Abstract
Adolescence represents a uniquely sensitive developmental stage in the transition from childhood to adulthood. During this transition, neuronal circuits are particularly susceptible to modification by experience. In addition, adolescence is a stage in which the incidence of anxiety disorders peaks in humans and over 75% of adults with fear-related disorders met diagnostic criteria as children and adolescents. While postnatal critical periods of plasticity for primary sensory processes, such as in the visual system are well established, less is known about potential critical or sensitive periods for fear learning and memory. Here, we review the non-linear developmental aspects of fear learning and memory during a transition period into and out of adolescence. We also review the literature on the non-linear development of GABAergic neurotransmission, a key regulator of critical period plasticity. We provide a model that may inform improved treatment strategies for children and adolescents with fear-related disorders.
Introduction
Recent advances have uncovered a wealth of knowledge about the neural circuitry involved in anxiety and stress related disorders. As the majority of research has focused on characterizing inappropriate and exaggerated fear responses in the mature adult brain, current clinical treatments have been implemented based on their efficacy in a mature neural framework. There is a comparative lack of knowledge about the development of fear circuitry that may limit successful treatment outcomes in children and adolescents (Liberman et al., Citation2006). In addition, early life environmental and physiological stressors can alter the development of this circuitry, highlighting the importance of understanding fear circuitry from early life, onward through adolescence, and into adulthood (Eiland & Romeo, Citation2012). While discrete postnatal windows or “critical periods” of plasticity for primary sensory learning, such as in the visual system, have been established in both animal models and humans (Hensch, Citation2004, Citation2005b), less is known of the about potential critical or sensitive periods for fear learning and memory. In this review, we present recent findings indicating that the circuitry underlying adaptive fear responses and anxiety has a dynamic nonlinear developmental trajectory, particularly during the transitions into and out of adolescence. We suggest that understanding the unique attributes of fear regulation during adolescence can provide insights into more effective targeted treatment strategies for children and adolescents with anxiety and stress related disorders.
Adolescence is a highly conserved developmental stage, in which mammals of many species transition from parental dependence to the sexual maturity and independence of adulthood (Spear, Citation2004). Adolescent animals differ markedly from younger and older animals in varied aspects of behavior (Spear & Brake, Citation1983). The behavioral changes across adolescence are mirrored by the dynamic non-linear maturation of multiple central nervous system (CNS) components. Gray matter density changes substantially with the onset of adolescence, reflecting the pruning of the redundant synapses of early childhood and increases in myelination (Gogtay et al., Citation2004; Gogtay et al., Citation2006; Giedd et al., Citation1999; Huttenlocher, Citation1984; Paus et al., Citation2008; Rakic et al., Citation1986; Yakovlev & Lecours, Citation1967). Meanwhile, major refinements of excitatory synapses and inhibitory circuits take place, including the pruning of excitatory glutamatergic synapses and subsequent proliferation and maturation of inhibitory GABAergic circuits and inputs (Anderson et al., Citation1995; Hashimoto et al., Citation2009; Huttenlocher & Dabholkar, Citation1997; Rakic et al., Citation1986).
Critical period plasticity
The transition into and out of adolescence also represents a critical, or sensitive, period, in which adverse events can alter normative developmental trajectories and predispose to behavioral and psychological disorders (Eiland & Romeo, Citation2012). This window of sensitivity may explain why adolescence is a developmental period characterized by increased incidence of psychopathologies including anxiety disorders and depression (Angold et al., Citation1999; Kessler et al., Citation2005; Kim-Cohen et al., 2003; Merikangas et al., 2010, 2011; Newman et al., Citation1996; Pine et al., Citation1998; Pollack et al., 1996). Functional impairment or aberrant development of the GABAergic system has been associated with the onset of these disorders (Kalueff & Nutt, Citation2007). Excitatory-inhibitory circuit maturation involves exceptionally precise calibration of excitatory-inhibitory balance. Lesions during this critical developmental period in adolescence may re-route pathways or developmental processes in specific brain regions, and furthermore, the effects of such an insult may not become evident until the fine-tuning of excitatory-inhibitory inputs has reached its mature balance (Bavelier et al., Citation2010; Insel, Citation2010). Disruption of excitatory/inhibitory balance during critical periods of early development is in fact one of the predominating explanations for the onset and complexity of Autism Spectrum Disorders (Gogolla et al., Citation2009b; Rubenstein, Citation2010; Rubenstein & Merzenich, Citation2003). Several lines of evidence have also implicated the involvement of disrupted excitatory/inhibitory balance in the etiology of schizophrenia (Lewis et al., Citation2005).
Critical periods of development are defined as specific developmental windows, during which “brain circuits that subserve a given function are particularly receptive to acquiring certain kinds of information or even need that instructive signal for their continued normal development” (Hensch, Citation2004). Further, critical periods represent an extreme form of general sensitivity, when neuronal properties are particularly susceptible to modification by experience. The vast majority of knowledge surrounding the existence of critical periods in development has stemmed from research in the visual system, in which monocular deprivation during a confined postnatal period results in a permanent loss of visual acuity in the deprived eye despite no physical damage to the eye itself (Hensch, Citation2005a). Monocular deprivation outside of this window does not result in loss of visual acuity in the deprived eye. Closure of the critical period for plasticity in the visual system is dependent on the inhibitory neurotransmitter, GABA (γ-aminobutyric acid) through late-developing GABAergic inhibitory connections impinging on pre-existent excitatory circuits. Functional enhancement of GABAergic transmission during the developmental window of visual plasticity triggers expansion of ocular dominance column width (Hensch, Citation2005a). In mice that lack the GABA synthesizing enzyme GAD65, the visual cortex remains in an immature, pre-critical period state throughout life. Enhancement of GABA transmission at any time point in GAD65 knockout mice with benzodiazepine treatment can trigger critical period onset (Fagiolini & Hensch, Citation2000). Closure of developmental critical periods appears to be related to maturation of the extracellular matrix surrounding fast-spiking GABAergic interneurons that express parvalbumin (PV). In particular, the formation of perineuronal nets (PNNs), an organized form of chondroitin sulfate proteoglycan-containing extracellular matrix, initiates critical period closure in the visual cortex (Berardi et al., Citation2003; Pizzorusso et al., Citation2002). Experimental degradation of PNNs by the enzyme chondroitinase ABC in adults reinitiates ocular dominance plasticity and restores visual acuity (Pizzorusso, Citation2009; Pizzorusso et al., Citation2002). Thus, the formation of PNNs indicates the initiation of one type of molecular “brake” on critical periods (Bavelier et al., Citation2010).
