Abstract
Effective coping strategies build resilience against stress-induced pathology. In the current study, young male rats were categorized as active, passive, or variable copers by observing their responses to being gently restrained on their backs (i.e., the back-test). The rats were subsequently exposed to chronic unpredictable stress, which included several ethologically relevant stressors such as predator odors and calls, for approximately three weeks. During this time, the variable copers, defined as rats that demonstrated a variable as opposed to a rigid response to stress, exhibited more seemingly adaptive responsiveness in three successive forced swim tests than the more consistently responding passive and active copers. This behavioral flexibility was accompanied by increased neuropeptide Y-immunoreactivity in the bed nucleus of the stria terminalis (BNST) and the amygdala and increased fos-immunoreactivity in the BNST. Additionally, the alterations in fecal corticosteroid levels and cardiovascular measures (systolic blood pressure and tail blood volume) between baseline and stress conditions differed according to coping strategy. Factor analysis indicates that variable copers were characterized by a distinct cardiovascular and neural response to the stress exposure. These results suggest that this animal coping model may be useful in discerning the adaptive nature of particular response strategies in the face of environmental exigencies.
Introduction
Chronic stress disrupts an individual's allostatic responses, or the ability to maintain optimal functioning via the coordination of integrative physiological responses, and leads to negative health effects such as cardiovascular disease, immunosuppression (Csermely Citation1998; McEwen Citation2003, Citation2008; Le Moal Citation2007), alterations in brain functions and structure (Lambert et al. Citation1998; Sapolsky Citation2000; McEwen Citation2005a, Citation2005b; Mitra et al. Citation2005; Mirescu and Gould Citation2006), and an increased vulnerability to various mental illnesses such as depression and posttraumatic stress disorder (Charney Citation2004).
In contrast to Selye's suggestion that the stress response is generalized, non-specific, and predictable (Selye Citation1936; Bertok Citation1998), recent findings emphasize the importance of individual differences in coping strategies and their concomitant health effects (Koolhaas et al. Citation1999). Coping styles are identified when an animal can make various responses to a stressor (Koolhaas et al. Citation2007). When faced with a threat, for example, an animal may freeze, attack, or flee. As various stress responses have been evaluated, the term resilience has been introduced to refer to an animal's ability to survive threatening situations with minimal allostatic load (Charney Citation2004; Yehuda et al. Citation2006). Given the specificity and variability of stress responses, more information is needed to determine the most effective coping strategies to protect against allostatic load and the subsequent emergence of mood and anxiety disorders (Bryce et al. Citation1989; Schouten and Wiegant Citation1997; Campbell et al. Citation2003; Cavigelli and McClintock Citation2003; McEwen Citation2003, Citation2005a, Citation2005b, 2008; Lambert Citation2006).
Effective coping strategies likely evolved to provide neurobiological adaptations to situations that threatened an animal's fitness (Wechsler Citation1995). Koolhaas and his colleagues (Citation1999) suggested that the concept of coping styles was useful in the determination of an animal's adaptive functions and subsequent susceptibility to stress-related disease. After viewing variability in the escape response of domesticated young pigs being restrained in a test known as the back-test, it was noted that response strategies differed in rigidity and flexibility. The more active animals seemed to be more rigid, whereas the more passive animals were more flexible and responsive to the specific nature of environmental stimuli. Although, generally, pigs demonstrating a more active response to back restraint (known as proactive) appeared to exhibit the more adaptive response evidenced by less activation of the HPA axis, it has been suggested that, in some cases, the more passive (also known as reactive) copers exhibited the most adaptive response because of their flexibility in responses. In mice, aggressive, proactive males are more successful when the colony conditions are held constant, whereas the less aggressive, reactive males are more successful in more variable conditions (Oortmerssen and Busser Citation1989; Koolhaas et al. Citation1999).
Although not emphasized, a few of the back-test studies noted that some pigs did not easily profile as active or passive copers; some displayed variable responses in multiple tests (e.g., passive on one test, active on another test) that made it impossible to categorize them. These animals were designated doubtful or intermediate copers and, although recognized as being important (Van Erp-Van der Kooij et al. Citation2000), these intermediate copers have also been discarded in other studies (Hessing et al. Citation1993). It is interesting to consider, however, the adaptive value of being classified in this intermediate coping category. Being a more variable coper allows the animal to adjust to the changing demands of environmental situations and may result in enhanced adaptation and resilience compared to the more rigid passive and active coping strategies (Bonanno et al. Citation2004).
Recently, the cotransmitter neuropeptide Y (NPY), a 36 amino acid peptide mediated by at least five classes of receptors, has received the most extensive and consistent support as one of the more prominent peptides implicated in the brain's resilience systems (Kask et al. 2002; Gutman et al. Citation2008). Specifically, NPY has been found to antagonize the behavioral effects of chronic stress through various actions in the brain (Heilig Citation2004). Accordingly, anti-anxiety effects of NPY have been demonstrated in several animal models including the elevated plus maze, the Vogel drinking conflict test, and social interaction tests (Pellow et al. Citation1985; Heilig et al. Citation1989; Heilig Citation2004; Eaton et al. Citation2007). In humans, plasma NPY levels were higher in Special Forces soldiers exposed to a Prisoner of War experience than in non-Special-Forces soldiers (Morgan et al. Citation2000). Additionally, patients suffering from PTSD had lower plasma levels of NPY than healthy subjects (Rasmusson et al. Citation2000).
NPY has also been implicated in gastrointestinal and cardiovascular functions (Redrobe et al. Citation2003; Chronwall and Zukowska Citation2004). Intracerebroventricular administration of NPY, for example, inhibited intestinal motility induced by both stress and corticotropin releasing hormone (Jimenez and Bueno Citation1990; Gue et al. Citation1992). Illustrating the complex effects of NPY, studies have reported vasoconstriction and increased blood pressure following systemic administration (Shine et al. Citation1994) and lowered blood pressure and heart rate following central administration of NPY (Fuxe et al. Citation1983).
Concerning potential neural mechanisms of NPY, repeated administration into the basolateral nucleus of the amygdala resulted in reduced anxiety in the social interaction test, an effect lasting for up to 8 weeks (Sajdyk et al. Citation2008). More specifically, the presence of calcineurin, a protein phosphatase known for its involvement in neuronal remodeling, with NPY receptors within the basolateral amygdala (BLA) neurons has been suggested as a mechanism of the anxiolytic response (Sajdyk et al. Citation2008). Additionally, whole-cell recordings in the bed nucleus of the stria terminalis (BNST) tissue indicated that NPY alters GABAergic transmission, a system also known for its involvement in the anxiety response (Kash and Winder Citation2006). Interestingly, recent research reported that both intracerebroventricular and BLA infusions facilitated the extinction of conditioned fear whereas a NPY Y1 receptor antagonist produced a deficit in extinction retention (Gutman et al. Citation2008). The ability to forget components of conditioned fear contexts that no longer reliably predict threats is likely an important factor in psychobiological resilience.
