thresholding analysis was carried out on a 200 × 250 micron field of view (at a magnification of 400 × ) using Bioquant Neuroimaging software (Nashville, TN, USA). Mean optical density for NPY-ir tissue was measured as percentage of the total immunoreactive tissue present in the specific field of vision. Optical density was preferred over number of NPY-ir neurons to provide a consistent measure across all areas quantified, including the amygdala where the presence of fibers make it difficult to quantify the number of neurons. To assure that the optical density was equivalent to the number of immunoreactive neurons, the NPY-ir neurons in the CA1 region were counted and correlated with the mean optical density in the same area; a significant positive correlation (r = 0.67, p < 0.001) was observed.
Statistical analysis
For each dependent variable, a factorial ANOVA with repeated measures (RM) was used to determine the effects of training (contingent vs. non-contingent EBR training) and coping strategies (passive, active, or variable) on each dependent measure. ANOVA with RM was used to assess the effects of training (2) and coping style (3) during the FST (3). A Tukey test was used for post hoc comparisons (α = 0.05), and estimated marginal means were used to calculate the confidence intervals for each group in the interaction training × coping style. SPSS statistical software (Chicago, IL, USA) was used for all statistical processing. Owing to the small sample size in each subgroup, statistical power was never enough to rule out Type II errors when non-significant results were found (power < 0.45 in all analyses). Consequently, a multivariate non-parametric analysis, multi-dimensional scaling (MDS), was used to further assess the data.
The MDS analysis provides a model of independent associations among the variables. The MDS constructed a visual representation or “map” of the distance between the variables. The usefulness of this technique comes from the fact that it generates a visual representation of the relationship among the variables without any knowledge or assumption of the reciprocal association. The parameters of the MDS technique include the Kruskal stress index and the R2 value. Goodness of fit, or how well the variables were accommodated by the dimensions of the model, was established by the Kruskal stress index. A stress value of 0.15 or lower was accepted as an indicator of good fit. The R2 is a percent value that determines the proportion of variance explained by the data set (Manly Citation1994).
Results
Persistence task
Coping style significantly affected performance in the PT (F2,22 = 3.59, p = 0.045; ). Rats classified as variable copers exhibited longer total contact durations with the ball than both active and passive copers (mean, 38 s vs. 27 s). Training also significantly affected the duration of contact with the ball (F1,22 = 14.5, p < 0.01), with contingent males exhibiting significantly longer contact total durations than the non-contingent rats (mean, 34 s vs. 28 s). No significant interactions between coping style and training were observed (F2,22 = 0.13, ns).
Figure 2. Mean duration of contact with the ball during the PT grouped by different coping styles and effort-reward training). Variable copers (n = 9) had longer total contact with the ball than active (n = 9) and passive (n = 10) copers (ANOVA, p = 0.045). Contingent rats (n = 14) had more total contact with the ball than non-contingent rats (n = 14) (ANOVA, p < 0.01). *p < 0.05 vs. other groups, Tukey. Groups: contingent variable, n = 4; non-contingent variable, n = 5; contingent active, n = 5; non-contingent active, n = 4; contingent passive, n = 5; non-contingent passive, n = 5.
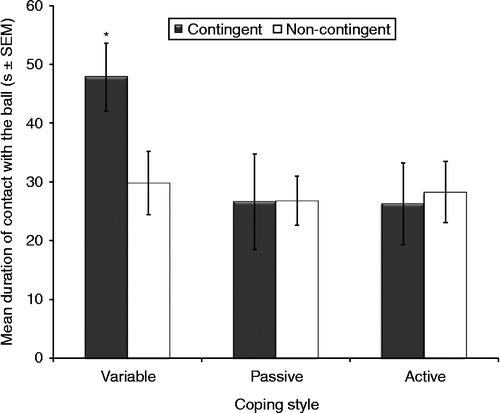
Coping style failed to significantly affect bout durations in the PT (F2,22 = 0.97, ns). When in contact with the ball, contingent rats stayed on task significantly longer than non-contingent rats (7 s vs. 5 s; F1,22 = 5.25, p = 0.032). Additionally, no significant interactions between coping style and training were observed (F2,22 = 0.72, ns).
All other behavioral measures taken during the PT (latency of contact with the ball, grooming, number of pickups, digging, and number of fecal boli excreted) were not significantly influenced by either coping style or training group.
Forced swim task
All rats spent significantly more time on floating in each test from day 1 through to day 3 (F2,44 = 23.1, p < 0.001). Variable copers spent significantly more time on floating on all 3 days than all active and passive copers (mean, 53 s vs. 43 s; F2,22 = 7.71, p = 0.041). Additionally, contingent males spent more time on floating than non-contingent males (mean, 55 s vs. 32 s; F1,22 = 15.4, p < 0.001). Specifically, a significant increase in the time spent on floating in each test from day 1 to day 3 was observed in contingent males. Furthermore, a significant interaction was found between coping style and training (F2,22 = 3.51, p = 0.047); specifically, contingent, variable copers spent significantly more time floating than any other groups ().
