Abstract
Cancer has become one of the most difficult health challenges of our time, accounting for millions of deaths yearly. Systemic chemotherapy is the most common therapeutic approach; however, considerable limitations exist including toxicities to healthy tissues and low achievable drug concentrations at tumor sites. More than 85% of human cancers are solid tumors, which can greatly benefit from localized delivery. This approach allows for high drug concentrations at the target site, lower systemic toxicity, and extended drug exposure which may be beneficial for cell cycle-specific drugs. Polymers have been widely considered in the development of localized delivery systems. This review focuses on both natural and synthetic biodegradable polymers that have been explored for localized chemotherapy, exploring their advantages, disadvantages, and clinical potential while citing examples of their use in pre-clinical development.
Introduction
Cancer is one of the leading causes of death worldwide, accounting for millions of deaths each year (CitationMa & Yu, 2006). Systemic chemotherapy is the most commonly used therapeutic strategy, although considerable limitations exist. It involves intravenous administration of anti-cancer drugs at maximum tolerable doses, which leads to severe toxicities in healthy tissues that range from neutropenia to cardiomyopathy (CitationGasparini, 2001; CitationScharovsky et al., 2009). Further, systemic chemotherapy is not efficient in delivering drugs to target sites at therapeutic concentrations, and maintaining adequate drug levels within tumors is a challenge (CitationJang et al., 2003). Chemotherapeutic delivery to solid tumors systemically involves barriers, including transport along the blood circulatory system to tissues, across vasculature walls into distant tumor sites and through interstitial space within a tumor (CitationJang et al., 2003). As a result, only a fraction of the administered dose reaches tumor cells, limiting therapeutic efficacy and increasing toxicity to healthy tissues.
Although various routes for chemotherapeutic delivery have been investigated (), localized delivery has gained increased attention in cancer therapy. This strategy maintains low systemic drug levels while ensuring therapeutic levels at tumor sites. As solid tumors account for 85% of all cancers, this strategy has the potential to dramatically deliver more efficient drug therapy () (CitationFung & Saltzman, 1997; CitationJang et al., 2003). In this review, localized chemotherapy refers to implantation or injection within (i.e. intra-tumoral) or in close proximity to the tumor site(s). Drug retention at target sites, which decreases systemic drug exposure, is an important consideration for minimal toxicity and maximal efficacy. This can be accomplished through the use of biomaterials which can retain the drug at the desired implant sites.
Table 1. Different approaches of administration for cancer chemotherapy (adapted from CitationExner & Saidel, 2008).
Figure 1. Advantages of localized chemotherapy over conventional systemic chemotherapy for the treatment of solid tumors.
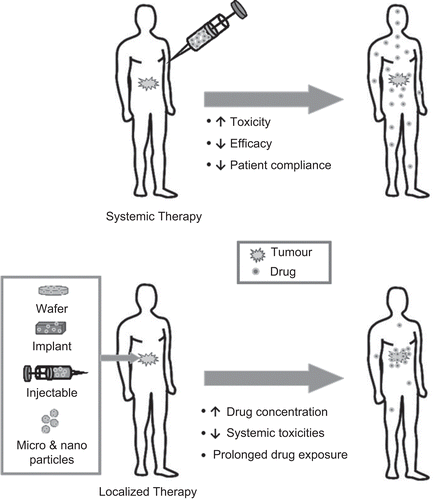
Polymers have been widely explored in the area of drug delivery due to their customizable nature which allows for the development of various systems such as implants, pastes, hydrogels, and/or micro/nanoparticles (CitationJagur-Grodzinski, 2009; CitationKim et al., 2009). The options for fabricating polymeric drug delivery systems are vast; however, one of the challenges in their use is biocompatibility (CitationPark & Park, 1996). The immune response elicited by a polymeric material can result in adverse effects as well as influence drug release properties (CitationRihova, 1996). An acute inflammatory response occurs upon implantation or injection and activation of macrophages, foreign body giant cells, and fibroblasts, resulting in fibrous encapsulation of the system (CitationRihova, 1996; CitationPark & Park, 1996). This encapsulation is a normal tissue response to a foreign material and does not necessarily denote a lack of biocompatibility, such as calcification and necrosis would (CitationRihova, 1996). However, the extent of encapsulation and its effects on drug release will dictate the biocompatibility of the material. It is also important to consider the degradation products of a polymer, as these may elicit immune or toxic responses. Biodegradation is also important, since surgical removal of the delivery system would decrease patient compliance. Factors influencing biodegradation of polymers include chemical structure and composition, physico-chemical factors (ion exchange, ionic strength, and pH), physical factors (shape, size, and porosity), morphology or microstructure, and whether degradation occurs through enzymatic or hydrolysis routes (CitationPillai & Panchagnula, 2001). These properties must be carefully considered when designing drug delivery systems so that proper biodegradation can be ensured.
outlines biodegradable polymers investigated for drug delivery applications. Although localized chemotherapy is promising, only a few polymer-based formulations have reached clinical testing. These include the poly(lactic-co-glycolic acid) (PLGA) based localized injectable delivery system OncoGel™ (CitationElstad & Fowers, 2009) and the implantable system Gliadel® Wafer, composed of a polyanhydride copolymer, for treatment of brain cancer (CitationWestphal et al., 2003; CitationAttenello et al., 2008). Many polymeric delivery systems for localized chemotherapy fail to reach the market as results obtained pre-clinically often do not translate successfully in clinical development. An example is Paclimer®, polyphosphoester microspheres for localized delivery of paclitaxel. This formulation showed promise in pre-clinical studies but failed during Phase I testing due to biocompatibility issues associated with the polymer (CitationHarper et al., 1999; CitationArmstrong et al., 2006).
Table 2. Biodegradable polymers used in drug delivery applications (adapted from CitationPillai & Panchagnula, 2001).
This review will explore biodegradable polymeric drug delivery systems pre-clinically investigated for localized chemotherapy that have shown anti-tumor promise in animal models of cancer. We have chosen to focus on delivery systems for chemotherapeutics only; thus, systems designed to deliver biologics will not be discussed. This review discusses polymers employed as carriers, and therefore does not include polymer–drug conjugates. Specifically, the review outlines both naturally occurring and synthetic polymers including polysaccharides (chitosan, alginates, and hyaluronan), protein-based polymers (collagen, gelatine, and albumin), polyesters, and polyanhydrides. Advantages, disadvantages, and clinical potential of the different classes of polymers are discussed.
Natural polymers
Natural polymers are extracted from abundant natural resources and many have excellent biocompatibility and degrade into non-toxic, non-immunogenic components (CitationPillai & Panchagnula, 2001). These include polysaccharides and protein-based polymers. Polysaccharides interact with living cells and have excellent hemocompatibility, while protein-based polymers form scaffolds that resemble the extracellular matrix and are, therefore, retained within the site of administration (CitationMalafaya et al., 2007). As described below, localized chemotherapeutic delivery systems formed from natural polymers show great promise. However, certain drawbacks exist including complexities associated with their purification, immunogenicity, difficulties in processing, the possibilities of disease transmission from their source organisms to humans, and batch-to-batch variations (CitationLee & Shin, 2007; CitationNair & Laurencin, 2007).