In the current study, young male Long-Evans rats were profiled with the back-test (adapted from the piglet study of Koolhaas et al. Citation1999) to yield passive, active, and variable copers that were later exposed to various behavioral assessments presented in the midst of a 3 week chronic unpredictable stress (CUS) paradigm. In addition to behavioral coping strategies, NPY- and fos-immunoreactivity were investigated in three brain areas [i.e., paraventricular nucleus of the hypothalamus (PVN), BNST, and BLA]. Fecal corticosterone levels and cardiovascular responses were monitored during both baseline and chronic stress conditions. Based on prior research in our laboratory, it was hypothesized that the variable copers would respond to the various stressors in a manner different from the more consistent passive and active copers; specifically, we expected variable copers to exhibit more NPY-immunoreactivity in assessed brain areas, lower cardiovascular responsivity, lower corticosteroid levels, and more variability in several behavioral challenges.
Methods
Animals
Upon arrival to the laboratory, 40 male 25-day-old Long-Evans rats (Sprague Dawley, IN, USA) were housed in groups of five in 48 cm × 26 cm × 21 cm cages to habituate to laboratory conditions prior to the commencement of the chronic stress paradigm. In order to differentiate each rat in their initial group housing, a color code was placed on their tails with a permanent marker. Following the coping profile assessment, the 24 rats selected for the study were housed individually for the remainder of the study. All cages were lined with Kay-Kob bedding (Kaytee Products, Chilton, WI, USA); rats were given food (Mazuri Rodent Chow, Purina Mills, St Louis, MI, USA) and water ad libitum throughout the study. With the exception of reversed light modifications in the chronic stress protocol, a 12 h:12 h light dark cycle began with lights on at 10.00 h. Ambient temperature was maintained at 24°C. All rats were maintained in accordance with the guidelines of the Randolph-Macon College Institutional Animal Care and Use Committee.
Materials
In the CUS paradigm, fox and raccoon urine (Wildlife Research Center, Anoka, MN, USA) were used as stressors. Additionally, a strobe light (Xenon Strobe Light, Multi Media Electronics, Inc., Farmingdale, NY, USA) was used to simulate environmental challenges such as lightening in thunderstorms, along with an audio tape of wildlife predator calls (played at approximately 90 decibels; Johnny Stewart Wildlife Calls, Waco, TX, USA). The open field apparatus was 122 cm × 91 cm × 51 cm and consisted of a white linoleum floor and walls. Activity in the Open Field was recorded by Videotrack (Viewpoint Life Sciences, Inc., Outterburn Park, QC, Canada). The final stress challenge utilized a wind-up toy truck 6 cm long to simulate a moving novel stimulus. In the midst of the CUS paradigm (see ), several common items were also used including cotton balls for the predator odors, a radio/tape player for the predator calls, small hair clips for the tail clip test, vinegar for the drinking water (approximately 0.2% vinegar/water concentration), toy blocks for novelty exposure (averaging 6.5 × 3.5 cm), soiled clumpable cat litter for an additional predator stimulus, and a 60 × 31 × 53 cm glass aquarium for the forced swim task [water was approximately 30 cm deep and was maintained at room temperature (20°C)].
Table I. Schedule of stressors used in the CUS paradigm.
Coping strategy assessment
To determine each rat's coping strategy, a back-test was conducted in a similar fashion as described with piglets (Koolhaas et al. Citation1999; Van Erp-Van der Kooij et al. Citation2000) approximately 24 h after arrival to the laboratory. During the back-test, the rats were gently restrained in a supine position by holding them at the nape of the neck as well as the base of the tail (). To maintain consistency in these tests, the same trained observers were used for all back-test assessments. During this 1 min test, the number of attempts to escape (defined as “wiggling” or movement of the limbs) was recorded for 60 s. When a rat paused during the escape attempt, a new bout was recorded (Geverink et al. Citation2004). Pilot research in our laboratory indicated that the escape responses appear in bouts rather than one continuous attempt to escape; therefore the number of escape attempt bouts was recorded in the present study. Following the test, rats were returned to their cages. Seven days later, a second back-test was conducted in exactly the same manner as the initial test so that the scores from the first and second assessments could be compared. The number of escape attempts in each test ranged from one to 12 responses. Three categories were subsequently created to categorize coping strategies: active, passive, and variable. Rats were categorized as active copers if they exhibited seven or more escape attempts in both back-test assessments. Rats were considered passive if they exhibited six or less escape attempts during both assessments. Rats that were categorized as active in one trial and passive on the second trial were categorized as variable copers. To maximize the differences among the groups, eight rats with the most “extreme” scores were chosen for each group; i.e., the eight rats with the highest avoidance scores were chosen for the active group, the eight rats with the lowest number of escape attempts were chosen as the passive rats and eight rats demonstrating the greatest differences between the first and second assessments were categorized as variable copers. Thus, 24 rats exhibiting the most representative active, passive, and variable coping responses were chosen from the sample of 40 rats that were assessed.
CUS paradigm
The CUS paradigm was adapted from previously described protocols (Matthews et al. Citation1995; Alfarez et al. Citation2003; Gouirand and Matuszewich Citation2005); however, food restriction was not used so that body weight would not be directly affected. Additionally, to create more ethologically relevant stressors, predator odors, sounds, and simulated non-invasive insect-like “bites” were added to the protocol. This chronic stress model was chosen because it has been shown to generate hallmark signs of exposure to chronic stress such as elevated glucocorticoid levels, adrenal hypertrophy, and reduced thymus weight. Further, the variability of stressors reduces the likelihood of adaptation in a chronic stress paradigm and provides a more relevant model of the variability of stressors typically encountered in an animal's daily life (Herman et al. Citation1995; Joels et al. Citation2004; Munhoz et al. Citation2006).
In the current study, the CUS paradigm persisted for 18 days for 12 rats and 20 days for 12 rats (all coping groups were represented in each group of 12 rats), during which approximately two stressors were presented daily. See for list of stressors used during the chronic stress paradigm. On the final day of CUS, rats were exposed to a novel stressor (i.e., a wind up car), so that stress responses could be assessed one last time prior to terminal procedures (i.e., perfusion).