Figure 3. Mean floating duration during the FST. Contingent rats (n = 14) floated more than non-contingent rats (n = 14) (ANOVA, p < 0.001). Coping style and training interacted (RM ANOVA, p = 0.047): contingent, variable copers floated longer than other groups. *p < 0.05 vs. other groups, Tukey. Groups: contingent variable, n = 4; non-contingent variable, n = 5; contingent active, n = 5; non-contingent active, n = 4; contingent passive, n = 5; non-contingent passive, n = 5.
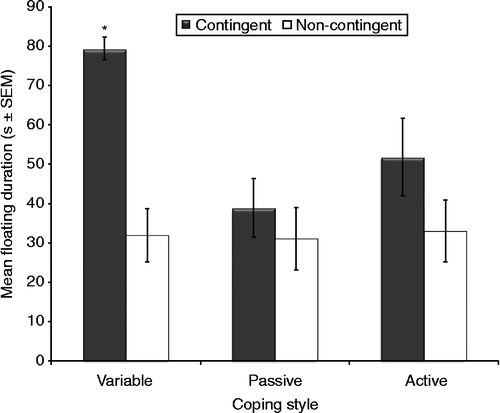
Although a decreased number of dives from day 1 to day 3 was observed for all rats, it was not statistically significant (F2,44 = 2.47, p = 0.097). Furthermore, coping style did not affect the number of dives (F2,22 = 0.43, ns) or the interaction between coping and training (F2,22 = 0.11, ns). A significant interaction between day and training, however, was found (F2,44 = 3.99, p = 0.026). Specifically, contingent rats attempted diving significantly more than non-contingent rats on the first day of the FST (). All other behavioral measures taken during the FST (shakes, grooming attempts, and number of fecal boli excreted) were not significantly affected by coping style or training.
Figure 4. Number of dives in the 3 days of testing in the FST. Day and training interacted (RM ANOVA, p = 0.026). Contingent males (n = 14) dived more than all other groups. *p < 0.05 vs. other groups, Tukey. Groups: contingent variable, n = 4; non-contingent variable, n = 5; contingent active, n = 5; non-contingent active, n = 4; contingent passive, n = 5; non-contingent passive, n = 5.
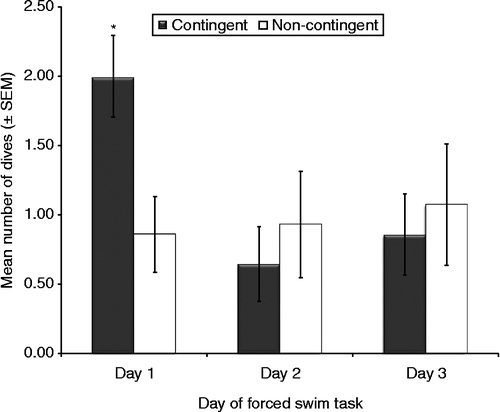
Physiological responses to the FST
The FST significantly induced physiological responses: increased fecal concentrations of corticosterone (F1,22 = 62.2, p < 0.001) and DHEA metabolites (F1,22 = 44.5, p < 0.001; ). A significant interaction was also observed between training and coping style for corticosterone (F2,22 = 3.58, p = 0.045), but not for DHEA (F2,22 = 1.24, ns). Variable copers exhibited the lowest concentrations of both corticosterone and DHEA after the FST (corticosterone: F2,22 = 3.21, p = 0.05; DHEA: F2,22 = 3.53, p = 0.04), whereas non-contingent rats exhibited the highest concentrations of corticosterone after the FST ().
Figure 5. Fecal corticosterone and DHEA metabolite concentrations before and after 3 days of testing in the FST. (A) The FST increased fecal corticosterone (ANOVA, p < 0.001) and DHEA (p < 0.001). (B) Training and coping style interacted (ANOVA, p = 0.045): after the FST, contingent variable copers and non-contingent variable copers had lowest and highest fecal corticosterone. *p < 0.05 vs. other groups, Tukey. Groups: contingent variable, n = 4; non-contingent variable, n = 5; contingent active, n = 5; non-contingent active, n = 4; contingent passive, n = 5; non-contingent passive, n = 5.