Recently, similar developmental plasticity mechanisms have been shown to influence emotional learning processes during the transition into adolescence. In the basolateral amygdala, the rapid increase formation of PNNs in pre-adolescence marks the end of a developmental period during which fear memories can be erased by extinction (Gogolla et al., Citation2009a). Ablation of amygdala PNNs with chondroitinase ABC in adulthood led to a switch back to a juvenile-like state in which extinction training led to permanent attenuation of the fear memories (Gogolla et al., Citation2009a). The possible mechanisms by which degradation of PNNs enables fear memory erasure are two-fold. PNNs may prevent fear memory erasure by rendering potentiated synapses resistant to long-term potentiation reversal, or these observed effects might involve changes in local GABA-mediated inhibition. The latter mechanism is plausible due to the fact that PNNs primarily forms around PV-positive GABAergic interneurons as well as the fact that GABAergic neurotransmission mediates several forms of BLA synaptic plasticity (Gogolla et al., Citation2009a). The role of GABAergic transmission in critical periods combined with the role of PNNs in fear regulation across development warrants a closer look at the developmental trajectory of the GABAergic system and its potential role in learning and memory.
Non-linear maturation of the gabaergic system
GABA is an inhibitory neurotransmitter that acts via ionotropic GABAA and metabotropic GABAB receptors in the mammalian CNS, mediating a variety of functions including modulation of neuronal excitability, long-term potentiation, and generation of post-synaptic potentials (Chang et al., Citation2003; Kalueff & Nutt, Citation2007; Korpi et al., Citation2002; Mendez & Bacci, Citation2011). Both GABAA and GABAB receptors are known to play roles in various aspects of cognition including working memory and emotional regulation (Cryan & Kaupmann, Citation2005; Kalueff & Nutt, Citation2007; Michels et al., Citation2012). Considering its complex contribution to normal neuronal function, the influence of GABA on the information flow between various brain regions is speculated to involve more than simple inhibitory influence. In early postnatal development, GABAA receptors are known to switch from mediating excitatory depolarizing membrane responses to inhibitory hyperpolarizing membrane responses (Mueller et al., Citation1984). In this early developmental time frame, GABA receptors and synapses are functional/operative even before glutamatergic synapses in many brain structures (Ben-Ari et al., Citation2004). GABAergic interneurons exert control in the developing hippocampus, forming functional synaptic networks and maturing well before the pyramidal neurons (Ben-Ari et al., Citation2004). This early excitatory/inhibitory switch is well characterized, but later development of the GABAergic system that occurs well into late adolescence is less understood. Much of the critical fine-tuning of GABAergic system components responsible for neuronal plasticity and activity levels takes place in adolescence and early adulthood (Kilb, Citation2012). The maturing elements of the GABAergic system across development are many-fold, and each contributes to the overall fine-tuning of GABAergic inhibitory tone. GABAergic synapse proliferation modulates GABAergic tone as much as the modulation of GABA receptor distribution, transporter distribution, and metabolic enzyme production. Each element has a distinct development trajectory and can influence changes in the homeostatic balance of GABAergic tone with implications for developmental stage-specific alterations in plasticity and behavior.
The postnatal maturation of the various GABA transporters is protracted, altering the behavior of GABAergic interneurons well into adolescence. The levels of the vesicular GABA transporter, which accumulates GABA in synaptic vesicles, remains relatively constant across development; however, other families of GABA transporters are strongly developmentally regulated (Pinto et al., Citation2010). GABA transporter GAT-1, involved in clearing GABA from the synaptic cleft and increasing intracellular GABA availability, has a marked non-linear profile (Borden, Citation1996). GAT-1 mRNA expression in rats appears low at birth and peaks between postnatal day 21 (P21) and P30 with levels 25–40% above adult levels (Xia et al., Citation1993). The subcellular localization of GAT-1 also varies in a non-linear manner throughout development. GAT-1 is transiently localized to the soma in rat hippocampus and neocortex between P5 and P30 suggesting that GAT-1 expression may play a key role in the functional maturation of the GABAergic system (Yan et al., Citation1997).
GABAA receptor subunits also undergo major brain region-specific developmental changes in adolescence. Whereas α2, α3 and α5 receptors are already present in the prenatal rat cortex, α1 subunit mRNA and protein expression does not begin until after P7 (Fritschy et al., Citation1994; Laurie et al., Citation1992). The expression α1 subunit mRNA continues to rise, peaking around P28 then steadily declining to reach adult levels at P60 (Gambarana et al., Citation1991). Meanwhile, α2 subunits levels remain relatively steady and both α5 subunit and β-subunit levels are appreciably downregulated between approximately P30 and P60 (Gambarana et al., Citation1991; Laurie et al., Citation1992). The sharpening of inhibitory transmission across development is thought to be attributable in large part to these changes in GABAA receptor subunit distribution (Okaty et al., Citation2009).
The expression of genes coding for proteins that affect membrane and synaptic properties (K+ and Ca2+ channels) show distinct changes between the P25 and P40 in GABAergic interneurons (Okaty et al., Citation2009). Inhibitory interneurons undergo major transcriptional and electrophysiological changes between P7 and P40 in the mouse (Okaty et al., Citation2009). The two pore K+ leak channels Kcnc1 and Kcnc2 undergo marked upregulation between P10 and P25 while the small conductance Ca2+-activated K+ channel Kcnn2 undergoes drastic downregulation, contributing to major differences between the intrinsic membrane properties of immature and adult inhibitory interneurons. These transcriptional changes lead to a narrowing of action potentials and emergence of high-frequency firing, among other changes.
Overall excitatory/inhibitory circuit maturation takes place in a non-linear brain-region specific manner, with the hippocampus the first to mature and the prefrontal cortex (PFC) the last to mature (Insel, Citation2010; Kilb, Citation2012). Studies focusing on fear circuitry across development suggest that surges in the proliferation of inhibitory GABAergic circuits in higher-order association areas such as the PFC occur only after the lower-order sensorimotor regions resulting in transient “natural lesions” wherein the functional connectivity from projections in those brain regions are temporarily blunted (McCallum et al., Citation2010; Hare et al., Citation2008; Pattwell et al., Citation2012). Fear learning and memory circuits integrate competing inputs from various brain regions to determine output. As a result, blunted functional connectivity from still-maturing projections across development can influence the expression of fear responses. In adolescence in particular, transient disruptions in prototypical functional connectivity due to normal maturation of the fear circuitry may result in major changes in fear expression.