Behavioral assessments
During the CUS, periodic behavioral assessments were conducted to discern the response patterns of the three coping groups. On the fourth day of the paradigm, during the tail clip task, a small rounded clip was placed at the base of the tail for 5 min. This clip fitted snugly around the tail but did not penetrate or wound the tail. During this time, the following behaviors were observed: Latency to contact the tail clip; duration of freezing (defined as no visible movement for at least 3 s) and the number of attempts the rat made to remove the clip.
On the fifth day of CUS, rats were exposed to a white Lego block in their home cages for 5 min prior to a 3 min forced swim session. During the swim task, latency to float, duration of floating, and the number of dives to the bottom of the aquarium were recorded. This assessment was repeated on the seventh and ninth days of CUS. Finally, on the 13th day of CUS, the Lego block was placed in the cage for 5 min so that the rats' responses to this conditioned stimulus for swimming could be assessed. In previous studies in our laboratory, various responses ranging from freezing to defensive burying have been observed in the presence of this conditioned stimulus. Specifically, the latency to approach the block, duration of freezing, bouts of defensive burying, and number of contacts with the block were recorded; further the increases in time spent floating from day 5 to day 7 as well as from day 7 to day 9 for each rat were recorded.
On day 14 of CUS, an open field test was conducted for 5 min. The video tracking system monitored the number of entries into the central area of the arena, the duration of freezing and total distance covered in the arena.
Cardiovascular assessments
Cardiovascular measurements were collected for all rats during baseline (one day prior to the commencement of CUS) and stress conditions (day 15 of CUS). Rats were placed in traditional restraining holders with an adjustable darkened nose cone to limit visual stimuli and potential additional stressors (Kent Scientific, Torrington, CT, USA; cones were developed for rats ranging in weight from 30–125 g and the length was adjusted to each rat's body length). To facilitate thermoregulation and appropriate blood flow during testing sessions, a warming pad (approximately 46°C) was placed underneath the holders. Cuffs were placed on the base of the tail for physiological monitoring. This volume and pressure non-invasive recording technique provided the following cardiovascular parameters: systolic and diastolic blood pressures, mean arterial pressure, heart rate, tail blood volume, and tail blood flow.
All cardiovascular measurements were collected via the Coda 1 Cardiovascular Cycle 2 software (Kent Scientific). For each testing session, the software was preset at 10 cycles per rat with a 5 s interval between each cycle. For data analysis, the average value (over the 10 cycles) was taken for each dependent measure.
Fecal corticosterone measurement
Fresh fecal samples were collected one day prior to the commencement of CUS and on day 15 of the CUS protocol. When rats were placed in the restraint tubes for each cardiovascular testing session, they were observed closely so that fecal samples could be collected before being contaminated with urine (Good et al. Citation2003; Cavigelli et al. Citation2006). All fecal samples were stored at − 70°C. To extract corticosterone from the samples, wet samples were weighed so that 0.1 g could be transferred to individually labeled test tubes. The fecal samples were added to 1 ml of 100% methanol in a glass test tube and homogenized with the large end of a glass Pasteur pipette. Each sample was then vortexed for 30 s and centrifuged for 10 min at 1000 g. The supernatant was transferred to a 13 × 10 mm glass test tube with a transfer pipette (adapted after Easterbrook et al. Citation2007). Samples were diluted 1:20 with the buffer from the corticosterone ImmunoAssay kit (AssayDesigns, Ann Arbor MI, USA). Assays were processed on a ELx8000 microplate reader interfaced with KC Junior software (Bio Tek, Winooski, VT, USA). Coefficient of Variation percentages were reported as approximately 8% for intra-assay measures and 10% for inter-assay measures.
Histological assessments
Perfusion–fixation was performed 80–100 min following the exposure to the novel stimulus so that fos-immunoreactivity could be assessed. Once rats were anesthetized with an intraperitoneal injection of sodium pentobarbital (100 mg/kg), they were checked closely for lack of responsiveness before the procedure commenced. Initially, the rats were transcardially perfused with phosphate-buffered solution for 3 min, followed by 4% paraformaldehyde for 12 min. Following the perfusion, brains were post fixed for 2 h in 4% paraformaldehyde and then placed in 20% sucrose–phosphate buffer saline solution (at 4°C) overnight. Brains were cut at − 15°C using a 505E Microm cryostat (Walldorf, Morfelden, Germany). Using the Paxinos and Watson (Citation1986) stereotaxic atlas as a guide 12 sections at a thickness of 40 microns were collected beginning at plate 16 in order to assess the BNST (anterior medial area). From these sections, the odd number sections were assigned to the NPY immunoreactivity assessment and the even number sections were assigned to the fos-immunoreactivity assessment so that continuous sections were not processed in either the fos or NPY assays. Another 12 sections were collected beginning at plate 25 through the BLA and PVN of the hypothalamus. Depending on the histological assignment, free-floating sections were exposed to either the primary antibody for NPY at a dilution of 1:6000 (Immunostar, Inc., Hudson, WI, USA) or fos (Immunostar, Inc.) at a dilution of 1:4000 following standard immunocytochemistry procedures. Sections designated as negative controls were not exposed to the primary antibody but were exposed to all subsequent steps. Following exposure to the primary antibody, and the appropriate PBS washes, brains were exposed to the appropriate secondary antibody for 1 h at room temperature at a dilution of 1:200 prior to incubation in avidin–biotin complex, also for 1 h at room temperature, and subsequent visualization with diaminobenzidine (DAB). All tissues were processed at the same time to assure consistency among groups. The secondary antibodies, avidin–biotin complex, and DAB were purchased from Vector, Inc. (Burlingame, CA, USA). All brain sections were mounted on gelatinized slides before being cleared in standard alcohol and clearing solutions.
Histomorphometry
A Zeiss Axioskop light microscope was used to identify the BNST, PVN, and BLA in appropriate brain sections. For the NPY-immunoreactivity analysis, light-thresholding analysis was conducted on 200 × 250 micron fields (at a magnification of 400 × ) of view using Bioquant Neuroimaging software (Nashville, TN, USA) so that the percentage of NPY-immunoreactive tissue per 50,000 sq micron visual field in the central portion of each targeted area could be determined. This strategy assured that approximately 30–50% of targeted brain areas were quantified in each brain section. Consequently, approximately eight visual fields were processed across the six brain sections for each rat and were averaged to yield a representative proportion of NPY-immunoreactive tissue for the specified visual field area in the BNST, PVN, and BLA.