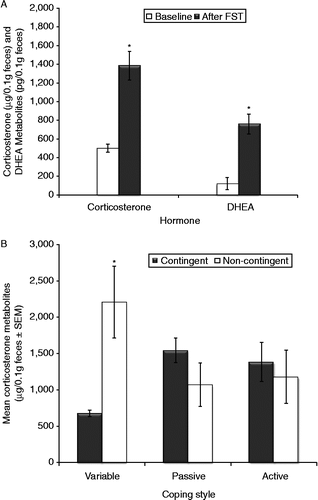
When fecal corticosterone concentrations were analyzed in conjunction with DHEA concentrations (i.e. DHEA/corticosterone ratio) after the FST, significant effects were observed for coping style (F2,22 = 4.36, p = 0.025), training group (F1,22 = 4.96, p = 0.036), and the interaction between coping style and training (F2,22 = 7.17, p < 0.01). Although variable copers secreted more DHEA than the other coping groups relative to corticosterone during the FST, contingent rats had higher concentrations of DHEA than non-contingent rats relative to corticosterone (). Post hoc tests revealed that the highest DHEA/corticosterone ratios were observed in contingent variable copers.
Figure 6. DHEA and corticosterone metabolite concentrations before and after 3 days of testing in the FST. Significant effects were for coping style (ANOVA, p = 0.025), training group (ANOVA, p = 0.036), and interaction between coping style and training (ANOVA, p < 0.01). *p < 0.05 vs. other groups, Tukey. Contingent variable, n = 4; non-contingent variable, n = 5; contingent active, n = 5; non-contingent active, n = 4; contingent passive, n = 5; non-contingent passive, n = 5.
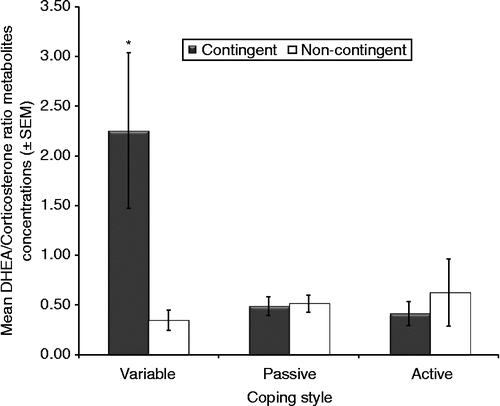
Neuroimmunoreactivity responses
Significant effects of coping strategy were observed in the amount of NPY-immunoreactive tissue in the hippocampus (CA1: F2,22 = 7.01, p < 0.01; CA3: F2,22 = 3.55, p = 0.045). In each case, variable copers exhibited higher levels of immunoreactive tissue than both active and passive coping groups; furthermore, active copers had higher levels than passive copers (). Conversely, the type of training had no significant effect on NPY-ir hippocampal tissue (F1,22 = 0.21, ns); furthermore, no significant interactions between training and coping were observed (F2,22 = 0.86, ns). Finally, no significant effects of training or coping style were observed in the amygdala or DG brain areas.
Figure 7. Neuroimmunoreactivity for NPY in the CA1 and CA3 hippocampal sub-fields. Coping strategy altered NPY-immunoreactivity in the hippocampus. (A) CA1: ANOVA, p < 0.01; CA3:, p = 0.045. Variable copers (n = 9) had higher NPY levels than both active (n = 9) and passive (n = 10) coping groups; active copers (n = 9) had higher levels than passive copers (n = 10). Groups: contingent variable, n = 4; non-contingent variable, n = 5; contingent active, n = 5; non-contingent active, n = 4; contingent passive, n = 5; non-contingent passive, n = 5. (B) Representative photomicrographs showing NPY-immunoreactive cells in the hippocampus CA1 area in the variable and passive groups (scale bar: 100 μm).
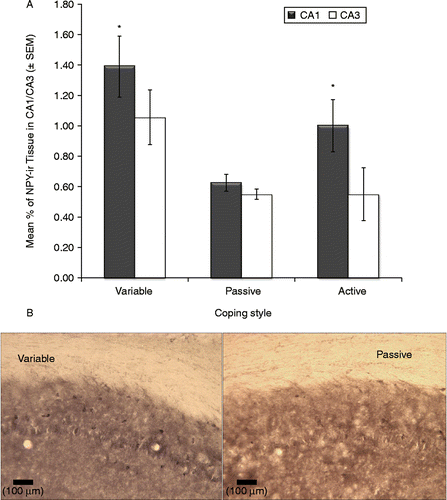
Multi-dimensional scaling
The MDS-generated associations among the variables are depicted in . Kruskal's stress index determined a stress value equal to 0.09, indicating a good fit between the dimensions and the mapped distances. The R2 value designated that 94.5% of the variance was explained by the data. Based on the two dimensions, the responses to the FST were divided into two major clusters, indicated in the figure by shaded circles within open ovals. The right oval represents rats that displayed higher physiological and neural activation for stress and fear (high NPY immunoreactivity of the amygdala and high fecal corticosterone concentrations). The second group, in the left oval, was characterized by the rats displaying high neural activity in the hippocampus (NPY immunoreactivity, including DG, CA1, and CA3). This latter group displayed higher levels of floating as well. Diving and DHEA activation were somewhat intermediate to both the groups. also depicts interesting associations between different behavioral and neuroendocrine responses: floating was associated with DHEA secretion and NPY-immunoreactivity in the CA1 and CA2 regions of the hippocampus, whereas diving was more closely associated with immunoreactivity in the DG region of the hippocampus, and the fear/stress responses mediated by the amygdala were characterized by high corticosterone levels.