Fear learning and the GABAergic system
Fear learning is a highly adaptive process that allows organisms to respond appropriately to cues associated with danger. The neural circuitry of fear learning and memory is highly conserved between rodents and humans and has allowed for in depth characterizations of the behavioral and molecular processes associated with fear learning and memory using Pavlovian-based fear conditioning paradigms (LeDoux, Citation2000; Maren, Citation2001). Pavlovian principles dictate that an initially emotionally neutral conditioned stimulus (CS), can acquire negative affective properties on repeated pairings with an adverse unconditioned stimulus (US) (LeDoux, Citation2000). This CS-US relation is quickly learned and consolidated such that innate physiological and behavioral responses are elicited with later presentations of the CS alone (LeDoux, Citation2000). Such is the case in rodents when a tone CS followed by an electric shock US is presented repeatedly, and a defensive response such as freezing behavior is elicited by the tone alone. In human studies, skin conductance response (SCR) can be measured to assess prototypical physiological fear responses (Monk et al., Citation2003, Soliman et al., Citation2010).
For the CS to take on the aversive properties of the US and subsequently induce a fear response several sequential events must occur. The CS-US pairing must be learned, via a process referred to as acquisition. After this pairing, the association must be consolidated, so that it may be accessed at later time points. Lastly, when the CS is encountered again, the subject must be able to retrieve the associated memory in order for the appropriate response to be made (Phelps & LeDoux, Citation2005). When cues previously associated with a threat are repeatedly presented in the absence of the initial aversive event, previously dangerous cues are re-assessed as safe and fear responses are reduced in a process of extinction learning. The reduction of fear responding through fear extinction learning is at the heart of clinical exposure therapies applied when fear responses become exaggerated and inappropriate, such as in the case of post-traumatic stress disorder (Rothbaum & Davis, Citation2003). Extinction learning is not an undoing of the acquired fear association but rather an active process through which the newly learned association of the CS with safety competes with the initial CS-US pairing (Sotres-Bayon & Quirk, Citation2010).
The amygdala lies at the crux of fear acquisition machinery (Fanselow, Citation1994; Maren et al., Citation1996). In standard auditory cued fear conditioning, projections from the prelimbic cortex (PL) and thalamic nuclei receive sensory inputs and converge on the lateral nucleus of the amygdala (LA) simultaneously. Collectively the basal (BA) and lateral (LA) nuclei comprise the BLA, the primary interface involved in fear learning and acquisition. After the relevant sensory information is integrated by the BLA, information is relayed to the central nucleus (CE). The CE acts as the amygdala’s secondary interface, serving to elicit fear responses through various downstream projections, including those to hypothalamic and brainstem nuclei to engage autonomic responses () (Maren, 2011). In terms of the cytoarchitecture of the amygdala, the BLA contains primarily glutamatergic projection neurons with fewer local GABA interneurons. In the more medial CE, the majority of neurons are GABAergic, with medium spiny neuronal morphology (Ehrlich et al., Citation2009). Finally, there are clusters of GABAergic interneurons bordering the BLA called intercalated cells (ITC) that mediate interactions between BLA and CE. ITCs receive glutamatergic projections from the ventromedial (vm) PFC (Pare & Duvarci, Citation2012; Pare & Smith, Citation1993). This projection is activated by the repeated CS-only presentations during fear extinction learning leading to reduced responses in the CE and decreased fear-related behavior (Milad & Quirk, Citation2012; Phelps et al., Citation2004).
Figure 1. Developmental neural circuitry of fear expression and extinction. Representation of the adult neural circuitry implicated in (A) fear expression and (B) fear extinction.
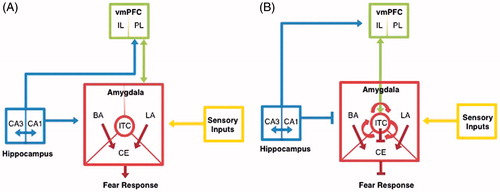
During fear acquisition, projections from the hippocampus (specifically the CA1 region) also supply information to the BA providing contextual information about the surrounding environment (Bouton et al., Citation2006; Phelps & LeDoux, Citation2005). Hippocampal-BA integration of contextual information has a major influence on downstream CE activity and subsequent downstream responses (Maren, Citation2001; Pattwell et al., 2011).
In the adult CNS, GABA transmission plays integral and complex roles in regulating pyramidal cell excitability and responsiveness within the multiple microcircuits in the amygdala, as well as other regions implicated in the fear circuit. The precise impact of the inhibitory circuits on fear learning and memory has recently been extensively reviewed (Ehrlich et al., Citation2009; Pare & Duvarci, Citation2012). In contrast, less has been established on the role of GABA in the developing fear circuitry. With the non-linear development of the GABAergic system, and the importance of GABA transmission in fear learning and memory, it is plausible that developmental differences in fear learning and memory may be mediated by these alterations in the GABA system. In particular, it is possible that during adolescence connections between the amygdala and brain regions such as the hippocampus and PFC are still developing (Bouwmeester et al., 2002a,b; Cunningham et al., Citation2002) and therefore that the forms of synaptic plasticity and excitatory and inhibitory balance in the amygdala may differ markedly from those in adulthood.
Sensitive periods for fear learning and memory
Cued fear and cued extinction in early development
Infant rats are known to display a form of rapid and spontaneous forgetting known as infantile amnesia (Campbell & Campbell, Citation1962). The neurobiological basis of spontaneous forgetting in the infant rat has been linked to increased GABAergic neurotransmission in early development (Kim et al., Citation2006; Kim & Richardson, 2007b). When tested shortly after fear conditioning, infant rats express fear memory retention comparable to adults; however, when tested after a delay of 7 d, the level of retention in infant rats is dramatically reduced, as measured by reduced freezing behavior to the CS (Kim et al., Citation2006; Tang et al., Citation2007). Notably, the forgotten memory can be recovered by an injection of the GABA receptor partial inverse agonist FG7142 prior to retention testing (Tang et al., Citation2007). These results suggest that increased GABA neurotransmission may suppress the retrieval of fear memories under normal circumstances in the infant rat.