Polysaccharides
Polysaccharides are biopolymers that consist of simple sugar units. Variations in the nature, molecular weight, and structure of these units give rise to the variety of polysaccharide-based polymers available (CitationChourasia & Jain, 2004). These are generally inexpensive as they are abundantly found in nature and can be derived from algals (e.g. alginate), plants (e.g. pectin, guar gum), microbes (e.g. dextran, xanthan gum), and animals (e.g. chitin, chondroitin) (CitationSinha & Kumria, 2001). They are stable, non-toxic, and are good candidates for developing biocompatible drug delivery materials (CitationLiu et al., 2008). The hydrophilic groups that are found on polysaccharides such as chitosan, alginates, and hyaluronan form bioadhesions with biological tissues, potentially increasing drug retention in tissues such as cancerous tumors (CitationLiu et al., 2008).
Chitosan
Chitosan is a linear natural polysaccharide composed of β-(1→4)-2-amido-2-deoxy-D-glucan (glucosamine) and β-(1→4)-2-acetamido-2-deoxy-D-glucan (acetyl glucosamine) units, and is obtained by deacetylation of chitin, which is found in crustaceans, insects, and certain fungi (CitationMourya & Inamdar, 2008). Chitosan has excellent biocompatibility, low toxicity, immunostimulatory activities, antibacterial and anti-fungal action, and anti-coagulant properties (CitationDodane & Vilivalam, 1998; CitationKumar, 2000). Furthermore, the degradation products of chitosan (i.e. aminosugars) are non-toxic, non-immunogenic, and non-carcinogenic (CitationMuzzarelli, 1993; CitationZheng et al., 2007). In cancer therapy applications, chitosan is attractive as it has demonstrated anti-tumor activity (CitationQin et al., 2002; CitationMaeda & Kimura, 2004). Unfortunately, chitosan-based localized delivery systems have not yet reached clinical use. Achieving a high degree of purity is a great challenge, and contaminants or unreacted cross-linking agents can significantly impact chitosan’s safety profile (CitationKean & Thanou, 2010). Furthermore, some chitosan derivatives are not degraded by natural enzymes ). Once these and other issues have been resolved, chitosan will likely have wide clinical application, as it has shown much promise in pre-clinical studies.
CitationTa et al. (2009) recently formulated an injectable chitosan-based doxorubicin hydrogel for osteosarcoma therapy. The hydrogel released its drug load over 19 days, effectively inhibiting primary tumor growth and metastasis to an extent 1.5-fold greater than free doxorubicin, while also decreasing the systemic toxicities associated with this drug. Chitosan was also employed by CitationRuel-Gariepy et al. (2004) to prepare an injectable paclitaxel delivery system that uses the BST-Gel™ platform technology developed at Biosyntech Inc., intended for intra-tumoral injection in the treatment of breast cancer. The group formulated an in situ thermosensitive gelling hydrogel that is liquid at room temperature and becomes a gel implant upon injection. One intra-tumoral injection (40 mg/kg) resulted in equivalent tumor inhibition to that obtained after four 10 mg/kg doses of intravenous paclitaxel in a murine xenograft model of breast cancer. Another thermosensitive chitosan hydrogel system has been recently developed by CitationHan et al. (2008) for the simultaneous intra-tumoral delivery of doxorubicin and the vaccinia virus to elicit immune-mediated destruction of tumors. While immunotherapies are not effective against established tumors on their own, synergistic effects are seen when combined with cytotoxic agents. When compared to monotherapy, greater anti-tumor activity and prolonged survival were observed in a subcutaneous murine model of cervical cancer.
CitationGrant et al. (2005) designed an implantable chitosan film formulated with phospholipids to provide continuous intra-peritoneal delivery of paclitaxel in the treatment of ovarian cancer. No fibrous encapsulation or toxicities of drug-free or paclitaxel-loaded film were observed in mice over a 4-week period after implantation in the peritoneal cavity (CitationHo et al., 2005). Complete tumor inhibition was achieved in a murine xenograft model of human ovarian cancer, whereas only 47% inhibition was observed in animals given equivalent bolus intraperitoneal doses of paclitaxel (CitationVassileva et al., 2007). Intermittent doses of paclitaxel also caused a pronounced induction of MDR1/P-glycoprotein, which plays a large role in multi-drug resistance, while continuous delivery of paclitaxel provided by the film did not cause MDR1 induction (CitationHo et al., 2006). To circumvent the need for surgical implantation, CitationGrant et al. (2008) and CitationZahedi et al. (2009) more recently developed injectable chitosan-phospholipid depot forming formulations for paclitaxel and docetaxel, respectively. No signs of toxicity or inflammation were detected upon administration of drug-free or drug-loaded formulation, and only a very thin fibrous capsid was observed within 1 month which did not interfere with in vivo drug release (CitationDe Souza et al., 2009; CitationZahedi et al., 2009). A dose-dependent response of the docetaxel formulation was observed in a xenograft model of human ovarian cancer in mice, with significant tumor inhibition of 87.3 ± 9.3% at clinically relevant doses () (CitationZahedi et al., 2009).
Figure 2. An injectable polymer-lipid docetaxel formulation (PoLigel-Docetaxel) is efficacious in the localized treatment of SKOV3 ovarian cancer xenografts. The PoLigel-Docetaxel was injected intraperitoneally 14 days following SKOV3 inoculation. Significant difference was found between treatment groups and controls (p < 0.05). Error bars are expressed as standard error (n = 4). Adapted from CitationZahedi et al. (2009).
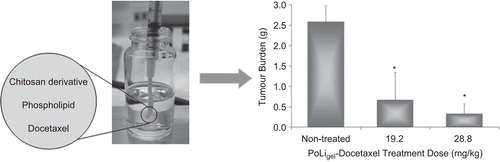
CitationObara et al. (2005) created an in situ forming chitosan-based hydrogel system for subcutaneous intra-tumoral drug delivery. An azide-chitosan-lactose derivative (Az–CH–LA) solution containing paclitaxel was injected beneath subcutaneous tumors derived from Lewis lung cancer cells. An optical fibre was then inserted into the Az–CH–LA solution through the needle pore and UV-laser irradiation was performed for 30 s to convert the solution into an insoluble hydrogel matrix. Over a 14 day study period, the paclitaxel-incorporated Az–CH–LA hydrogel inhibited tumor growth more effectively than drug-free Az–CH–LA hydrogel or free paclitaxel. Furthermore, there was a marked reduction in the number of CD34-positive vessels in the tumor microenvironment, indicating a strong inhibition of angiogenesis.