Figure 8. MDS analysis model of independent associations among the variables. Kruskal's stress index = 0.09, indicating a good fit between the dimensions and the mapped distances. R2 value indicating 94.5% of the variance was explained by the data. The responses to the FST, indicated by differently shaded circles (VAR: variable copers), were divided into two major clusters, representing the two dimensions. The right oval represents rats showing greater physiological and neural activation for stress and fear (NPY in amygdala and fecal corticosterone [CORT]). The left oval represents rats showing high neural activity in DG and hippocampus (CA1 and CA3): this group floated more. Diving (DIVE) and DHEA activation were between the groups. Floating (FLOAT) was associated with DHEA secretion and NPY immunoreactivity in the hippocampus; diving was associated with NPY in the DG; fear/stress responses were related to amygdala NPY and corticosterone.
![Figure 8. MDS analysis model of independent associations among the variables. Kruskal's stress index = 0.09, indicating a good fit between the dimensions and the mapped distances. R2 value indicating 94.5% of the variance was explained by the data. The responses to the FST, indicated by differently shaded circles (VAR: variable copers), were divided into two major clusters, representing the two dimensions. The right oval represents rats showing greater physiological and neural activation for stress and fear (NPY in amygdala and fecal corticosterone [CORT]). The left oval represents rats showing high neural activity in DG and hippocampus (CA1 and CA3): this group floated more. Diving (DIVE) and DHEA activation were between the groups. Floating (FLOAT) was associated with DHEA secretion and NPY immunoreactivity in the hippocampus; diving was associated with NPY in the DG; fear/stress responses were related to amygdala NPY and corticosterone.](/cms/asset/568d5c61-f026-40ed-8111-314b915d7520/ists_a_623739_f0008_b.gif)
Focusing on individual differences by training, also illustrates how the contingent rats were characterized by a quite distinctive map of association in comparison to non-contingent rats. The former rats are clustered together with high neural activation coupled with the secretion of putative neuroprotectants such as DHEA, and also display higher frequencies of seemingly adaptive behaviors such as floating and diving. Non-contingent rats, in contrast, are clustered with high stress/fear responses, and they spent most of their time in the FST just swimming.
Discussion
In the current study, male rats profiled as active, passive, and variable copers were subsequently exposed to 4 weeks of contingent or non-contingent EBR training to determine the impact on neurobiological responses accompanying two behavioral challenge tasks. The results emphasize the importance of both behavioral training and predisposed coping styles on various components of resilience. In the PT, EBR contingent-trained rats and variable coping rats exhibited longer durations on task. Additionally, the main effect of coping style was found in the brain data; specifically, increased NPY-immunoreactivity was observed in the hippocampus of variable coping rats, regardless of training group.
Although both innate and acquired strategies exerted significant independent effects on responses exhibited during the appetitive challenge task (PT), these variables interacted in informative and unexpected ways during the stressful challenge task (FST). Specifically, variable copers in the contingent group exhibited longer floating durations than variable copers in the non-contingent group. Compared to the first day of floating, all rats exhibited longer durations of floating on the third day, indicating that as rats habituated to the stress of swimming, less frantic swimming strategies emerged and the decision to float occurred sooner than observed in the original swim test. Similarly, variable coping males in the contingent group exhibited more dive responses (a seemingly bold exploratory response) in the swim task than variable non-contingent males, with no differences observed between contingent and non-contingent active and passive coping groups. Comparable trends extended to the stress hormone data; contingent variable copers had lower fecal levels of corticosterone and higher DHEA/corticosterone ratios than non-contingent copers, differences were not observed in passive and active coping groups. Higher DHEA/corticosterone ratios have been associated with mental health/resilience (Charney Citation2004; Markopoulou et al. Citation2009). Although both floating and persistence on a task have been frequently associated with depression-like behavioral states (Porsolt et al. Citation1977; Cryan et al. Citation2002), the current results suggest that these behaviors can be alternatively explained as adaptive coping strategies. Indeed, floating could be interpreted as a mechanism to conserve energy in view of a possible escape, and persistence as a strategy to obtain a reward that is within reach. In this respect, it was particularly intriguing that the rate of floating increased from day 1 (when rats had no prior experience with the consequences of the FST) to day 3 (when rats had learned the session would end after a brief time period) specifically in those individuals that were able to better control their stress responses for both neural and hormonal activation.