For the fos-immunoreactivity assessment, Neurolucida software (MicroBrightfield, Inc., Williston, VT, USA) was used to quantify the number of fos-immunoreactive cells in the BNST, PVN, and BLA areas. Once again, at 400 × magnification, a 200 × 250 visual field was identified in the targeted area so that the total number of fos-immunoreactive cells could be determined. As described previously, approximately eight visual fields were processed for each of the eight rats in each coping group to yield an average fos-immunoreactive cell count for the specified visual field area for each rat. Because at least 80 microns separated the tissue that was quantified for each slide, the cell bodies were only quantified once in the neuroimaging assessment.
Statistical analysis
For each dependent variable, a one-way analysis of variance was used to determine the effects of coping strategy (passive, active, or variable) on each dependent measure. A Tukey test was used for post hoc comparisons (α = 0.05). SPSS statistical software (Chicago, IL, USA) was used for all statistical processing. For the forced swimming assessment, a 3 × 3 repeated measures ANOVA (three coping groups × three testing phases) was used to discern differences in the coping strategies upon repeated presentation to the same stimulus; likewise, a 2 × 3 repeated measures ANOVA was used for the cardiovascular data to discern the differences between baseline and chronic stress measures.
In an attempt to discern consistent differences across the various dependent measures assessed in the current study, a factor analysis was conducted to determine the most valuable factors characterizing variable copers and consistent copers (i.e., passive and active copers combined). Subsequently, a principal component analysis was used to extract the most relevant factorial dimensions from eight measures of physiological and neural stress reactions assessed in the analysis (i.e., systolic blood pressure; diastolic blood pressure; mean arterial blood pressure; heart rate; corticosterone levels during stress; NPY and Fos-immunoreactivity in the BNST; NPY-immunoreactivity in the amygdala). Subsequently, a scatterplot of two significant factorial dimensions was created to determine if the variable and consistent copers were separated into two distinct groups.
Results
Behavioral responses to stressors
In the forced swim test, the overall analysis indicated a significant main effect of time for the dependent measure of float duration [F(2,42) = 37.2; p < 0.001]. As observed in , there was a general trajectory for all groups to increase their float duration times from the first to the third swims. Further, a between groups effect [F(2,23) = 5.0; p = 0.016] was observed from the first to the second swim; specifically, the variable group increased the float duration time significantly more than the passive and active groups. As indicated in , all rats increased their float times by the third swim assessment.
Figure 2 Float duration (mean ± SEM) in forced swim task. Compared to the passive and active coping rats, the variable coping rats (as defined by the back test) exhibited significant increases in float duration time from the first to second swim (*p = 0.016); n = 8 for each group. Additionally, all rats increased float duration time from the first to third swim **p < 0.001.
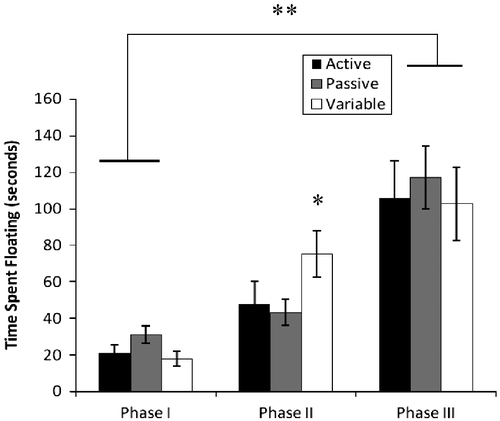
A non-significant trend was observed in the tail clip data; specifically, active rats made more contacts (tugs) toward the clip than the passive rats (p = 0.06; one-tailed test). There were, however, no significant effects found in the behavioral responses to the following measures: response to the conditioned fear stimulus; open field test; or the final stress exposure to the novel stimulus (data not shown).
Cardiovascular measures
During the baseline cardiovascular assessment, a repeated measures analysis indicated a significant effect of time ([F(1,21) = 4.6; p = 0.04] on the systolic blood pressure, with increased values observed during the chronic stress measure. Additionally, a between groups effect [F(2,21) = 3.7; p = 0.04] was observed during the chronic stress assessment. As shown in , passive copers had higher systolic blood pressure than variable copers (145 and 121 mm/Hg, respectively). Focusing on tail blood volume, a significant effect of time was observed [F(1,21) = 4.7; p = 0.04], with blood volume decreasing from the baseline to the chronic stress assessment; volume measures decreased from baseline values of 52 μl to chronic stress values of 39 μl (see for trajectories). Further, in planned comparisons, a significant effect of coping strategy was observed in tail blood volume during the chronic stress assessment [F(2,21) = 4.062; p = 0.03]. Specifically, the passive copers had significantly higher tail blood volumes than the variable rats (see ). Finally, a significant effect of time was observed for heart rate measures [F(1,21) = 105.4; p < 0.001]; specifically, heart rate measures increased by 30% from baseline to chronic stress assessments. No significant effects were observed for diastolic blood pressure or mean arterial pressure.
Figure 3 Systolic blood pressure (mmHg; mean ± SEM) measured via tail cuff during baseline and chronic stress. Whereas no group differences were observed during baseline assessment, the passive rats exhibited higher systolic blood pressure during chronic stress (*p = 0.04); n = 8 for each group.
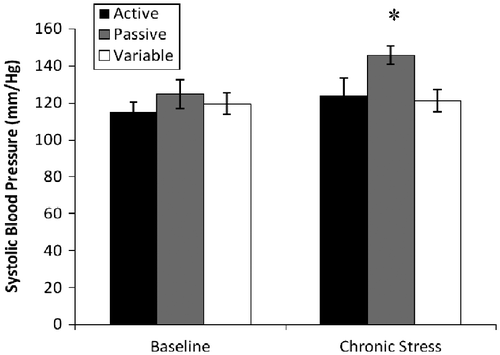
Figure 4 Tail blood volume (mean ± SEM) during baseline and chronic stress. During chronic stress, passive coping animals exhibited higher tail blood volume (μl) than active and flexible animals (*p = 0.03); n = 8 for each group. No group differences in tail blood volume were observed during baseline measurement.
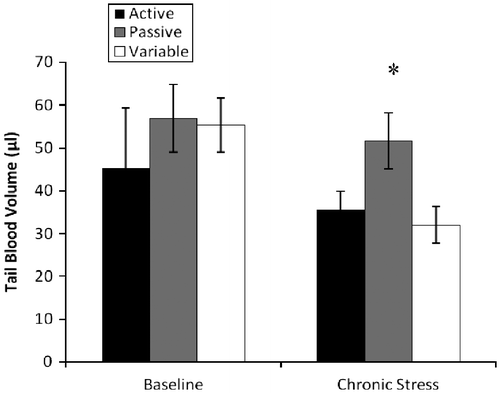
Fecal corticosterone
An overall analysis of corticosterone concentrations indicated a significant effect of time [F(1,20) = 20.73; p < 0.001]. Specifically, higher concentrations were observed in the stress condition than during the baseline condition (141 and 328 ng/ml, respectively). Additionally, a significant time × coping effect was observed [F(2,20) = 8.82; p = 0.002)]. Although there were no group differences in the baseline data, the variable coping rats had higher scores than the passive and active copers during the chronic stress measure (). Comparing the percent increases in corticosterone levels between baseline and chronic stress phases of the study, the variable rats exhibited the most drastic increases compared to the active and passive rats.