The effectiveness of using a chitosan-β-glycerophosphate (C-GP) system to locally deliver sustained doses of camptothecin to a RIF-1 fibrosarcoma mouse model was demonstrated in a study by CitationBerrada et al. (2005). Intra-tumoral injection led to greater tumor reduction than intra-peritoneal doses of camptothecin. Animals treated in this manner reached the end points on day 8, while those treated with C-GP-loaded camptothecin survived for 25 days.
Alginates
Alginate is a natural linear anionic heteropolysaccharide consisting of repeat units of β-D-mannuronic acid (M) and its C-5 epimer, α-L-guluronic acid (G) joined together by 1,4-glycosidic linkages (CitationShilpa et al., 2003). Alginates are extracted from brown seaweed and are recognized for their lack of toxicity as evidenced in pre-clinical studies (CitationTonnesen & Karlsen, 2002). This polymer has great potential for applications in drug delivery, owing to its incredible versatility. For instance, both neutral and charged gels can be made depending on the pH of the preparation medium, allowing for retention of drugs with a wide range of physicochemical properties. Yet, as with other polymers extracted from natural sources, many impurities are co-extracted with alginates including endotoxins, heavy metals, and proteins (CitationTonnesen & Karlsen, 2002). Pyrogenicity can be controlled, but not completely eliminated, if ultrapure grades of alginates are used. Thus, local delivery systems formed from alginates are still in the pre-clinical development stage.
Recently, CitationAbe et al. (2008) investigated a local delivery system for paclitaxel in the form of hydroxyapatite-alginate composite beads for therapy of metastatic spine cancer in a rat model. Animals in the control group (drug-free beads) developed hind-limb paralysis at 11 days and died within 16 days. Animals treated with intra-tumoral injections of the paclitaxel-loaded beads showed a 140–150% prolongation in disease-free survival compared to controls. Moreover, significant therapeutic effects were not seen in animals dosed with up to 30-fold higher intravenous doses of paclitaxel solution, highlighting the enhanced effect of localized delivery. CitationBouhadir et al. (2001) developed a hydrogel for localized and controlled delivery of anti-cancer agents by oxidizing sodium alginate and cross-linking with adipic dihydrazine. The amount of adipic dihydrazine used in the formulation was modified to manipulate the rate of drug release, which could be varied from days to weeks due to ionic interactions between the drugs and the polymer. The formulations can successfully load and release methotrexate, doxorubicin, and mitoxantrone simultaneously or individually. An alginate implant to encapsulate endostatin-secreting cells for the treatment of malignant brain tumors was also developed by CitationRead et al. (2001). Sustained local release of endostatin from the implant was found to successfully decrease vascular density and diameter, inhibiting glioma cell invasion, and decreasing tumor volume. The anti-angiogenic activity of endostatin was tumor-specific, as vessels from healthy tissues formed over the implant. The authors examined the biocompatibility of the system subcutaneously and intracranially, both of which showed no signs of an immune reaction.
Hyaluronan
Hyaluronan (also referred to as hyaluronic acid) is an anionic, non-sulfated glycosaminoglycan composed of D-glucuronic acid and D-N-acetylglucosamine, linked via alternating β-1,4 and β-1,3 glycosidic bonds, and is distributed widely throughout connective, epithelial, and neural tissues (CitationFraser et al., 1997). Hyaluronan and its derivatives have been developed as topical, injectable, and implantable vehicles for the delivery of biologically active molecules due to their biocompatibility, unique viscoelastic nature, and non-immunogenicity (CitationLiao et al., 2005; CitationYadav et al., 2008). However, their use in the development of localized delivery systems of anti-cancer agents has been rather limited. Recently, CitationAl-Ghananeem et al. (2009) reported on novel paclitaxel-loaded cross-linked hyaluronan nanoparticles for the local treatment of breast cancer. Intra-tumoral administration of the drug-loaded nanoparticles in female rats showed effective inhibition of tumor growth in all treated rats over 50 days. In the case of the free paclitaxel-treated group, the mean tumor volume increased linearly to a size that was 4.9-fold larger than the baseline volume at 57 days post-drug administration.
Protein-based
Proteins are amino acid polymers arranged in a three-dimensional folded structure. As one of the most important classes of biomolecules, proteins play a vital role in virtually every intra- and extra-cellular process. Proteins and other amino acid-derived polymers are major components of natural tissues, as such they are the preferred biomaterials for development of sutures, hemostatic agents, and scaffolds for tissue engineering (CitationNair & Laurencin, 2007). Furthermore, protein-based biomaterials are known to undergo naturally-controlled degradation. They generally show good biocompatibility, due in part to their ability to mimic the extracellular matrix (CitationMalafaya et al., 2007). A significant advantage of protein-based polymers over polysaccharides is the ability to use recombinant protein technologies which allows polymer properties to be precisely defined, which decreases batch-to-batch variation (CitationMalafaya et al., 2007). On the other hand, as proteins are very susceptible to degradation, a major limitation in the clinical use of these polymers is the development of stable formulations (CitationHawe & Friess, 2007). The principal protein-based polymers that have been used to develop localized chemotherapy formulations include albumin, collagen, and gelatin.
Albumin
Albumin is a protein of 584 amino acids, is highly soluble, has a strong negative charge, and makes up half of the normal intra-vascular protein mass (CitationMendez et al., 2005). Albumin has been used in microsphere preparation for lipophilic drug delivery due to its widespread availability in nature, lack of toxicity and anti-genicity, and its ability to prolong drug levels in the systemic circulation (CitationPatil, 2003). One obstacle in making albumin available for clinical use is that albumin is extracted from human or animal plasma, posing the risk of blood-borne pathogen transmission (CitationHawe & Friess, 2007). This can be avoided by high-temperature pasteurization, which could in turn denature the protein. However, various desirable properties of albumin make it an attractive natural polymer for localized delivery of anti-cancer agents. For instance, albumin has been observed to accumulate in the tumor interstitium, resulting in high intra-tumoral concentrations of drugs bound to it (CitationRugo, 2008).
A novel tissue adhesive composed of human serum albumin (HAS) and tartaric acid derivative (TDA) was developed for delivery of doxorubicin hydrochloride (CitationKakinoki & Taguchi, 2007). Intra-tumoral injection in mice with subcutaneous human colon carcinoma xenografts reduced tumor volume compared to non-treated, doxorubicin hydrochloride, and drug-free HSA–TAD system treatment groups. Another formulation exploring intra-tumoral delivery was developed by CitationAlmond et al. (2003), in the form of injectable mitoxantrone-albumin microspheres for breast cancer treatment. When administered intra-tumorally in a murine mammary adenocarcinoma model, greater survival was observed, and much larger doses could be tolerated than that seen after systemic administration. Intra-tumoral delivery is particularly beneficial in breast cancer, as drug that escapes the tumor microenvironment will drain through the lymph nodes, which is the most common site of breast cancer metastasis (CitationAlmond et al., 2003).