Further support for causal link between increased GABA neurotransmission and infantile amnesia is provided by studies of the reactivation effect (Kim & Richardson, 2007b). Kim & Richardson (Citation2007) find that under normal conditions, a reminder (an abbreviated form of the training session) administered 24 h prior to retention testing reactivates a previously forgotten memory by decreasing GABAergic inhibition (Kim & Richardson, 2007b). Indeed, fear conditioned infant rats given a post-reminder injection of midazolam (a benzodiazepine that increases GABAergic neurotransmission) exhibit a diminished reactivation effect compared to saline-treated controls (Kim & Richardson, 2007b). The finding that a reminder treatment reactivates a forgotten memory in infant rats and that midazolam blocks its effects further supports the hypothesis that infantile amnesia results from a retrieval deficit due to activation of the GABAergic system early in development (Kim & Richardson, 2007b).
Fear extinction learning is a complex active learning process, wherein a second, competing association is made that inhibits the expression of the original paired US-CS fear memory (Bouton, Citation2002). As such, the expression of extinguished fear memories is often susceptible to renewal. In renewal, subjects exposed to the CS, in a novel context distinct from that in which extinction occurred, can experience a recovery of fear response. Previous studies examining the extinction of conditioned fear in P16 and P23 rats find that when rats are tested in a novel context distinct from the extinction context, P16 rats fail to exhibit renewal whereas P23 rats exhibit a renewal of extinguished fear similar to that seen in adults (Kim & Richardson, 2007a). The absence of renewal in P16 rats suggests that the neural circuitry mediating extinction in P16 rats differs markedly from that of older rats, and as described above, may be due to developmental differences in GABAergic transmission within the fear neural circuitry.
During the expression of extinction memories, the medial PFC (mPFC) is thought to inhibit the amygdala by activating GABAergic interneurons (Hobin et al., Citation2003). By facilitating the retrieval of the original fear memory, injections of the GABA receptor partial inverse agonist FG7142 in adult rats prior to testing in the extinguished context are known to reverse extinction (Kim & Richardson, 2007a,b). Similar to adults, pre-test injections of FG7142 were found to lead to recovery of extinguished freezing response to the CS in P23 rats (Kim & Richardson, 2007b). In contrast, pre-test injections of FG7142 had no effect on P16 rats. Taken together, these results suggest that the hippocampus–mPFC–amygdala network is functionally or structurally different from that of older rats (Kim & Richardson, 2007b).
Cued fear and cued extinction in peri-adolescence
While cue fear learning emerges during early infancy (Sullivan et al., Citation2000) adult-like extinction fails to develop until much later. For example, inactivation of the mPFC fails to disrupt long-term extinction in pre-adolescent, P17 rats, suggesting a process more similar to forgetting. A fragile memory trace may underlie the behavioral decrement during an extinction paradigm in young animals. Indeed, adult-like mechanisms of extinction appear to occur around weaning as inactivation of the mPFC does not disrupt long-term extinction in pre-adolescent, P17 rats, but does disrupt this memory in P24 rats (Kim et al., Citation2009). Extinction training in these young age groups leads to increased levels of phosphorylated MAPK (pMAPK) in both the prelimbic (PL) and infralimbic (IL) cortices (both components of the mPFC), suggestive of non-specific, global mPFC activity, as opposed to the inverse pattern of IL/PL activity typically seen with successful adult extinction retention. A similar diffuse activation pattern, with less focal activity, has also been observed in the PFC of human children and adolescents during tasks requiring cognitive control (Durston et al., Citation2006). Developmental studies of innate fear regulation in rodents demonstrate that during innate fear, the mPFC of infant rats is neither active nor responsive, whereas in pre-adolescence the PL becomes active but does not yet regulate freezing behavior. Finally, in adolescence, the PL becomes functional and its activity corresponds to an appropriately expressed cued fear response (Chan et al., Citation2011). These developmentally altered roles of the mPFC are independent of amygdala activity, which suggests that mPFC neural circuitry develops enhanced capacities for fear regulation as an animal matures. In addition, injections of anterograde tracers placed into the BLA of developing rats show that amygdalo-cortical connectivity is late maturing, with fiber density reaching a plateau at approximately P45, thus confirming that maturation of this circuit continues into adolescence (Cunningham et al., Citation2002).
Recent investigations into the neural circuitry underlying fear extinction learning implicates hippocampal and amygdala inputs to PL in the gating of conditioned fear responses after extinction. Specfically, the hippocampus can modulate fear expression via projections to inhibitory interneurons in PL, whereas the amygdala can modulate fear via projections to excitatory pyramidal neurons in PL (Sotres-Bayon et al., Citation2012). While it is known that the PFC continues to develop throughout adolescence, a more precise understanding of a potential shift in excitatory/inhibitory connections within the PL, along with their incoming projections from other brain regions, remains to be investigated and may shed light on dynamic developmental learning processes.
Adolescence is a time of considerable change in the PFC in both humans and rodents (Sowell et al., Citation1999; van Eden et al., Citation1990). Gray matter density decreases dramatically in adolescence reflecting elimination and reorganization of PFC synaptic connections (Blakemore & Choudhury, Citation2006; Gogtay et al., Citation2004; Huttenlocher, Citation1984; Mrzljak et al., Citation1990). The decrease in mPFC volume results in dramatic changes in fear extinction learning during this dynamic developmental period. Anatomical studies of human cortical development have shown delayed maturation of the PFC relative to other lower-order sensorimotor regions both in terms of local decreases in gray matter density and increases in the myelination of fibers (Gogtay et al., Citation2004; Hare et al., Citation2008). Converging evidence from human and rodent studies suggest that immature top-down PFC regulation of subcortical structures such as the amygdala due to ongoing fine tuning of excitatory/inhibitory balance in the PFC coincide with diminished fear extinction learning (Pattwell et al., Citation2012).
Parallel human and rodent behavioral studies examined fear extinction learning in preadolescence, peri-adolescence, and adulthood in humans and mice, respectively (Pattwell et al., Citation2012). Conditioned fear responses in humans are characterized physiologically by changes in autonomic arousal and behavior. By monitoring changes in perspiration via skin SCR, prototypical physiological fear response during conditioned fear acquisition and fear memory extinction can be measured. Subjects across all ages learned to discriminate between the threat cue and the safety cue and fear as assessed by skin conductance measurements. While there was no difference in extinction learning between children and adults, peri-adolescents showed attenuated fear extinction learning compared to children and adults (Pattwell et al., Citation2012). Similar to human peri-adolescents, peri-adolescent mice also displayed attenuated fear extinction learning compared with their preadolescent and adult counterparts () (Pattwell et al., Citation2012).