Figure 5 Fecal corticosterone concentrations (mean ± SEM) during baseline and chronic stress. A significant interaction (p = 0.002) revealed no difference in baseline values, yet flexible rats had higher concentrations during chronic stress (*p < 0.001); n = 8 for each group. A significant effect of stress revealed that rats had higher corticosterone concentrations during chronic stress than during baseline (*p < 0.001).
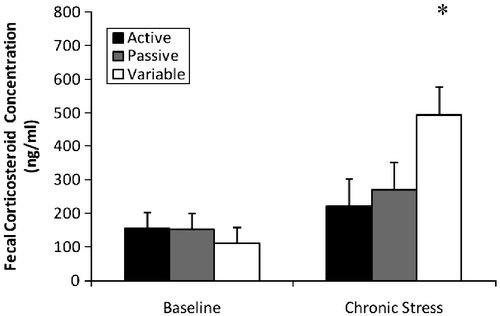
Neuroimmunoreactivity measures: NPY and fos
Significant effects of coping strategy were observed in the amount of NPY-immunoreactive tissue in the BLA and the BNST [(F(2,20) = 19.18; p < 0.001; F(2,20) = 5.24; p = 0.015 respectively). In each case, variable copers had significantly higher levels of immunoreactive tissue than active rats (). Conversely, no significant effects were observed for NPY-immunoreactive (NPY-ir) tissue in the PVN.
Figure 6 Percentage of NPY-immunoreactive tissue (mean ± SEM) in sections of amygdala (n = 7, 8, and 8 for active passive, and flexible groups respectively) and BNST (n = 8, 7, and 8 for passive, active, and flexible groups respectively). The flexible coping group exhibited increased NPY-immunoreactivity in both the amygdala and BNST than the active and passive coping rats. *p < 0.001 and 0.015 respectively.
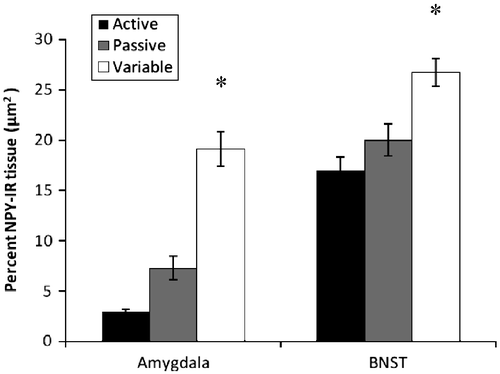
Significant effects for fos-immunoreactive (fos-ir) tissue were observed in the BNST [F(2,18) = 13.33; p < 0.001] with variable copers exhibiting more reactivity than active copers (). No significant effects were observed in fos-ir in the BLA or PVN. Additionally, BNST fos-ir and BNST NPY-ir were significantly correlated (r = 0.631; p = 0.002).
Factor analysis
Based on the percentage of variance explained, principal component analysis extracted two significant components from the eight variables examined. Varimax rotation of these components provided two non-correlated factors, each representing a different aspect of the stress response (). Factor 1 loadings demonstrated a relation between the first component and cardiovascular activation, whereas Factor 2 loadings exhibited a relation between the second component and neural activation. The interpretation of the different factors is dependent on the factor loadings (), that is, the correlation between the original variables and each of the new factors. Since only the first two components had eigenvalues above one, these factors were selected to obtain a linear combination of the eight original variables. Factor 1 explained 46% of the total variance and was characterized as cardiovascular activation because the factor loadings were related to the cardiovascular measures (e.g., blood pressure and heart rate). Factor 2 explained 28.5% of the variance and was characterized as the neural activation factor due to its factor loadings being highly correlated with the neural immunoreactive measures (i.e., NPY and fos) and corticosteroid concentrations during stress (presumably in response to activation of the hypothalamic–pituitary–adrenal axis; ). The factorial scores calculated for each subject are shown in . Factorial scores are calculated on the basis of the two new factors (cardiovascular and neural activation) and are useful in classifying different groups of subjects in the original dataset. The scatterplot of the factorial scores indicated a clear separation between consistent and variable subjects, thus indicating that variable copers were characterized by a distinct cardiovascular and neural response to the stress exposure.
Table II. Total variance explained and eigenvalues for each component of the factor analysis.
Table III. Factor loadings for the two components after the matrix was rotated using the varimax algorithm (varimax finds non-correlated dimensions maximizing the percentage of variance explained).
Figure 8 Scatterplot of individual scores calculated on the basis of the two factors revealed by the principal component analysis with varimax rotation: Factor 1 (on the x-axis) was related to measures of cardiovascular activation (e.g., blood pressure and heart rate); Factor 2 (y-axis) was related to measures of neural activation (fecal corticosterone concentrations, and NPY level and Fos expression in the BNST, and NPY level in the amygdala). Back-test responses: Consistent: passive+active; Variable: variable.
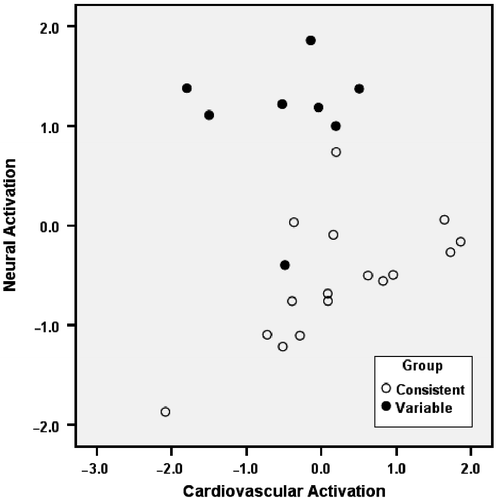
Discussion
The results of the current study suggest that coping strategies assessed early in a rat's life may provide important information about subsequent neurobiological and behavioral responses to stress. Approximately one month following the profile tests, differences continued to be observed among the three coping groups in the form of hormonal, neurophysiological, cardiovascular, and behavioral responses. For example, lower systolic blood pressure (also observed in active copers), increased corticosterone responsivity during chronic stress, increased NPY-immunoreactivity in the BLA and BNST, as well as increased fos-immunoreactivity in the BNST, were observed in variable copers.