Collagen
Collagen is the main structural protein in the bodies of all vertebrates (CitationGelse et al., 2003). Due to its large abundance in the human body and high biocompatibility, collagen has been used for several years in suturing materials and has current widespread application in tissue engineering (CitationWallace & Rosenblatt, 2003). In localized chemotherapeutic applications, collagen has been under-explored, one issue being that collagen has poor mechanical properties, undergoing rapid degeneration shortly after implantation (CitationFriess, 1998). One approach to overcome this is through cross-linking methods.
Recently, CitationYe et al. (2008) developed a collagen film loaded with polylactic acid microspheres containing epirubicin for intra-tumoral implantation. Intra-tumoral implantation in a murine liver cancer model resulted in considerably greater tumor inhibition than intra-tumoral administration of the epirubicin microspheres alone. Prior to this study, the only other application of collagen for localized chemotherapeutic delivery found in the literature dates back to 1995, when CitationDavidson et al. (1995) evaluated a collagen matrix for localized delivery of cisplatin following tumor resection, which completely prevented tumor recurrence in all experimental animals, whereas administration of cisplatin solution led to bulky tumor recurrence. The viscous collagen matrix was able to contain the drug at high levels in the resection site for 7 days, leading to prolonged local exposure while sparing healthy tissues.
Gelatin
Gelatin is a natural, biocompatible, and non-toxic macromolecule obtained by denaturing of collagen derived from the skin, white connective tissue, and bones of animals (CitationNezhadi et al., 2009). Concerns exist over transmittance of pathogens such as prions that survive the high temperatures required for the denaturation process. As such, synthetic or plant-derived collagen is often favorable in biomedical applications (CitationOlsen et al., 2003).
CitationKonishi et al. (2003) have studied a gelatin hydrogel for localized cisplatin delivery, which was evaluated in a subcutaneous model of fibrosarcoma in mice. The hydrogel was injected directly under the tumor mass. The localized release of cisplatin over 14 days resulted in a significant anti-tumor effect and improved survival, while eliminating the undesirable side-effects associated with systemic cisplatin administration. CitationHanes et al. (2001) developed injectable gelatin-based microspheres for the long-term localized delivery of bioactive interleukin-2 (IL-2) for anti-tumor immunotherapy. More specifically, IL-2 was encapsulated into gelatin and chondroitin-6-sulfate using an aqueous-based complex coacervation technique. Anti-tumor efficacy of this system was studied in primary (9L gliosarcoma) and metastatic (B16-F10 melanoma) brain tumor models in rats and mice, respectively, as well as a murine liver tumor model (CT26 carcinoma). Release of IL-2 from the gelatin microspheres occurred over 2 weeks in vitro and significant levels were seen for up to 21 days in vivo. The gelatin IL-2 microspheres injected intra-tumorally were more effective in protecting animals challenged with fatal tumor doses in the brain and the liver than placebo or autologous tumor cells genetically engineered to secrete IL-2. Localized delivery of a gelatine-based doxorubicin formulation was also achieved by CitationStuart et al. (1993) using a chemoembolization technique. This formulation was injected in hepatic arteries closest to the tumors, which were catheterized so that the formulation would remain localized. The 69% overall response rate as a result of treatment was significant, although changes in disease-free survival times were not achieved.
Synthetic polymers
As synthetic polymers can be tailor-made or modified to provide desirable mechanical and chemical properties for specific applications, they are commonly used in the development of novel drug delivery systems (CitationGhosh, 2004). Importantly, unlike natural polymers, synthetic polymers pose very little risk of immunogenicity and disease transmission. On the other hand, due to their synthetic nature, these polymers often lead to inflammatory reactions, low clearance rates, and localized drops in pH due to acidic degradation products (CitationLee & Shin, 2007). However, formulations based on synthetic polymers for localized chemotherapy have reached clinical use as discussed below, which demonstrates that the benefits associated with these polymers outweigh their disadvantages.
Polyesters
Polyester-based polymers are the most widely explored class of biodegradable polymers for drug delivery applications, with poly(lactic acid-co-glycolic acid) (PLGA) alone being associated with more than 500 patents (CitationAnderson & Shive, 1997; CitationPillai & Panchagnula, 2001). The majority of approved pharmaceutical implants that are commercially available are based on PLGA. While these polymers have been criticized for acidic degradation products that can cause local irritation and instability of the drugs being delivered (CitationTaylor et al., 1994; CitationAthanasiou et al., 1996; CitationFu et al., 2000), polyester-based polymers such as PLGA are considered biocompatible and biodegradable, and are therefore attractive candidates for use in drug delivery systems.
PLGA has been used to develop a wide range of localized delivery systems, including microparticles, pastes, and implants. As many drugs and their vehicles are not able to penetrate the blood–brain barrier, CitationLee et al. (2005) developed a wafer formulation for the treatment of gliosarcoma. The wafer was prepared by compressing mixtures of PLGA and 1,3-bis(2-chloroethyl)-1-nitrosourea (carmustine, also known as BCNU), which released over a period of 7 days. Implantation adjacent to the tumor site was efficacious in a subcutaneous xenograft model of gliosarcoma. Since development of BCNU resistance in the brain is a concern, a paclitaxel-loaded thin PLGA implant was developed by CitationRanganath and Wang (2008). The authors claim that this thin implant provides a greater surface area-to-volume ratio than wafers, resulting in a more favorable drug release profile. Indeed, results show a low initial burst release of paclitaxel followed by a constant and prolonged release for over 80 days. A 30-day study in a subcutaneous tumor model showed greater tumor inhibition in animals treated with the paclitaxel-loaded implant than in animals treated intra-tumorally with the commercially available formulation of paclitaxel (Taxol®).
CitationAzouz et al. (2008) have investigated a PLGA microsphere formulation for paclitaxel delivery. In this study, PLGA microspheres loaded with paclitaxel were found to successfully inhibit local tumor growth after local injection following lung tumor resection. A large advantage of microspheres over implants is injectability, however there is a disadvantage in that there is a great potential for rapid migration to other tissues and into the systemic circulation. A solution to this is the development of injectable systems which can form a gel in situ. CitationD’Souza et al. (2006) developed an injectable PLGA gel capable of releasing bleomycin over 30 days, which significantly inhibited tumor growth in a murine intra-dermal model of fibrosarcoma.
An injectable PLGA drug delivery implant for use in the treatment of head and neck cancers has been studied by CitationDesai et al. (2008). PLGA millicylinders were used to formulate N-acetylcysteine, a drug that inhibits extracellular matrix degradation, thereby not allowing tumor progression. The millicylinders were prepared by slowly extruding a solution of drug and polymer through a syringe and into silicone tubing, which was dried and cut to extract solid millicylinders. Continuous drug release was achieved for over 1 month, although efficacy has not yet been tested in vivo. CitationWeinberg et al. (2007) formulated doxorubicin-loaded PLGA millirods for the treatment of liver cancer. PLGA microspheres were mixed with doxorubicin/NaCl powder using a mortar and pestle, and the mixture was packed and compressed to form millirods. Intra-tumoral treatment proved to be efficacious in a liver cancer model in rabbits. Necrosis could be detected in the tumors from the site of implantation, indicating that the prolonged 8-day release of doxorubicin caused damage to tumors while sparing the healthy liver tissue surrounding them.