Figure 2. Cued extinction learning across development in mice and humans. (A) Experimental paradigms for fear conditioning experiments in humans and mice. (B) Analysis of extinction indices reveals the effect of age group for humans, such that adolescents show attenuated fear extinction learning compared younger and older age groups. (C) A lack of extinction learning and retention of extinction memory is observed in adolescent mice (P29), as displayed by a significantly decreased differential extinction indices, (***p < 0.001) compared with older (P70) and younger (P23) ages. From (Pattwell et al., Citation2012) with permission.
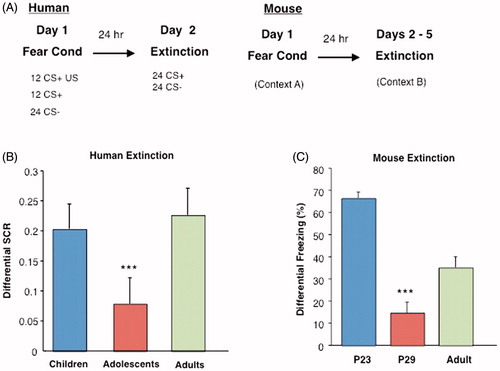
In a separate study, adolescent rats were also found to exhibit impaired extinction retention, compared to both their younger and older counterparts (McCallum et al., Citation2010). Notably, all adolescents displayed normal within-session fear extinction. Studies suggest that short-term extinction memory relies on the activation of L-type voltage gated calcium channels, whereas consolidation of extinction depends on NMDAR activation in the mPFC and in the BLA (Herry et al., Citation2006). This is corroborated by studies in rats with PFC lesions, which display normal learned fear expression and within-session rate of extinction but impaired expression of extinction memory when tested 24 hours post extinction training (Lebron et al., Citation2004; Quirk et al., Citation2000), highlighting the importance of excitatory/inhibitory balance within the PFC during extinction learning.
Within the vmPFC, the dorsally located PL is associated with production of conditioned-fear responses and expression of conditioned fear behaviors whereas the more ventral IL is associated with suppression of conditioned fear responses during successful extinction learning and upon recall of extinction memory (Burgos-Robles et al., Citation2009; Hefner et al., Citation2008; Knapska & Maren, Citation2009; Milad et al., Citation2004). Successful fear extinction learning in the IL of adult rodents can be predictably measured by immunohistochemical detection of the immediate early gene c-Fos (Santini et al., Citation2004). Immunohistochemical studies of c-Fos in both regions of the vmPFC of pre-adolescent and adult mice revealed markedly higher density c-Fos labeled cells in the IL of fear extinguished mice than in nonextinguished fear conditioned controls. There was no detectable change in the density of c-Fos labeling in peri-adolescent mice, suggesting that neural activity in the vmPFC of peri-adolescent mice differs from adult neural activity observed during fear extinction (Pattwell et al., Citation2012).
Electrophysiological recordings in vmPFC brain slices of mice after fear acquisition and fear extinction showed potentiation of PL excitatory synapses after fear acquisition in P23 and adult mice and subsequent depotentiation upon extinction, suggesting that PL excitatory synapses regulate fear expression. Simultaneously, potentiation of IL excitatory synapses in adult fear-extinguished mice is observed, suggesting an additional mechanism by which vmPFC excitatory synapses mediate extinction. These synaptic plasticity changes in the PL and IL observed in pre-adolescent and adult mice were absent in peri-adolescent mice suggesting that the vmPFC is not similarly engaged in the regulation of learned fear at this age (Pattwell et al., Citation2012). Given the delayed development of cortical GABAergic transmission, it is plausible that an imbalance in inhibitory synaptic transmission during peri-adolescence interferes with synaptic plasticity in mPFC (Pattwell et al., Citation2012).
Conclusions and future directions
The studies presented here review the dynamic nonlinear development of behavioral fear responses and the neural circuitry underlying fear learning and memory. Adolescence, in particular, represents a unique developmental stage for fear learning and memory, where cued fear extinction learning is markedly attenuated relative to earlier and later stages (McCallum et al., Citation2010; Pattwell et al., Citation2012). The divergence in adolescence from the prototypical fear responses associated with both younger and older ages underscores the importance of identifying the molecular underpinnings of this developmental period. The evidence presented here suggests that, at least in part, the dynamic changes in fear learning reflect and occur as a consequence of alterations in GABAergic neurotransmission. The GABAergic system undergoes substantial nonlinear postnatal maturation, which begins in childhood and continues until the end of adolescence. GABAergic synapses undergo profound changes during adolescence, becoming faster and more precise through alterations in membrane properties, enhanced GABA release, faster subunit dominance, and enhanced GABA uptake (Kilb, Citation2012). The transitions into and out of adolescence mark a sensitive period in fear learning when neuronal properties are primed to be particularly sensitive to modifications by experience. Enhanced GABA function is known to trigger the closure of the sensitive plastic states in the visual system, and could play a role in the closure of this sensitive period in fear extinction learning.
Adolescence represents a key period of exploration and independence and it should follow that certain danger clues remain resistant to extinction during adolescence, allowing the adolescent to remain both exploratory and cautious. That being said, the same features of adolescent brain development required for survival may also contribute to increased sensitivity to and treatment resistance of fear-related disorders such a PTSD or anxiety during this period. If evolutionarily conserved fear learning neural circuitry primes the adolescent to exhibit attenuated fear extinction, therapeutic methods relying on exposure therapy may benefit from considering new avenues of treatment, including those that take into account the influence of GABAergic neurotransmission. The development of treatments specific for this unique developmental time period is critical for the future success of adolescent fear and anxiety disorder treatments.
Declaration of interest
The authors report no conflicts of interest.
This work was supported by the DeWitt-Wallace Fund of the New York Community Trust (F.S.L.), Irma T. Hirschl/Monique Weill-Caulier Trust (I.D., F.S.L.), International Mental Health Research Organization (F.S.L.), Burroughs Wellcome Foundation (F.S.L.), Pritzker Consortium (F.S.L.), NARSAD (F.S.L.), and National Institutes of Health grants NS052819 (F.S.L.), MH088814 (F.S.L.), HD055177 (S.S.P.).