The NPY-immunoreactivity data in the current study suggest that the brains of the three coping groups are differentially responsive to the presentation of various stressors. Prior research reporting that antidepressants increase NPY activity in several brain areas, along with electroconvulsive shock therapy (Heilig et al. Citation1988; Redrobe et al. Citation2005), suggests that data providing information elucidating individual differences in NPY responsivity may provide valuable information concerning individual variation in susceptibility to stress-induced psychopathology. The inclusion of a non-stress control group in the current study would have provided important confirmation that these findings extend to baseline conditions; however, the differences observed among the three coping groups during chronic stress provide evidence that, in rats, varying coping strategies defined by the back-test are accompanied by variations in brain NPY levels. Additionally, other research indicates that animals adapt to repeated stress by exhibiting an up-regulation of NPY peptide in the amygdala, an effect not seen after a single administration of a stressor (Thorsell et al. Citation1999). Thus, the neurobiological differences characterizing the various coping strategies are likely to be exacerbated following repeated or chronic stress exposure. In the current study, the lack of differences in corticosterone responses during baseline conditions compared to the dramatic differences observed during chronic stress provides further evidence that the variable coping rats adjust their responsiveness to the presence of challenges and threats encountered in their environment.
In addition to the NPY data, greater fos activation in the BNST, a brain structure involved in emotional responses, was observed in the variable copers. Although the NPY- and fos-immunoreactivity findings are interesting, these measures are rather crude and fail to provide specific information about the specific mechanisms contributing to the observed differences.
Focusing on corticosterone levels, the higher levels in the variable copers, rats previously observed to exhibit more adaptive responses, were an unexpected finding in the current study. Further analysis of the literature, however, reveals it is becoming increasingly apparent that higher corticosteroid levels are indeed adaptive in some situations. Corticosteroids, for example, facilitate both the storage of novel information as well as the extinction of behavior that is no longer adaptive or relevant in a particular situation (De Kloet et al. Citation1999). The emerging theory from recent stress research suggests that, if physiological and environmental events converge optimally, stress enhances learning and memory processes (Joels et al. Citation2006). Accordingly, the unpredictable nature of the CUS paradigm does not allow the animal to make relevant associations between the timing and nature of the stressors, requiring continued activation of the mineralocorticoid and glucocorticoid receptor systems. Along these lines, the predictable nature of the activity stress used in a previous study did not appear to require persistent learning; accordingly, the variable animals had significantly lower corticosterone levels during chronic stress than the active and passive copers (Lambert et al. Citation2006). Although learning was not specifically assessed in the current study, the faster shift from swimming to floating in the repeated swim sessions may indicate facilitated learning, as well as adaptive extinction of non-relevant behavior, in the variable responding animals. These findings support previous perceptions that, once it has been determined that there is no pathway to escape, quietly floating in the forced swim task is a more adaptive response that reduces energy expenditure (Koolhaas et al. Citation2005).
It is interesting to note, as described above, that NPY has also been implicated in facilitated extinction of non-relevant behavioral responses (Gutman et al. Citation2008). Previous research indicating higher levels of corticosterone in NPY-injected animals exhibiting increased resilience to restraint-induced decreases in social interactions further confirms the importance of corticosterone in adaptive stress responses (Sajdyk et al. Citation2008). Persistent increases in corticosterone have also been shown to compromise neural functioning in brain areas such as the hippocampus (Sapolsky Citation2000; McEwen Citation2005a, Citation2005b); thus, the adaptive nature of varying levels of corticosterone is a complex question that provides further sophisticated research to more thoroughly understand.
The factor analysis in the current study provided information about putative factorial dimensions that may be important components of coping strategies. The principal component analysis revealed that the cardiovascular factor explained the most variability of the generated factors. The neural factor (correlated brain measures and corticosterone) was the next factor identified as explaining a significant portion of variability. In order to conduct this analysis, both passive and active copers were converged to form the consistent copers (due to the rigid responses exhibited by each group) that were subsequently compared to the variable coping group. Recently, multidimensional approaches have been utilized to discern relevant factors contributing to varying coping styles. In these studies, emotionality has been identified as an important factor (Koolhaas et al. Citation2007). Additionally, in the distinction between proactive and reactive copers, proactive copers have been characterized by high sympathetic reactivity (accompanied by increased heart rate) whereas the reactive copers have been described as having higher parasympathetic activity (Koolhaas et al. Citation1999, Citation2007). The addition of a third coping group (i.e., the variable coping group) in the current study provides an alternative perspective to these earlier studies. Based on the scatterplot (), the two factors extracted in the current principal component analysis suggest that both neural and cardiovascular factors explain 75% of the variation observed among the variables examined and suggest that differences in relevant physiological measures exist between variable and consistent copers. Although still in its infancy, empirical attempts to discern critical features and dimensions of coping styles are necessary for understanding individual differences in susceptibility to stress-related diseases.
Although not well understood at this time, the significant reductions in tail blood volume in the variable coping rats (especially compared to the passive rats) suggest that peripheral blood flow, as assessed at the base of the tail, during stress is also different in these animals. Whereas the tail blood volumes decreased in all rats from baseline to chronic stress phases of the experiment, a more drastic decrease was observed in the variable copers. The rat uses its tail for both thermoregulation (Raman et al. Citation1983) and balance. Being placed in the restraint tube against the contextual backdrop of the CUS paradigm, provoked varying peripheral blood flow responses in the various coping groups. Perhaps the rerouting of blood to the body's core may be adaptive for a more immediate escape response. More research is required to illuminate the role of altered peripheral blood flow during acute and chronic stress.
Recently, a proposal for translational studies of resilience suggested that an individual differences approach including a diverse range of biological and behavioral responses to stress could be used across both human samples and various species (Yehuda et al. Citation2006). Accordingly, the identification of naturally occurring alterations in various types of coping strategies may provide important clues related to different susceptibility rates observed in the emergence of certain mental illnesses (Redrobe et al. 2002). Psychological resilience was recently defined as “a flexible adaptation to the ever-changing demands of life” (Yehuda et al. Citation2006, p. 380). The variable coping animals in the current study could be viewed as exhibiting flexible adaptation responses to various stressful situations. The variable coping rats exhibited decreased responsiveness in the second forced swim test, a trend toward increased responsiveness in the tail clip test, and no differences in responsiveness in the open-field test; their stress responses appeared to vary in accordance with the type of stressor and its associated context. Thus, the back-test, or other escape tests, appears to be useful in the delineation of important individual differences in adaptive coping strategies. Considering that, ultimately, the fate of the organism may lie in its critical ability to adapt to a fast changing set of circumstances, further investigations of variable and flexible coping strategies should provide valuable information about adaptive responses to stress and the avoidance of allostatic load and associated pathologies.