Polyanhydrides
Like polyesters, polyanhydrides are a class of synthetic polymers widely studied for drug delivery applications. A significant advantage of polyanhydrides over polyesters is that they degrade into metabolites that are not cytotoxic or inflammatory (CitationKumar et al., 2002). These highly biocompatible polymers can be manipulated to yield drug release from days to months (CitationJain et al., 2005). The most successful application of polyanhydrides in localized cancer therapy is the FDA-approved polyanhydride poly(1,3-bis-(p-carboxyphenoxy propane)-co-(sebacic anhydride), or P(CPP-SA), for drug delivery in brain cancer (CitationWang et al., 2002). The commercially available formulation Gliadel® employs P(CPP-SA) in the form of a dime-sized wafer that is implanted at the site of tumor resection and gradually releases BCNU (CitationJain et al., 2005). This system is advantageous over the BCNU-PLGA wafer, as PLGA is known to elicit inflammatory reactions (CitationHickey et al., 2002; CitationFulzele et al., 2003; CitationGrayson et al., 2004). Clinical trials have shown that when the wafer is inserted at the tumor resection site, it provides continuous drug release for 7–10 days post-implantation, and survival is prolonged in patients with recurrent malignant glioma (CitationJain et al., 2008).
Many localized delivery systems that have been evaluated pre-clinically have been formed from P(CPP-SA). For example, CitationBerrada et al. (2002) used this polymer to deliver 5-fluorouracil intra-tumorally. The polymer was mixed with drug, ground into a fine powder, heated, and extruded to form rods, which were implanted via a trocar into fibrosarcoma tumors established subcutaneously in mice. Although the entire drug load was released within 3 days, with half released within 24 h, tumor growth inhibition resulting from intra-tumoral implantation was significantly greater than that resulting from systemic administration of 5-fluorouracil alone. P(CPP-SA) was also used by CitationStorm et al. (2002) to develop a localized delivery system for camptothecin, a very potent anti-cancer agent that has limited use due to high systemic toxicity. Drug-containing polymer discs were implanted in the brains of rats inoculated with gliosarcoma cells. Discs loaded with camptothecin resulted in an impressive median survival of 69 days, while control non-treated animals had a median survival of 17 days. The authors detected no systemic toxicity as a result of the treatment. CitationShikani and Domb (2000) evaluated the in vitro and in vivo efficacy of four anti-cancer agents (cisplatin, fluorouracil, methotrexate, and paclitaxel) loaded into implantable disks formed from a copolymer of fatty acid dimmer (FAD) and sebacic acid (SA), poly(FAD:SA), for localized delivery in the treatment of head and neck cancer. Efficacy of the cisplatin-poly(FAD:SA) disc was evaluated in a murine xenograft model of human mouth carcinoma, in which the polymer disc was implanted adjacent to the subcutaneous tumor. Tumor growth delay was doubled compared to animals receiving intravenous doses of cisplatin. In addition, the localized delivery strategy resulted in a reduction in systemic toxicity, with LD50 values 4-times greater than systemic administration. Polyanhydride polymers were used by CitationHsu et al. (2005) to explore the concept of multimodal therapy. The polymers were loaded with the anti-angiogenic drug adriamycin and were compressed to form disc-shaped implants. The discs were implanted intra-cranially in a gliosarcoma tumor model in rats after intra-cranial administration of gelatin microspheres loaded with interleukin-2 (IL-2). The administration of IL-2 leads to a strong immune reaction that eventually leads to necrosis of tumor cells. This action, coupled with the inhibition of angiogenesis, led to a median survival of 53 days, which is significantly greater than the median survival times of 30 and 39 days seen in rats treated with adriamycin-loaded polyanhydride polymer or IL-2 gelatin microspheres monotherapy, respectively. A very similar approach was adopted by CitationRhines et al. (2002), whereby IL-2-loaded gelatin microspheres were injected intra-cranially in a gliosarcoma model in rats, and polyanhydride polymer wafers were used to deliver BCNU. As was done by CitationHsu et al. (2005), the wafers were implanted 5 days after administration of the microspheres. The synergetic effect of immunotherapy and chemotherapy was evident, as median survival of animals treated with combination therapy was ∼ 2-fold greater than monotherapy with either agent.
Challenges and concluding remarks
As a dominant killer in the developing world, cancer is one of the most difficult health challenges of our time. Various treatment strategies have been explored to improve upon current systemic chemotherapy methods. Localized delivery of chemotherapeutics for solid tumors is one approach that has been considered in recent years. Biodegradable polymeric systems, both natural and synthetic, in the forms of microparticles, implantable wafers and films, injectable pastes, and gels have been investigated for this purpose due to their customizability. These formulations must be further pursued into clinical development, as they have shown great promise pre-clinically. Synthetic polymers have reached clinical use for localized delivery in cancer therapy. The success of these polymers is attributed to the high level of control in their production and customization, and minimal risk of disease transmission, an issue that poses great concern in the use of natural polymers. As illustrated by the various examples cited in this review, natural polymers show incredible potential as localized chemotherapeutic delivery systems due to their superior biocompatibility, biodegradability, and lack of immunogenicity, properties that are attributable to the presence of these polymers in living organisms, including the human body. Unfortunately, the fact that these polymers come from natural sources poses many challenges that have hindered their clinical use in this application, including concerns over pathogen transmission, purity, stability following sterilization, batch-to-batch variations, and immunogenicity. Once more suitable production protocols have been developed to resolve these issues it is highly plausible that the advantages of natural polymers could make them superior to synthetic polymers in localized chemotherapy.
As a whole, localized chemotherapy still does not have widespread clinical use, since some concerns still need to be addressed. One challenge of localized drug delivery using polymers is the lack of control in drug distribution. Intravenously delivered chemotherapeutics must overcome barriers including the vasculature wall, and hypoxic tumor regions distant from vasculature may not be exposed to adequate amounts of drug. On the other hand, drug penetration following localized drug delivery is hindered by the extravascular compartment of tumor tissue, which limits drug diffusion to within only 3 mm of the site of origin (CitationSawyer et al., 2006). Drug diffusion from a polymer matrix depends on the drug gradient in the environment and the tumor’s interstitial fluid pressure, which varies greatly; as a consequence, the drug distribution profile within the tumor becomes somewhat unpredictable. The effectiveness of the drug is compromised if it cannot reach some of the clonogenic cells within a tumor (CitationMinchinton & Tannock, 2006). However, continuous drug release from a polymer matrix allows for the elimination of superficial cells, allowing the drug to reach inner cell layers. The key determinant of overall efficacy is whether chemotherapy eliminates cell layers at a greater rate than tumor cell proliferation. To abrogate the problem of drug penetration, CitationExner and Saidel (2008) suggest that in situ forming implants should be combined with tumor thermal ablation. In this way, the implant serves to attack residual disease that was not affected by ablation. Localized chemotherapy could also be combined with low-dose systemic administration to increase drug distribution within the tumors.