References
- Anderson SA, Classey JD, Conde F, Lund JS, Lewis DA. (1995). Synchronous development of pyramidal neuron dendritic spines and parvalbumin-immunoreactive chandelier neuron axon terminals in layer III of monkey prefrontal cortex. Neuroscience 67:7–22
- Angold A, Costello EJ, Erkanli A, Worthman CM. (1999). Pubertal changes in hormone levels and depression in girls. Psychol Med 29:1043–53
- Bavelier D, Levi DM, Li RW, Dan Y, Hensch TK. (2010). Removing brakes on adult brain plasticity: from molecular to behavioral interventions. J Neurosci 30:14964–71
- Ben-Ari Y, Khalilov I, Represa A, Gozlan H. (2004). Interneurons set the tune of developing networks. Trends Neurosci 27:422–7
- Berardi N, Pizzorusso T, Ratto GM, Maffei L. (2003). Molecular basis of plasticity in the visual cortex. Trends Neurosci 26:369–78
- Blakemore SJ, Choudhury S. (2006). Development of the adolescent brain: implications for executive function and social cognition. J Child Psychol Psychiatry 47:296–312
- Borden LA. (1996). GABA transporter heterogeneity: pharmacology and cellular localization. Neurochem Int 29:335–56
- Bouton ME. (2002). Context, ambiguity, and unlearning: sources of relapse after behavioral extinction. Biological Psychiatry 52:976–86
- Bouton ME, Westbrook RF, Corcoran KA, Maren S. (2006). Contextual and temporal modulation of extinction: behavioral and biological mechanisms. Biological Psychiatry 60:352–60
- Bouwmeester H, Smits K, Van Ree JM. (2002a). Neonatal development of projections to the basolateral amygdala from prefrontal and thalamic structures in rat. J Comparative Neurol 450:241–55
- Bouwmeester H, Wolterink G, van Ree JM. (2002b). Neonatal development of projections from the basolateral amygdala to prefrontal, striatal, and thalamic structures in the rat. J Comparative Neurol 442:239–49
- Burgos-Robles A, Vidal-Gonzalez I, Quirk GJ. (2009). Sustained conditioned responses in prelimbic prefrontal neurons are correlated with fear expression and extinction failure. J Neurosci 29:8474–82
- Campbell BA, Campbell EH. (1962). Retention and extinction of learned fear in infant and adult rats. J Comp Physiol Psychol 55:1–8
- Chan T, Kyere K, Davis BR, Shemyakin A, Kabitzke PA, Shair HN, Barr GA, et al. (2011). The role of the medial prefrontal cortex in innate fear regulation in infants, juveniles, and adolescents. J Neurosci 31:4991–9
- Chang L, Cloak CC, Ernst T. (2003). Magnetic resonance spectroscopy studies of GABA in neuropsychiatric disorders. J Clin Psychiatry 64:7–14
- Cryan JF, Kaupmann K. (2005). Don't worry 'B' happy!: a role for GABA(B) receptors in anxiety and depression. Trends Pharmacol Sci 26:36–43
- Cunningham MG, Bhattacharyya S, Benes FM. (2002). Amygdalo-cortical sprouting continues into early adulthood: implications for the development of normal and abnormal function during adolescence. J Comparative Neurol 453:116–30
- Durston S, Davidson MC, Tottenham N, Galvan A, Spicer J, Fossella JA, Casey BJ. (2006). A shift from diffuse to focal cortical activity with development. Dev Sci 9:1–8
- Ehrlich I, Humeau Y, Grenier F, Ciocchi S, Herry C, Luthi A. (2009). Amygdala inhibitory circuits and the control of fear memory. Neuron 62:757–71
- Eiland L, Romeo RD. (2012). Stress and the developing adolescent brain. Neuroscience. http://dx.doi.org/10.1016/j.neuroscience.2012.10.048
- Fagiolini M, Hensch TK. (2000). Inhibitory threshold for critical-period activation in primary visual cortex. Nature 404:183–6
- Fanselow MS. (1994). Neural organization of the defensive behavior system responsible for fear. Psychon B Rev 1:429–38
- Fritschy JM, Paysan J, Enna A, Mohler H. (1994). Switch in the expression of rat GABAA-receptor subtypes during postnatal development: an immunohistochemical study. J Neurosci 14:5302–24
- Gambarana C, Beattie CE, Rodriguez ZR, Siegel RE. (1991). Region-specific expression of messenger RNAs encoding GABAA receptor subunits in the developing rat brain. Neuroscience 45:423–32
- Giedd JN, Blumenthal J, Jeffries NO, Castellanos FX, Liu H, Zijdenbos A, Paus T, et al. (1999). Brain development during childhood and adolescence: a longitudinal MRI study. Nature Neurosci 2:861–3
- Gogolla N, Caroni P, Luthi A, Herry C. (2009a). Perineuronal nets protect fear memories from erasure. Science 325:1258–61
- Gogolla N, Leblanc JJ, Quast KB, Sudhof TC, Fagiolini M, Hensch TK. (2009b). Common circuit defect of excitatory-inhibitory balance in mouse models of autism. J Neurodevelop Disord 1:172–81
- Gogtay N, Giedd JN, Lusk L, Hayashi KM, Greenstein D, Vaituzis AC, Nugent TF 3rd, et al. (2004). Dynamic mapping of human cortical development during childhood through early adulthood. Proc Natl Acad Sci U S A 101:8174–9
- Gogtay N, Nugent TF 3rd, Herman DH, Ordonez A, Greenstein D, Hayashi KM, Clasen L, et al. (2006). Dynamic mapping of normal human hippocampal development. Hippocampus 16:664–72
- Hare TA, Tottenham N, Galvan A, Voss HU, Glover GH, Casey BJ. (2008). Biological substrates of emotional reactivity and regulation in adolescence during an emotional go-nogo task. Biol Psychiatry 63:927–34
- Hashimoto T, Nguyen QL, Rotaru D, Keenan T, Arion D, Beneyto M, Gonzalez-Burgos G, et al. (2009). Protracted developmental trajectories of GABAA receptor alpha1 and alpha2 subunit expression in primate prefrontal cortex. Biol Psychiatry 65:1015–23