As the data were assessed, the finding that variable copers possess adaptive responses (e.g. more efficient stress hormone responses, increased persistence, and more diving) is in agreement with previous research (Lambert Citation2006; Bardi et al. Citation2010). This finding, however, held true only in the contingent EBR-trained rats. When variable coping rats were placed in a non-contingent group, the variable copers appeared to have lost their adaptive advantage. Perhaps this effect was more salient in the current study where, in the non-contingent group, prized food rewards were regularly encountered with no specific effort required to obtain them. Although the variable copers appeared to respond maximally to contingency training, the removal of these behavioral consequences negated the adaptive advantage in the non-contingent group. This effect was unexpected as we predicted the lack of contingency to be a mere control for contingency training, not necessarily the powerful experimental manipulation observed in the current data.
The influence of EBR on the appetitive challenge task may have resulted from alterations in several factors implicated in building resilience against depression, including stressor controllability (Maier and Watkins Citation2005) and response–cost evaluations (Ishiwari et al. Citation2004; Salamone et al. Citation2009).
It is also important to note that the EBR training for the contingent group started at a relatively young age for these rats, a period in which they are still developing and thus are more sensitive to environmental challenges. Considering that the onset and treatment of adolescent depression are controversial, and studies on combined treatment including behavioral therapy have produced conflicting results (Dubicka et al. Citation2010), further studies assessing the effects of ERB training at different ages during the development would be beneficial.
The results of the current study indicate that the behavioral training can have a significant impact on appetitive challenge tasks, enhancing persistence and building resilience against the negative impact of stress (evidenced by training-induced changes in DHEA/corticosterone ratios). Yet, the data also indicate that certain coping and learning strategies influence the efficacy of behavioral training, namely EBR training. Resilience, defined as the utilization of active coping strategies such as problem solving to restrain the stress response, has been associated with an enhanced negative feedback system regulating stress neurochemicals, as well as a form of cognitive reappraisal of stressful events (Charney Citation2004; Feder et al. Citation2009). Focusing on EBR training, enhanced DHEA secretion may serve as a neurobiological mechanism sensitizing the negative feedback system for stress hormones. Furthermore, the display of increased floating following the initial swim task (more pronounced in contingency trained rats) suggests that these rats were “reappraising” the situation by adjusting their response to the same stimulus. Thus, the behavioral and neurobiological effects observed in the current study suggest that EBR is an appropriate animal model to further explore the emergence and maintenance of neurobiological aspects of resilience.
The MSD analysis provided further information about the relationships of variables involved in resilience and stress. A differential association with NPY was observed; specifically, amygdala immunoreactivity (although not significant in the initial ANOVA analysis) was associated with increased corticosterone secretion in the MDS analysis, while hippocampal NPY immunoreactivity was associated with resilience in the contingent-trained rats. Past research has indicated that transgenic rats overexpressing hippocampal NPY exhibit suppressed sensitivity to stress (Thorsell et al. Citation2000), a response observed in the contingent rats, especially the variable copers. If brain activation associated with responses as diverse as movement, reward, cognitive functions, and stressor controllability is important for building resilience against the emergence of depression (Tremblay et al. Citation2002; Tricomi et al. Citation2004; Watanabe Citation2007; Dimidjian and Davis Citation2009; Roshanaei-Moghaddam et al. Citation2009; Maier and Watkins Citation2010), it is necessary to utilize appropriate behavioral models that have the capacity to modulate many brain areas and accompanying neurochemicals in depression research (Lambert Citation2008). Additionally, animal models assessing flexible decision-making strategies based on the processing of information beyond current sensory input (Buckner and Carroll Citation2007; Schacter et al. Citation2008), including both predisposed coping strategies and relevant learned responses that are more flexible or variable in nature (Korte et al. Citation2005; Roitman et al. Citation2005), are important for investigations of resilience and effecting coping. As indicated in the current study, adaptive responses in the midst of challenging circumstances, a hallmark of emotional resilience, comprise a complex, integrative neurobiological response (Joels and Baram Citation2009). Coping style did not emerge as a possible dimension in the MDS analysis, and there are several interpretations for this result. From a statistical perspective, the number of rats in each group was probably too small to take into account the independent association of each of the six subgroups (contingent variable, non-contingent variable, contingent active, and so forth). In this case, the stronger of the two effects, between training and coping style, would be revealed by MDS. An alternative hypothesis is related to the interaction between EBR training and the original coping style. It is possible that EBR training could have significantly altered the original coping style. To test this hypothesis, further studies would need to repeat the back-test after the EBR training to see whether the original coping style was still valid.