A great contributor to high interstitial fluid pressure within a tumor is the disorganization and leakiness of existing vasculature (CitationAu et al., 2001). The administration of anti-angiogenic agents has been shown to not only prevent new blood vessel formation, but also normalize existing blood vessels. This reduces vasculature leakiness, thereby reducing interstitial fluid pressure and allowing greater penetration of drugs into the tumor (CitationExner & Saidel, 2008). In addition, the prevention of new blood vessel formation increases drug retention within the tumor.
Further issues hindering the clinical use of localized chemotherapy include the inability to reach metastatic sites and difficulties in accurate placement during implantation. A possible solution for treating metastatic sites is the use of low-dose systemic chemotherapy in combination with localized chemotherapy. Alternatively, the amount of drug that enters the systemic circulation from the delivery system may be enough to treat metastatic sites, especially if the delivery system has been designed for prolonged release. Although plasma drug levels may be significantly lower than levels resulting from intravenous chemotherapy, prolonged exposure of low-dose chemotherapy has been shown to be clinically beneficial (CitationVassileva et al., 2007; CitationZahedi et al., 2009). In terms of accurate placement of the delivery system, imaging modalities have recently proven to be valuable in non-invasively assisting intra-tumoral and peri-tumoral implantation (CitationExner & Saidel, 2008).
Overall, the principal advantages of localized systems can be attributed to high local drug concentrations at the tumor site for extended periods of time while maintaining low systemic drug exposure. This not only results in higher therapeutic efficacy, lower toxicity, and lower acquired drug resistance (CitationHo et al., 2006), but also reduces the need for repeat chemotherapeutic administrations, increasing patience compliance and improving quality of life. Further research is necessary to establish methods for accurate and non-invasive implantation into desired sites, means to reach metastatic sites, and to enhance tumor drug penetration upon delivery from polymeric implants. Once these obstacles are overcome, localized chemotherapy via polymeric systems has the potential to greatly improve therapeutic efficacy and toxicity profiles in the treatment of solid tumors.
Declaration of interest
Work in the authors’ laboratories is supported by a research grant obtained from the Canadian Cancer Society, Ontario Division. R. De Souza and P. Zahedi are grateful to the Natural Sciences and Engineering Research Council for post-graduate scholarships. The authors report no conflicts of interest. The authors alone are responsible for the content and writing of the paper.
References
- Abe, T., Sakane, M., Ikoma, T., Kobayashi, M., Nakamura, S., Ochiai, N. (2008). Intraosseous delivery of paclitaxel-loaded hydroxyapatite-alginate composite beads delaying paralysis caused by metastatic spine cancer in rats. J Neurosurg Spine. 9:502–10.
- Al-Ghananeem, A.M., Malkawi, A.H., Muammer, Y.M., Balko, J.M., Black, E.P., Mourad, W., Romond, E. (2009). Intratumoral delivery of paclitaxel in solid tumor from biodegradable hyaluronan nanoparticle formulations. AAPS Pharmscitech. 10:410–7.
- Almond, B., Hadba, A., Freeman, S., Cuevas, B., York, A., Detrisac, C., Goldberg, E.P. (2003). Efficacy of mitoxantrone-loaded albumin microspheres for intratumoral chemotherapy of breast cancer. J Contr Rel. 91:147–55.
- Anderson, J.M., Shive, M.S. (1997). Biodegradation and biocompatibility of PLA and PLGA microspheres. Adv Drug Deliv Rev. 28:5–24.
- Armstrong, D.K., Fleming, G.F., Markman, M., Bailey, H.H. (2006). A phase I trial of intraperitoneal sustained-release paclitaxel microspheres (Paclimer((R))) in recurrent ovarian cancer: a Gynecologic Oncology Group study. Gynecol Oncol. 103:391–6.
- Athanasiou, K.A., Niederauer, G.G., Agrawal, C.M. (1996). Sterilization, toxicity, biocompatibility and clinical applications of polylactic acid polyglycolic acid copolymers. Biomaterials. 17:93–102.
- Attenello, F.J., Mukherjee, D., Datoo, G., Mcgirt, M.J., Bohan, E., Weingart, J.D., Olivi, A., Quinones-Hinojosa, A., Brem, H. (2008). Use of Gliadel (BCNU) wafer in the surgical treatment of malignant glioma: a 10-year institutional experience. Ann Surg Oncol. 15:2887–93.
- Au, J.L.S., Jang, S.H., Zheng, J., Chen, C.T., Song, S., Hu, L., Wientjes,M.G. (2001). Determinants of drug delivery and transport to solid tumors. J Contr Rel. 74:31–46.
- Azouz, S.M., Walpole, J., Amirifeli, S., Taylor, K.N., Grinstaff, M.W., Colson, Y.L. (2008). Prevention of local tumor growth with paclitaxel-loaded microspheres. J Thorac Cardiovasc Surg. 135:1014–21.
- Berrada, M., Serreqi, A., Dabbarh, F., Owusu, A., Gupta, A., Lehnert,S. (2005). A novel non-toxic camtothecin formulation for cancer chemotherapy. Biomaterials. 26:2115–20.
- Berrada, M., Yang, Z., Lehnert, S. (2002). Tumor treatment by sustained intratumoral release of 5-fluorouracil: effects of drug alone and in combined treatments. Int J Radiat Oncol Biol Phys. 54:1550–7.
- Bouhadir, K.H., Alsberg, E., Mooney, D.J. (2001). Hydrogels for combination delivery of antineoplastic agents. Biomaterials. 22:2625–33.
- Chourasia, M.K., Jain, S.K. (2004). Polysaccharides for colon targeted drug delivery. Drug Deliv. 11:129–48.
- D’Souza, R., Mutalik, S., Udupa, N. (2006). In vitro and in vivo preparation evaluations of bleomycin implants and microspheres prepared with DL-poly(lactide-co-glycolide). Drug Dev Ind Pharm. 32:175–84.
- Davidson, B.S., Izzo, F., Cromeens, D.M., Stephens, L.C., Siddik, Z.H., Curley, S.A. (1995). Collagen matrix cisplatin prevents local tumor growth after margin-positive resection. J Surg Res. 58:618–24.
- De Souza, R., Zahedi, P., Allen, C., Piquette-Miller, M. (2009). Biocompatibility of injectable chitosan-phospholipid implant systems. Biomaterials. 30:3818–24.
- Desai, K.G., Mallery, S.R., Schwendeman, S.P. (2008). Formulation and characterization of injectable poly(DL-lactide-co-glycolide) implants loaded with n-acetylcysteine, a MMP inhibitor. Pharm Res. 25586–597.
- Dodane, V., Vilivalam, V.D. (1998). Pharmaceutical applications of chitosan. Pharm Sci Technol To. 1:246–53.