- Hefner K, Whittle N, Juhasz J, Norcross M, Karlsson RM, Saksida LM, Bussey TJ, et al. (2008). Impaired fear extinction learning and cortico-amygdala circuit abnormalities in a common genetic mouse strain. J Neurosci 28:8074–85
- Hensch TK. (2004). Critical period regulation. Annu Rev Neurosci 27:549–79
- Hensch TK. (2005a). Critical period plasticity in local cortical circuits. Nat Rev Neurosci 6:877–88
- Hensch TK. (2005b). Critical period mechanisms in developing visual cortex. Curr Top Dev Biol 69:215–37
- Herry C, Trifilieff P, Micheau J, Luthi A, Mons N. (2006). Extinction of auditory fear conditioning requires MAPK/ERK activation in the basolateral amygdala. Eur J Neurosci 24:261–9
- Hobin JA, Goosens KA, Maren S. (2003). Context-dependent neuronal activity in the lateral amygdala represents fear memories after extinction. J Neurosci 23:8410–16
- Huttenlocher PR. (1984). Synapse elimination and plasticity in developing human cerebral cortex. Am J Ment Defic 88:488–96
- Huttenlocher PR, Dabholkar AS. (1997). Regional differences in synaptogenesis in human cerebral cortex. J Comparative Neurol 387:167–78
- Insel TR. (2010). Rethinking schizophrenia. Nature 468:187–93
- Kalueff AV, Nutt DJ. (2007). Role of GABA in anxiety and depression. Depress Anxiety 24:495–517
- Kessler RC, Demler O, Frank RG, Olfson M, Pincus HA, Walters EE, Wang P, et al. (2005). Prevalence and treatment of mental disorders, 1990 to 2003. N Engl J Med 352:2515–23
- Kilb W. (2012). Development of the GABAergic system from birth to adolescence. Neuroscientist 18:613–30
- Kim JH, Hamlin AS, Richardson R. (2009). Fear extinction across development: the involvement of the medial prefrontal cortex as assessed by temporary inactivation and immunohistochemistry. J Neurosci 29:10802–8
- Kim JH, McNally GP, Richardson R. (2006). Recovery of fear memories in rats: role of gamma-amino butyric acid (GABA) in infantile amnesia. Behav Neurosci 120:40–8
- Kim JH, Richardson R. (2007a). A developmental dissociation of context and GABA effects on extinguished fear in rats. Behav Neurosci 121:131–9
- Kim JH, Richardson R. (2007b). Immediate post-reminder injection of gamma-amino butyric acid (GABA) agonist midazolam attenuates reactivation of forgotten fear in the infant rat. Behav Neurosci 121:1328–32
- Kim-Cohen J, Caspi A, Moffitt TE, Harrington H, Milne BJ, Poulton R. (2003). Prior juvenile diagnoses in adults with mental disorder: developmental follow-back of a prospective-longitudinal cohort. Arch General Psychiatry 60:709–17
- Knapska E, Maren S. (2009). Reciprocal patterns of c-Fos expression in the medial prefrontal cortex and amygdala after extinction and renewal of conditioned fear. Learn Mem 16:486–93
- Korpi ER, Grunder G, Luddens H. (2002). Drug interactions at GABA(A) receptors. Prog Neurobiol 67:113–59
- Laurie DJ, Wisden W, Seeburg PH. (1992). The distribution of thirteen GABAA receptor subunit mRNAs in the rat brain. III. Embryonic and postnatal development. J Neurosci 12:4151–72
- Lebron K, Milad MR, Quirk GJ. (2004). Delayed recall of fear extinction in rats with lesions of ventral medial prefrontal cortex. Learn Mem 11:544–8
- LeDoux JE. (2000). Emotion circuits in the brain. Annu Rev Neurosci 23:155–84
- Lewis DA, Hashimoto T, Volk DW. (2005). Cortical inhibitory neurons and schizophrenia. Nat Rev Neurosci 6:312–24
- Liberman LC, Lipp OV, Spence SH, March S. (2006). Evidence for retarded extinction of aversive learning in anxious children. Behav Res Ther 44:1491–502
- Maren S. (2001). Neurobiology of Pavlovian fear conditioning. Annu Rev Neurosci 24:897–931
- Maren S, Aharonov G, Stote DL, Fanselow MS. (1996). N-methyl-D-aspartate receptors in the basolateral amygdala are required for both acquisition and expression of conditional fear in rats. Behav Neurosci 110:1365–74
- McCallum J, Kim JH, Richardson R. (2010). Impaired extinction retention in adolescent rats: effects of D-cycloserine. Neuropsychopharmacology 35:2134–42
- Mendez P, Bacci A. (2011). Assortment of GABAergic plasticity in the cortical interneuron melting pot. Neural Plasticity 2011:976856
- Merikangas KR, He JP, Burstein M, Swanson SA, Avenevoli S, Cui L, Benjet C, et al. (2010). Lifetime prevalence of mental disorders in U.S. adolescents: results from the National Comorbidity Survey Replication--Adolescent Supplement (NCS-A). J Am Acad Child Adolesc Psychiatry 49:980–9
- Merikangas KR, He JP, Burstein M, Swendsen J, Avenevoli S, Case B, Georgiades K, et al. (2011). Service utilization for lifetime mental disorders in U.S. adolescents: results of the National Comorbidity Survey-Adolescent Supplement (NCS-A). J Am Acad Child Adolesc Psychiatry 50:32–45
- Michels L, Martin E, Klaver P, Edden R, Zelaya F, Lythgoe DJ, Lüchinger R, et al. (2012). Frontal GABA levels change during working memory. PLoS One 7:e31933
- Milad MR, Quirk GJ. (2012). Fear extinction as a model for translational neuroscience: ten years of progress. Annu Rev Psychol 63:129–51
- Milad MR, Vidal-Gonzalez I, Quirk GJ. (2004). Electrical stimulation of medial prefrontal cortex reduces conditioned fear in a temporally specific manner. Behav Neurosci 118:389–94
- Monk CS, McClure EB, Nelson EE, Zarahn E, Bilder RM, Leibenluft E, Charney DS, et al. (2003). Adolescent immaturity in attention-related brain engagement to emotional facial expressions. Neuroimage 20:420–8
- Mrzljak L, Uylings HB, Van Eden CG, Judas M. (1990). Neuronal development in human prefrontal cortex in prenatal and postnatal stages. Prog Brain Res 85:185–222