Declaration of interest: The authors report no conflicts of interest. The authors alone are responsible for the content and writing of the paper.
References
- Alfarez DN, Joels M, Krugers HJ. 2003. Chronic unpredictable stress impairs long-term potentiation in rat hippocampal CA1 area and dentate gyrus in vitro. Eur J Neurosci. 17:1928–1934.
- Bertok L. 1998. Stress and nonspecific resistance. In: Csermely P, editors. Stresss of life from molecules to man. New York: Annals of the New York Academy of Sciences. 1–2.
- Bonanno GA, Papa A, Lalande K, Westphal M, Coifman K. 2004. The importance of being flexible. Psychol Sci. 15:482–487.
- Bryce JW, Waler N, Ghorayeb G, Kanj M. 1989. Life experiences response styles and mental health among mothers and children in Beirut, Lebanon. Soc Sci Med. 28:685–695.
- Campbell T, Lin S, De Vries C, Lambert K. 2003. Coping strategies in male and female rats exposed to multiple stressors. Physiol Behav. 78:925–935.
- Cavigelli SA, McClintock MK. 2003. Fear of novelty in infant rats predicts adult corticosterone dynamics and an early death. Proc Natl Acad Sci. 100:16131–16136.
- Cavigelli SA, Guhad FA, Ceballos RM, Whetzel CA, Nevalainen T, Lang CM, Klein LC. 2006. Fecal corticoid metabolites in aged female male and rats after husbandry-related disturbances in the colony room. J Am Assoc Lab Anim Sci. 45:17–21.
- Charney DS. 2004. Psychobiological mechanisms of resilience and vulnerability: Implications for successful adaptation to extreme stress. Am J Psychiatry. 161:195–216.
- Chronwall BM, Zukowska Z. 2004. Neuropeptide Y, ubiquitous and elusive. Peptides. 25:359–363.
- Csermely P, 1998. Stress of life from molecules to manNew York: The New York Academy of Sciences.
- De Kloet ER, Oitzl MS, Joels M. 1999. Stress and cognition: Are corticosteroids good or bad guys?. Trends Neurosci. 22:422–426.
- Easterbrook JD, Kaplan JB, Glass GE, Pletnikov MV, Klein SL. 2007. Elevated testosterone and reduced 5-HiAA concentrations are associated with wounding and hantavirus infection in male Norway rats. Horm Behav. 52:474–481.
- Eaton K, Sallee FR, Sah R. 2007. Relevance of neuropeptide Y (NPY) in psychiatry. Curr Top Med Chem. 7:1645–1659.
- Fuxe K, Agnati LF, Harfstrand A, Zini I, Tatemoto K, Pich EM, Hokfelt T, Mutt V, Terenius L. 1983. Central administration of neuropeptide Y induces hopotension, bradypnea, and EEG synchronization in the rat. Acta Physiol Scand. 118:189–192.
- Geverink NA, Heetkamp MJW, Schouten WGP, Wiegant VW, Schrams JW. 2004. Backtest type and housing condition of pigs influence energy metabolism. J Am Sci. 82:1227–1233.
- Good T, Khan MZ, Lynch JW. 2003. Biochemical and physiological validation of a corticosteroid radioimmunoassay for plasma and fecal samples in oldfield mice (Peromyscus polionotus). Physiol Behav. 80:405–411.
- Gouirand AM, Matuszewich L. 2005. The effects of chronic unpredictable stress on male rats in the water maze. Physiol Behav. 86:21–31.
- Gue M, Junien JL, Del Rio C, Bueno L. 1992. Neuropeptide Y and sigma ligand (JO 1784) suppress stress-induced colonic motor disturbances in rats through sigma and cholecystokinin receptors. J Pharmacol Exp Ther. 261:850–855.
- Gutman AR, Yang Y, Ressler KJ, Davis M. 2008. The role of neuropeptide Y in the expression and extinction of fear-potentiated startle. J Neurosci. 28:12682–12690.
- Heilig M. 2004. The NPY system in stress, anxiety and depression. Neuropeptides. 38:213–224.
- Heilig M, Wahlestedt C, Ekman R, Widerlov E. 1988. Antidepressant drugs increase the concentration of neuropeptide Y (NPY)-like immunore activity in the rat brain. Eur J Pharmacol. 147:465–467.
- Heilig M, Soderpalm B, Engel JA, Widerlov E. 1989. Centrally administered neuropeptide Y (NPY) produces anxiolytic-like effects in animal anxiety models. Psychopharmacology. 98:524–529.
- Herman JP, Adams D, Prewitt C. 1995. Regulatory changes in neuroendocrine stress-integrative circuitry produced by a variable stress paradigm. Neuroendocrinology. 61:180–190.
- Hessing MJC, Hagelso AM, van Beek JA, Wiepkema PR, Schouten WGP, Krukow R. 1993. Individual behavioural characteristics in pigs. Appl Anim Behav Sci. 37:285–295.
- Jimenez M, Bueno L. 1990. Inhibitory effects of neuropeptide Y (NPY) on CRF and stress-induced cecal motor response in rats. Life Sci. 47:205–211.
- Joels M, Karst H, Alfarez D, Heine VM, Qin Y, van Riel E, Verkuyl M, Lucassen PJ, Krugers HJ. 2004. Effects of chronic stress on structure and cell function in rat hippocampus and hypothalamus. Stress. 7:221–231.
- Joels M, Pu Z, Wiegert O, Oitzl MS, Krugers HJ. 2006. Learning under stress: How does it work?. Trends Cogn Sci. 10:152–158.
- Kask A, Harro J, von Horsten S, Redrobe JP, Dumont Y, Quirion R. 2002. The neurocircuitry and receptor subtypes mediating anxiolytic-like effects of neuropeptide Y. Neurosci Biobehav Rev. 26:259–283.
- Kash TL, Winder DG. 2006. Neuropeptide Y and corticotrophin-releasing factor bi-directionally modulate inhibitory synaptic transmission in the bed nucleus of the stria terminalis. Neuropharmacology. 51:1013–1022.
- Koolhaas JM, Korte SM, De Boer SF, Van Der Begt BJ, Van Reenen CG, Hopsger H, De Jong IC, Ruis MAW, Blokhuis HJ. 1999. Coping styles in animals: Current status in behavior and stress-physiology. Neurosci Biobeh Rev. 23:925–935.