- Elstad, N.L., Fowers, K.D. (2009). OncoGel (ReGel/paclitaxel)—clinical applications for a novel paclitaxel delivery system. Adv Drug Deliv Rev. 61:785–94.
- Exner, A.A., Saidel, G.M. (2008). Drug-eluting polymer implants in cancer therapy. Expert Opin Drug Deliv. 5:775–88.
- Fraser, J.R.E., Laurent, T.C., Laurent, U.B.G. (1997). Hyaluronan: its nature, distribution, functions and turnover. J Intern Med. 242:27–33.
- Friess, W. (1998). Collagen—biomaterial for drug delivery. Eur J Pharm Biopharm. 45:113–36.
- Fu, K., Pack, D.W., Klibanov, A.M., Langer, R. (2000). Visual evidence of acidic environment within degrading poly(lactic-co-glycolic acid) (PLGA) microspheres. Pharm Res. 17:100–6.
- Fulzele, S.V., Satturwar, P.M., Dorle, A.K. (2003). Study of the biodegradation and in vivo biocompatibility of novel biomaterials. Eur J Pharm Sci. 20:53–61.
- Fung, L.K., Saltzman, W.M. (1997). Polymeric implants for cancer chemotherapy. Adv Drug Deliv Rev. 26:209–30.
- Gasparini, G. (2001). Metronomic scheduling: the future of chemotherapy? Lancet Oncol. 2:733–40.
- Gelse, K., Poschl, E., Aigner, T. (2003). Collagens—structure, function, and biosynthesis. Adv Drug Deliv Rev. 55:1531–46.
- Ghosh, S. (2004). Recent research and development in synthetic polymer-based drug delivery systems. J Chem Res S. 241–6.
- Grant, J., Blicker, M., Piquette-Miller, M., Allen, C. (2005). Hybrid films from blends of chitosan and egg phosphatidylcholine for localized delivery of paclitaxel. J Pharm Sci. 94:1512–27.
- Grant, J., Lee, H., Soo, P.L., Cho, J., Piquette-Miller, M., Allen, C. (2008). Influence of molecular organization and interactions on drug release for an injectable polymer-lipid blend. Int J Pharm. 360:83–90.
- Grayson, A.C.R., Voskerician, G., Lynn, A., Anderson, J.M., Cima, M.J., Langer, R. (2004). Differential degradation rates in vivo and in vitro of biocompatible poly(lactic acid) and poly(glycolic acid) homo- and co-polymers for a polymeric drug-delivery microchip. J Biomater Sci Polym Ed. 15:1281–304.
- Han, H.D., Song, C.K., Park, Y.S., Noh, K.H., Kim, J.H., Hwang, T., Kim,T.W., Shin, B.C. (2008). A chitosan hydrogel-based cancer drug delivery system exhibits synergistic antitumor effects by combining with a vaccinia viral vaccine. Int J Pharm. 350:27–34.
- Hanes, J., Sills, A., Zhao, Z., Suh, K.W., Tyler, B., Dimeco, F., Brat, D., Choti, M., Leong, K., Pardoll, D., Brem, H. (2001). Controlled local delivery of interleukin-2 by biodegradable polymers protects animals from experimental brain tumors and liver tumors. Pharm Res. 18:899–906.
- Harper, E., Dang, W.B., Lapidus, R.G., Garver, R.I. (1999). Enhanced efficacy of a novel controlled release paclitaxel formulation (PACLIMER delivery system) for local-regional therapy of lung cancer tumor nodules in mice. Clin Cancer Res. 5:4242–8.
- Hawe, A., Friess, W. (2007). Formulation development for hydrophobic therapeutic proteins. Pharm Dev Technol. 12:223–37.
- Hickey, T., Kreutzer, D., Burgess, D.J., Moussy, F. (2002). In vivo evaluation of a dexamethasone/PLGA microsphere system designed to suppress the inflammatory tissue response to implantable medical devices. J Biomed Mater Res. 61:180–7.
- Ho, E.A., Lim Soo, P., Allen, C., Piquette-Miller, M. (2006). Impact of intraperitoneal, sustained delivery of paclitaxel on the expression of P-glycoprotein in ovarian tumors. J Contr Rel. 117:20–7.
- Ho, E.A., Vassileva, V., Allen, C., Piquette-Miller, M. (2005). In vitro and in vivo characterization of a novel biocompatible polymer-lipid implant system for the sustained delivery of paclitaxel. J Contr Rel. 104:181–91.
- Hsu, W., Lesniak, M., Tyler, B., Brem, H. (2005). Local delivery of interleukin-2 and adriamycin is synergistic in the treatment of experimental malignant glioma. J Neuro-Oncol. 74:135–40.
- Jagur-Grodzinski, J. (2009). Polymers for targeted and/or sustained drug delivery. Polym Adv Technol. 20:595–606.
- Jain, J.P., Chitkara, D., Kumar, N. (2008). Polyanhydrides as localized drug delivery carrier: an update. Expert Opin Drug Del. 5:889–907.
- Jain, J.P., Modi, S., Domb, A.J., Kumar, N. (2005). Role of polyanhydrides as localized drug carriers. J Contr Rel. 103:541–63.
- Jang, S.H., Wientjes, M.G., Lu, D., Au, J.L.S. (2003). Drug delivery and transport to solid tumors. Pharm Res. 20:1337–50.
- Kakinoki, S., Taguchi, T. (2007). Antitumor effect of an injectable in-situ forming drug delivery system composed of a novel tissue adhesive containing doxorubicin hydrochloride. Eur J Pharm Biopharm. 67:676–81.
- Kean, T., Thanou, M. (2010). Biodegradation, biodistribution and toxicity of chitosan. Adv Drug Deliv Rev. 62:3–11.
- Kim, S., Kim, J.H., Jeon, O., Kwon, I.C., Park, K. (2009). Engineered polymers for advanced drug delivery. Eur J Pharm Biopharm. 71:420–30.
- Konishi, M., Tabata, Y., Kariya, M., Suzuki, A., Mandai, M., Nanbu, K., Takakura, K., Fujii, S. (2003). In vivo anti-tumor effect through the controlled release of cisplatin from biodegradable gelatin hydrogel. J Contr Rel. 92:301–13.
- Kumar, M.N.V.R. (2000). A review of chitin and chitosan applications. React Funct Polym. 377:76-84..
- Kumar, N., Langer, R.S., Domb, A.J. (2002). Polyanhydrides: an overview. Adv Drug Deliv Rev. 54:889–910.
- Lee, J.S., An, T.K., Chae, G.S., Jeong, J.K., Cho, S.H., Lee, H.B., Khang,G. (2005). Evaluation of in vitro and in vivo antitumor activity of BCNU-loaded PLGA wafer against 9L gliosarcoma. Eur J Pharm Biopharm. 59:169–75.
- Lee, S.H., Shin, H. (2007). Matrices and scaffolds for delivery of bioactive molecules in bone and cartilage tissue engineering. Adv Drug Deliv Rev. 59:339–59.