- Mueller AL, Taube JS, Schwartzkroin PA. (1984). Development of hyperpolarizing inhibitory postsynaptic potentials and hyperpolarizing response to gamma-aminobutyric acid in rabbit hippocampus studied in vitro. J Neurosci 4:860–7
- Newman DL, Moffitt TE, Caspi A, Magdol L, Silva PA, Stanton WR. (1996). Psychiatric disorder in a birth cohort of young adults: prevalence, comorbidity, clinical significance, and new case incidence from ages 11 to 21. J Consult Clin Psychol 64:552–62
- Okaty BW, Miller MN, Sugino K, Hempel CM, Nelson SB. (2009). Transcriptional and electrophysiological maturation of neocortical fast-spiking GABAergic interneurons. J Neurosci 29:7040–52
- Pare D, Duvarci S. (2012). Amygdala microcircuits mediating fear expression and extinction. Curr Opin Neurobiol 22:717–23
- Pare D, Smith Y. (1993). The intercalated cell masses project to the central and medial nuclei of the amygdala in cats. Neuroscience 57:1077–90
- Pattwell SS, Bath KG, Casey BJ, Ninan I, Lee FS. (2011). Selective early-acquired fear memories undergo temporary suppression during adolescence. Proc Natl Acad Sci U S A 108:1182–7
- Pattwell SS, Duhoux S, Hartley CA, Johnson DC, Jing D, Elliott MD, Ruberry EJ, et al. (2012). Altered fear learning across development in both mouse and human. Proc Natl Acad Sci U S A 109:16318–23
- Paus T, Keshavan M, Giedd JN. (2008). Why do many psychiatric disorders emerge during adolescence? Nat Rev Neurosci 9:947–57
- Phelps EA, Delgado MR, Nearing KI, LeDoux JE. (2004). Extinction learning in humans: role of the amygdala and vmPFC. Neuron 43:897–905
- Phelps EA, LeDoux JE. (2005). Contributions of the amygdala to emotion processing: from animal models to human behavior. Neuron 48:175–87
- Pine DS, Cohen P, Gurley D, Brook J, Ma Y. (1998). The risk for early-adulthood anxiety and depressive disorders in adolescents with anxiety and depressive disorders. Arch General Psychiatry 55:56–64
- Pinto JG, Hornby KR, Jones DG, Murphy KM. (2010). Developmental changes in GABAergic mechanisms in human visual cortex across the lifespan. Front Cell Neurosci 4:16
- Pizzorusso T. (2009). Neuroscience. Erasing fear memories. Science 325:1214–15
- Pizzorusso T, Medini P, Berardi N, Chierzi S, Fawcett JW, Maffei L. (2002). Reactivation of ocular dominance plasticity in the adult visual cortex. Science 298:1248–51
- Pollack MH, Otto MW, Sabatino S, Majcher D, Worthington JJ, McArdle ET, Rosenbaum JF. (1996). Relationship of childhood anxiety to adult panic disorder: correlates and influence on course. Am J Psychiatry 153:376–81
- Quirk GJ, Russo GK, Barron JL, Lebron K. (2000). The role of ventromedial prefrontal cortex in the recovery of extinguished fear. J Neurosci 20:6225–31
- Rakic P, Bourgeois JP, Eckenhoff MF, Zecevic N, Goldman-Rakic PS. (1986). Concurrent overproduction of synapses in diverse regions of the primate cerebral cortex. Science 232:232–5
- Rothbaum BO, Davis M. (2003). Applying learning principles to the treatment of post-trauma reactions. Ann N Y Acad Sci 1008:112–21
- Rubenstein JL. (2010). Three hypotheses for developmental defects that may underlie some forms of autism spectrum disorder. Curr Opin Neurol 23:118–23
- Rubenstein JL, Merzenich MM. (2003). Model of autism: increased ratio of excitation/inhibition in key neural systems. Genes Brain Behav 2:255–67
- Santini E, Ge H, Ren K, Pena de Ortiz S, Quirk GJ. (2004). Consolidation of fear extinction requires protein synthesis in the medial prefrontal cortex. J Neurosci 24:5704–10
- Soliman F, Glatt CE, Bath KG, Levita L, Jones RM, Pattwell SS, Jing D, et al. (2010). A genetic variant BDNF polymorphism alters extinction learning in both mouse and human. Science 327:863–6
- Sotres-Bayon F, Quirk GJ. (2010). Prefrontal control of fear: more than just extinction. Curr Opin Neurobiol 20:231–5
- Sotres-Bayon F, Sierra-Mercado D, Pardilla-Delgado E, Quirk GJ. (2012). Gating of fear in prelimbic cortex by hippocampal and amygdala inputs. Neuron 76:804–12
- Sowell ER, Thompson PM, Holmes CJ, Jernigan TL, Toga AW. (1999). In vivo evidence for post-adolescent brain maturation in frontal and striatal regions. Nature Neurosci 2:859–61
- Spear LP. (2004). Adolescent brain development and animal models. Ann N Y Acad Sci 1021:23–6
- Spear LP, Brake SC. (1983). Periadolescence: age-dependent behavior and psychopharmacological responsivity in rats. Dev Psychobiol 16:83–109
- Sullivan RM, Landers M, Yeaman B, Wilson DA. (2000). Good memories of bad events in infancy. Nature 407:38–9
- Tang HH, McNally GP, Richardson R. (2007). The effects of FG7142 on two types of forgetting in 18-day-old rats. Behav Neurosci 121:1421–5
- van Eden CG, Kros JM, Uylings HB. (1990). The development of the rat prefrontal cortex. Its size and development of connections with thalamus, spinal cord and other cortical areas. Prog Brain Res 85:169–83
- Xia Y, Poosch MS, Whitty CJ, Kapatos G, Bannon MJ. (1993). GABA transporter mRNA: in vitro expression and quantitation in neonatal rat and postmortem human brain. Neurochem Int 22:263–70
- Yakovlev PI, Lecours AR. Regional development of the brain in early life. Philadelphia: F.A. Davis Co.; 1967
- Yan XX, Cariaga WA, Ribak CE. (1997). Immunoreactivity for GABA plasma membrane transporter, GAT-1, in the developing rat cerebral cortex: transient presence in the somata of neocortical and hippocampal neurons. Brain Res Dev Brain Res 99:1–19