In closing, the EBR training model provides a valuable animal model for the investigation of neurobiological aspects of resilience. Such a model also offers an opportunity to observe the roles of specific neurochemicals such as NPY and DHEA over the course of training and in response to various types of challenge tasks. Tracking these neurochemical factors, in addition to corticosterone and other relevant neurochemicals such as dopamine, in ethologically relevant animal models will allow researchers to more systematically evaluate neurobiological events accompanying the emergence of key diagnostic criteria in various MDs. Further research is necessary to more clearly elucidate the interaction between therapeutic approaches and individual differences observed in critical responses such as stress responsivity and coping strategies. A more detailed histological assessment of the subregions of the hippocampus involved in the regulation of the interaction between training and coping style, including the stratum oriens and stratum lucidum, would also be informative. Considering the complexity of the neurobiological factors associated with MDD, including active processes to gain control over stressors, it is becoming increasingly unlikely that the passive administration of a single chemical, outside of the context of relevant behavioral responses, represents the most effective treatment approach.
Acknowledgements
This research was funded by the Shapiro Undergraduate Research Fellowship program, the Macon and Joan Brock Professorship, and the Psychology Department at Randolph-Macon College.
Declaration of interest: The authors report no conflicts of interest. The authors alone are responsible for the content and writing of the paper.
References
- Amat J, Baratta MV, Paul E, Bland ST, Watkins LR, Saier SF. 2005. Medial prefrontal cortex determines how stressor controllability affects behavior and dorsal raphe nucleus. Nat Neurosci. 8:365–371.
- Bardi M, Hampton JH, Lambert KG. 2010. Fecal dehydroepiandrosterone (DHEA) immunoreactivity as a noninvasive index of circulating DHEA activity in young male laboratory rats. Comp Med. 52:460–465.
- Bardi M, Franssen CL, Hampton JE, Shea EA, Fanean A, Lambert KG. Paternal experience and stress responses in the California mouse (Peromyscus californicus). Comp Med. 2011a; 61:20–30.
- Bardi M, Koone T, Mewaldt S, O'Connor K. Behavioral and physiological correlates of stress related to examination performance in college chemistry students. Stress. 2011b; 14:557–566.
- Buckner RL, Carroll DC. 2007. Self-projection and the brain. Trends Cogn Sci. 11:49–57.
- Charney DS. 2004. Psychobiological mechanisms of resilience and vulnerability: Implications for successful adaptation to extreme stress. Am J Psychiatry. 161:195–216.
- Cryan JF, Markou A, Lucki I. 2002. Assessing antidepressant activity in rodents: Recent developments and future needs. Trends Pharmacol Sci. 23:238–245.
- Dalley JW, Cardinal RN, Robbins TW. 2004. Prefrontal executive and cognitive functions in rodents: Neural and neurochemical substrates. Neurosci Biobehav Rev. 28:771–784.
- Dichter GS, Felder JN, Smoski MJ. 2010. The effects of brief behavioral activation therapy for depression on cognitive control in affective contexts: An fMRI investigation. J Affect Disord. 126:236–244.
- Dimidjian S, Davis KJ. 2009. Newer variations of cognitive-behavioral therapy: Behavioral activation and mindfulness-based cognitive therapy. Curr Psychiatry Rep. 11:453–458.
- Dubicka B, Elvins R, Roberts C, Chick G, Wilkinson P, Goodyer IM. 2010. Combined treatment with cognitive-behavioural therapy in adolescent depression: Meta-analysis. Br J Psychiatry. 197:433–440.
- Duman RS. 2009. Neuronal damage and protection in the pathophysiology and treatment of psychiatric illness: Stress and depression. Dialogues Clin Neurosci. 11:239–255.
- Feder A, Nestler EJ, Charney DS. 2009. Psychobiology and molecular genetics of resilience. Nat Rev Neurosci. 10:446–457.
- Gelenberg AJ. 2010. The prevalence and impact of depression. J Clin Psychiatry. 71:e06.
- Geverink NA, Heetkamp MJ, Schouten WG, Wiegant VM, Schrama JW. 2004. Backtest type and housing condition of pigs influence energy metabolism. J Anim Sci. 82:1227–1233.
- Gutman AR, Yang Y, Ressler KJ, Davis M. 2008. The role of neuropeptide Y in the expression and extinction of fear-potentiated startle. J Neurosci. 28:12682–12690.
- Hawley DF, Bardi M, Everette AM, Higgins TJ, Tu KM, Kinsley CH, Lambert KG. 2010. Neurobiological constituents of active, passive, and variable coping strategies in rats: Integration of regional brain neuropeptide Y levels and cardiovascular responses. Stress. 13:172–183.
- Horwitz AV. 2010. How an age of anxiety became an age of depression. Milbank Q. 88:112–138.