- Koolhaas JM, de Boer SF, Buwalda B. 2005. Stress. In: Whishaw IQ, Kolb B, editors. The behavior of the laboratory rat. New York: Oxford University Press.
- Koolhaas JM, de Boer SF, Buwalda B, van Reenen K. 2007. Individual variation in coping with stress: A multidimensional approach of ultimate and proximate mechanisms. Brain Behav Evol. 70:218–226.
- Lambert KG. 2006. Rising rates of depression in today's society: Consideration of the roles of effort-based rewards and enhanced resilience in day-to-day functioning. Neurosci Biobehav Rev. 30:497–510.
- Lambert KG, Buckelew SK, Staffiso-Sandoz G, Garrga S, Carpenter W, Fisher J, Kinsley CH. 1998. Activity-stress induces atrophy of apical dendrites of hippocampal pyramidal neurons in male rats. Physiol Behav. 65:43–49.
- Lambert KG, Tu K, Everette A, Love G, McNamara I, Bardi M, Kinsley CH. 2006. Explorations of coping strategies, learned persistence, and resilience in Long–Evans rats. Ann NY Acad Sci. 1094:319–324.
- Le Moal M. 2007. Historical approach and evolution of the stress concept: A personal account. Psychoneuroendocrinology. 32:S3–S9.
- Matthews K, Forbes N, Reid IC. 1995. Sucorse consumption as an hedonic measure following chronic unpredictable mild stress. Physiol Behav. 57:241–248.
- McEwen BS. 2003. Interacting mediators of allostasis and allostatic load: Towards an understanding of resilience in aging. Metabolism. 52:10–16.
- McEwen BS. Glucocorticoids, depression and mood disorders: Structural remodeling in the brain. Metab: Clin Exp. 2005a; 54:20–23.
- McEwen BS. Stressed or stressed out: What is the difference?. J Psychiatry Neurosci. 2005b; 30:315–318.
- McEwen BS. 2008. Central effects of stress hormones in health and disease: Understanding the protective and damaging effects of stress and stress mediators. Eur J Pharmacol. 583:174–185.
- Mirescu C, Gould E. 2006. Stress and adult neurogenesis. Hippocampus. 16:233–238.
- Mitra R, Jadhav S, McEwen BS, Vyas A, Chatterji S. 2005. Stress duration modulates the spatiotemporal patterns of spine formation in the basolateral amygdala. Proc Natl Acad Sci. 102:9371–9376.
- Morgan C, Wang S, Southwick SM, Rasmusson A, Hazlett G, Hauger RL, Charney DS. 2000. Plasma neuropeptide Y concentrations in humans exposed to military survival training. Biol Psychiatry. 47:902–909.
- Munhoz CD, Lepsch LB, Kawamoto EM, Malta MB, de Sa Lima L, Avellar MCW, Sapolsky RM, Scavone C. 2006. Chronic unpredictable stress exacerbates lipopolysaccharide-induced activation of nucluear factor B in the frontal cortex and hippocampus via glucocorticoid secretion. J Neurosci. 26:3813–3820.
- Oortmerssen GA, Busser J. 1989. Studies in wild house mice III: Disruptive selection on aggression as a possible force in evolution. In: Brain PF, Mainardi D, Parmigiani S, editors. House mouse aggression: A model of understanding the evolution of social behavior. Chur: Harwood Academic Publishers. 87–117.
- Paxinos G, Watson C. 1986. The rat brain in stereotaxic coordinates. New York: Academic Press.
- Pellow S, Chopin P, File SE, Briley M. 1985. Validation of open:Closed arm entries in an elevated plus-maze as a measure of anxiety in the rat. J Neurosci Methods. 14:149–167.
- Raman ER, Roberts MF, Vanhuyse VJ. 1983. Body temperature control of rat tail blood flow. Am J Physiol Regul Integr Comp Physiol. 245:426–432.
- Rasmusson AM, Hauger RL, Morgan CA, Bremner JD, Charney DS, Southwick SM. 2000. Low baseline and yohimbine-stimulated plasma neuropeptide Y (NPY) levels in combat-related PTSD. Biol Psychiatry. 47:526–539.
- Redrobe JP, Dumont Y, Herzog H, Quirion R. 2003. Neuropeptide Y (NPY) Y2 receptors mediate behaviour in two animal models of anxiety: Evidence from Y2 receptor knockout mice. Behav Brain Res. 141:251–255.
- Redrobe JP, Dumont Y, Quirion R. 2002. Neuropeptide Y (NPY) and depression: From animal studies to the human condition. Life Sci. 71:2921–2937.
- Redrobe JP, Dumont Y, Fournier A, Baker GB, Quirion R. 2005. Role of serotonin (5-HT) in the antidepressant-like properties of neuropoetide Y (NPY) in the mouse forced swim test. Peptides. 26:1394–1400.
- Sajdyk TJ, Johnson PL, Leitermann RJ, Fitz SD, Dietrich A, Morin M, Gehlert DR, Urban JH, Shekhar A. 2008. Neuropeptide Y in the amygdala induces long-term resilience to stress-induced reductions in social responses but not hypothalamic-adrenal-pituitary axis activity or hyperthermia. J Neurosci. 28:893–903.
- Sapolsky RM. 2000. Glucocorticoids and hippocampal atrophy in neuropsychiatric disorders. Arch Gen Psychiatry. 57:925–935.
- Schouten WGP, Wiegant VM. 1997. Individual responses to acute and chronic stress in pigs. Acta Physiol Scand. 640:188–191.
- Selye H. 1936. A syndrome produced by diverse noxious agents. Nature. 138:32.
- Shine J, Potter EK, Biden T, Selbie LA, Herzog H. 1994. Neuropeptide Y and regulation of the cardiovascular system. J Hypertens. 12:S41–S45.
- Thorsell A, Carlsson K, Ekman R, Heilig M. 1999. Behavioral and endocrine adaptation, and up-regulation of NPY expression in rat amygdale following repeated restraint stress. NeuroReport. 10:3003–3007.
- Van Erp-Van der Kooij E, Kuijpers HH, Schrama JW, Ekkel ED, Tielen MJM. 2000. Individual behavioural characteristics in pigs and their impact on production. Appl Anim Behav Sci. 66:171–185.
- Wechsler B. 1995. Coping and coping strategies: A behavioural view. Appl Anim Behav Sci. 43:123–134.
- Yehuda R, Flory JD, Southwick S, Charney DS. 2006. Developing an agenda from translational studies of resilience and vulnerability following trauma exposure. Ann NY Acad Sci. 1071:379–396.