- Ishiwari K, Weber SM, Mingote S, Correa M, Salamone JD. 2004. Accumbens dopamine and the regulation of effort in food-seeking behavior: Modulation of work output by different ratio or force requirements. Behav Brain Res. 151:83–91.
- Izawa S, Sugaya N, Shirotsuki K, Yamada KC, Ogawa N, Ouchi Y, Nagano Y, Suzuki K, Nomura S. 2008. Salivary dehydroepiandrosterone secretion in response to acute psychosocial stress and its correlations with biological and psychological changes. Biol Psychol. 79:294–298.
- Joels M, Baram TZ. 2009. The neuro-symphony of stress. Nat Rev Neurosci. 10:459–466.
- Kanter JW, Manos RC, Bowe WM, Baruch DE, Busch AM, Rusch LC. 2010. What is behavioral activation? A review of the empirical literature. Clin Psychol Rev. 30:608–620.
- Kelley AE. 1999. Functional specificity of ventral striatal compartments in appetitive behaviors. Ann N Y Acad Sci. 877:71–90 In: McGinty (Ed). Advancing from the ventral striatum to the extended amygdala: Implications for neuropsychiatry and drug abuse.
- Kessler RC, Berglund P, Demler O, Jin R, Koretz D, Merikangas KR, Rush AJ, Waters EE, Wang PS. 2010. The epidemiology of major depression disorder. JAMA. 289:3095–3105.
- Kirsch I. 2009. The Emperor's New Drugs. New York: Basic Books.
- Koolhaas JM, Korte SM, De Boer SF, Van der Begt BJ, Van Reenen CG, Hopsger H, De Jong IC, Ruis MAW, Blokhuis HJ. 1999. Coping styles in animals: Current status in behavioral and stress-physiology. Neurosci Biobehav Rev. 23:925–935.
- Korte SM, Koolhaas JM, Wingfield JC, McEwen BS. 2005. The Darwinian concept of stress: Benefits of allostasis and costs of allostatic load and the trade-offs in health and disease. Neurosci Biobehav Rev. 29:3–38.
- Lambert KG. 2006. Rising rates of depression in today's society: Consideration of the roles of effort-based rewards and enhanced resilience in day-to-day functioning. Neurosci Biobehav Rev. 30:497–510.
- Lambert KG. 2008. Lifting depression. New York: Basic Books.
- Lambert KG, Tu K, Everette A, Love G, McNamara I, Bardi M, Kinsley CH. 2006. Explorations of coping strategies, learned persistence, and resilience in Long-Evans rats: Innate versus acquired characteristics. Ann N Y Acad Sci. 1094:319–324.
- Lindell SG, Schwandt ML, Sun H, Sparenborg JD, Björk K, Kasckow JW, Sommer WH, Goldman D, Higley JD, Suomi SJ, Heilig M, Barr CS. 2010. Functional NPY variation as a factor in stress resilience and alcohol consumption in rhesus macaques. Arch Gen Psychiatry. 67:423–431.
- Maier SF, Watkins LR. 2005. Stressor controllability and learned helplessness: The roles of the dorsal raphe nucleus, serotonin, and corticotrophin-releasing factor. Neurosci Biobehav Rev. 29:829–841.
- Maier SF, Watkins LR. 2010. Role of the medial prefrontal cortex in coping and resilience. Brain Res. 1355:52–60.
- Maninger N, Wolkowitz OM, Reus VI, Epel ES, Mellon SH. 2009. Neurobiological and neuropsychiatric effects of dehydroepiandrosterone (DHEA) and DHEA sulfate (DHEAS). Front Neuroendocrinol. 30:65–91.
- Manly BFJ. 1994. Multivariate statistical methods. London: Chapman and Hall.
- Markopoulou K, Papadopoulos A, Juruena MF, Poon L, Pariante CM, Cleare AJ. 2009. The ratio of cortisol/DHEA in treatment resistant depression. Psychoneuroendocrinology. 34:19–26.
- Mathew SJ, Price RB, Charney DS. 2008. Recent advances in the neurobiology of anxiety disorders: Implications for novel therapeutics. Am J Med Genet C Semin Med Genet. 148C:89–98.
- Morgan CA, Southwick S, Hazlett G, Rasmusson A, Hoyt G, Zimolo Z, Charney D. 2004. Relationships among plasma dehydroepiandrosterone sulfate and cortisol levels, symptoms of dissociation, and objective performance in humans exposed to acute stress. Arch Gen Psychiatry. 61:819–825.
- Neill DB, Fenton H, Justice JB. 2002. Increase in accumbal dopaminergic transmission correlates with response cost not reward of hypothalamic stimulation. Behav Brain Res. 137:129–138.
- Oitzl MS, Champagne DL, van der Veen R, de Kloet ER. 2010. Brain development under stress: Hypotheses of glucocorticoid actions revisited. Neurosci Biobehav Rev. 34:853–866.
- Olfson M, Marcus SC. 2009. National patterns in antidepressant medication treatment. Arch Gen Psychiatry. 66:848–856.
- Oortmerssen GA, Busser J. 1989. Studies in wild house mice III: Disruptive selection on aggression as a possible force in evolution. In: Brain PF, Mainardi D, Parmigiani S. editors. House mouse aggression: A model of understanding the evolution of social behaviorHarwood Academic Publishers87–117.
- Pinnock SB, Lazic SE, Wong HT, Wong IHW, Herbert J. 2009. Synergistic effects of dehydroepiandrosterone and fluoxetine on proliferation of progenitor cells in the dentate gyrus of the adult male rat. Neuroscience. 158:1644–1651.
- Porsolt R, Le Pichon M, Jalfre M. 1977. Depression: New animal model sensitive to antidepressant treatments. Nature. 266:730–732.
- Roitman MF, Wheeler RA, Carelli RM. 2005. Nucleus accumbens neurons are innately tuned for rewarding and aversive taste stimuli, encode their predictors, and are linked to motor output. Neuron. 45:587–597.
- Roshanaei-Moghaddam B, Katon WJ, Russo J. 2009. The longitudinal effects of depression on physical activity. Gen Hosp Psychiatry. 3:306–315.
- Rotzinger S, Lovejoy DA, Tan LA. 2010. Behavioral effects of neuropeptides in rodent models of depression and anxiety. Peptides. 31:736–756.
- Sah R, Ekhator NN, Strawn JR, Sallee FR, Baker DG, Horn PS, Geracioti TDJr. 2009. Low cerebrospinal fluid neuropeptide Y concentrations in posttraumatic stress disorder. Biol Psychiatry. 66:705–707.
- Sajdyk TJ, Johnson PL, Leitermann RJ, Fitz SD, Dietrich A, Morin M, Gehlert DR, Urban JH, Shekhar A. 2008. Neuropeptide Y in the amygdala induces long-term resilience to stress-induced reductions in social responses but not hypothalamic–adrenal–pituitary axis activity or hyperthermia. J Neurosci. 28:893–903.
- Salamone JD, Correa M, Farrar AM, Nunes EJ, Pardo M. 2009. Dopamine, behavioral economics, and effort. Front Behav Neurosci. 3:13.
- Schacter DL, Addis DR, Buckner RL. 2008. Episodic simulation of future events: Concepts, data, and applications. Ann N Y Acad Sci. 1124:39–60.
- Thorsell A. 2010. Brain neuropeptide Y and corticotropin-releasing hormone in mediating stress and anxiety. Exp Biol Med. 235:1163–1167.
- Thorsell A, Michalkiewicz M, Dumont Y, Quirion R, Caberlotto L, Rimondini R, Mathe AA, Heilig M. 2000. Behavioral insensitivity to restraint stress, absent fear suppression of behavior and impaired spatial learning in transgenic rats with hippocampal neuropeptide Y overexpression. Proc Natl Acad Sci U S A. 97:12852–12857.
- Tremblay LK, Naranjo CA, Cardenas L, Herrmann N, Busto UE. 2002. Probing brain reward system function in major depressive disorder. Arch Gen Psychol. 59:409–416.
- Tricomi EM, Delgado MR, Fiez JA. 2004. Modulation of caudate activity by action contingency. Neuron. 41:281–292.
- Ulmann L, Rodeau JL, Danoux L, Contet-Audonneau JL, Pauly G, Schlichter R. 2009. Dehydroepiandrosterone and neurotrophins favor axonal growth in a sensory neuron-keratinocyte coculture model. Neuroscience. 159:514–525.
- Van Erp-an der Kooij E, Kuijpers HH, Schrama JW, Ekkel ED, Tielen MJM. 2000. Individual behavioural characteristics in pigs and their impact on production. Appl Anim Behav Sci. 66:171–185.
- Xu J, Li M, Shen P. 2010. A G-protein-coupled neuropeptide Y-like receptor suppresses behavioral and sensory response to multiple stressful stimuli in Drosophila. J Neurosci. 30:2504–2512.
- Watanabe M. 2007. Role of anticipated reward in cognitive behavioral control. Curr Opin Neurobiol. 17:213–219.
- Wemm S, Koone T, Blough ER, Mewaldt S, Bardi M. 2010. The role of DHEA in relation to problem solving and academic performance. Biol Psychol. 85:53–61.
- Zahm DS. 1999. Functional-anatomical. Ann N Y Acad Sci. 877:113–138 In: McGinty, editor. Advancing from the ventral striatum to the extended amygdala: Implications for neuropsychiatry and drug abuse.
- Zender R, Olshansky E. 2009. Women's mental health: Depression and anxiety. Nurs Clin North Am. 44:355–364.