- Liao, Y.H., Jones, S.A., Forbes, B., Martin, G.P., Brown, M.B. (2005). Hyaluronan: pharmaceutical characterization and drug delivery. Drug Deliv. 12:327–42.
- Liu, Z.H., Jiao, Y.P., Wang, Y.F., Zhou, C.R., Zhang, Z.Y. (2008). Polysaccharides-based nanoparticles as drug delivery systems. Adv Drug Deliv Rev. 60:1650–62.
- Ma, X., Yu, H. (2006). Global burden of cancer. Yale J Biol Med. 79:85–94.
- Maeda, Y., Kimura, Y. (2004). Antitumor effects of various low-molecular-weight chitosans are due to increased natural killer activity of intestinal intraepithelial lymphocytes in sarcoma 180-bearing mice. J Nutr. 134:945–50.
- Malafaya, P.B., Silva, G.A., Reis, R.L. (2007). Natural-origin polymers as carriers and scaffolds for biomolecules and cell delivery in tissue engineering applications. Adv Drug Deliv Rev. 59:207–33.
- Mendez, C.M., Mcclain, C.J., Marsano, L.S. (2005). Albumin therapy in clinical practice. Nutr Clin Pract. 20:314–20.
- Minchinton, A.I., Tannock, I.F. (2006). Drug penetration in solid tumours. Nat Rev Cancer. 6:583–92.
- Mourya, V.K., Inamdar, N.N. (2008). Chitosan-modifications and applications: opportunities galore. React Funct Polym. 68:1013–51.
- Muzzarelli, R.A.A. (1993). Biochemical significance of exogenous chitins and chitosans in animals and patients. Carbohydr Polym. 20:7–16.
- Nair, L.S., Laurencin, C.T. (2007). Biodegradable polymers as biomaterials. Prog Polym Sci. 32:762–98.
- Nezhadi, S.H., Choong, P.F.M., Lotfipour, F., Dass, C.R. (2009). Gelatin-based delivery systems for cancer gene therapy. J Drug Target. 17:731–8.
- Obara, K., Ishiharab, M., Ozekia, Y., Ishizukaa, T., Hayashib, T., Nakamuraa, S., Saitod, Y., Yurad, T., Matsuib, T., Hattorib, H., Takaseb, B., Ishiharac, M., Kikuchib, M., Maeharaa, T. (2005). Controlled release of paclitaxel from photocrosslinked chitosan hydrogels and its subsequent effect on subcutaneous tumor growth in mice. J Contr Rel. 110:79–89.
- Olsen, D., Yang, C., Bodo, M., Chang, R., Leigh, S., Baez, J., Carmichael,D., Perala, M., Hamalainen, E., Jarvinen, M., Polarek, J. (2003). Recombinant collagen and gelatin for drug delivery. Adv Drug Deliv Rev. 55:1547–67.
- Park, H., Park, K. (1996). Biocompatibility issues of implantable drug delivery systems. Pharm Res. 13:1770–6.
- Patil, G.V. (2003). Biopolymer albumin for diagnosis and in drug delivery. Drug Dev Res. 58:219–47.
- Pillai, O., Panchagnula, R. (2001). Polymers in drug delivery. Curr Opin Chem Biol. 5:447–51.
- Qin, C.Q., Du, Y.M., Xiao, L., Li, Z., Gao, X.H. (2002). Enzymic preparation of water-soluble chitosan and their antitumor activity. Int J Biol Macromol. 31:111–7.
- Ranganath, S.H., Wang, C. (2008). Biodegradable microfiber implants delivering paclitaxel for post-surgical chemotherapy against malignant glioma. Biomaterials. 29:2996–3003.
- Read, T.A., Farhadi, M., Bjerkvig, R., Olsen, B.R., Rokstad, A.M., Huszthy, P.C., Vajkoczy, P. (2001). Intravital microscopy reveals novel antivascular and antitumor effects of endostatin delivered locally by alginate-encapsulated cells. Cancer Res. 61:6830–7.
- Rhines, L.D., Sampath, P., Dimeco, F., Lawson, H.C., Tyler, B., Hanes, J., Olivi, A., Brem, H. (2002). Local immunotherapy with interleukin-2 delivered from biodegradable polymer microspheres combined with interstitial chemotherapy: a novel treatment for experimental malignant glioma. Neurosurgery. 52:872–80.
- Rihova, B. (1996). Biocompatibility of biomaterials: hemocompatibility, immunocompatibility and biocompatibility of solid polymeric materials and soluble targetable polymeric carriers. Adv Drug Deliv Rev. 21:157–76.
- Ruel-Gariepy, E., Shive, M., Bichara, A., Berrada, M., Le Garrec, D., Chenite, A., Leroux, J.C. (2004). A thermosensitive chitosan-based hydrogel for the local delivery of paclitaxel. Eur J Pharm Biopharm. 57:53–63.
- Rugo, H.S. (2008). New treatments for metastatic breast cancer: mechanisms of action of nanoparticle albumin-bound taxanes. Community Oncol. 5:8–12.
- Sawyer, A.J., Piepmeier, J.M., Saltzman, W.M. (2006). New methods for direct delivery of chemotherapy for treating brain tumors. Yale J Biol Med. 79:141–52.
- Scharovsky, O.G., Mainetti, L.E., Rozados, V.R. (2009). Metronomic chemotherapy: changing the paradigm that more is better. Curr Oncol. 16:91–99.
- Shikani, A.H., Domb, A.J. (2000). Polymer chemotherapy for head and neck cancer. The Laryngoscope. 110:907–17.
- Shilpa, A., Agrawal, S.S., Ray, A.R. (2003). Controlled delivery of drugs from alginate matrix. J Macromol Sci-Pol R. 43:187–221.
- Sinha, V.R., Kumria, R. (2001). Polysaccharides in colon-specific drug delivery. Int J Pharm. 224:19–38.
- Storm, P.B., Moriarity, J.L., Tyler, B., Burger, P.C., Brem, H., Weingart,J. (2002). Polymer delivery of camptothecin against 9L gliosarcoma: release, distribution, and efficacy. J Neuro-Oncol. 56:209–17.
- Stuart, K., Stokes, K., Jenkins, R., Trey, C., Clouse, M. (1993). Treatment of hepatocellular carcinoma using doxorubicin/ethiodized oil/gelatin powder chemoembolization. Cancer. 72:3202–9.
- Ta, H.T., Dass, C.R., Larson, I., Choong, P.F.M., Dunstan, D.E. (2009). A chitosan-dipotassium orthophosphate hydrogel for the delivery of doxorubicin in the treatment of osteosarcoma. Biomaterials. 30:3605–13.
- Taylor, M.S., Daniels, A.U., Andriano, K.P., Heller, J. (1994). 6 Bioabsorbable polymers—in-vitro acute toxicity of accumulated degradation products. J Appl Biomater. 5:151–7.
- Tonnesen, H.H., Karlsen, J. (2002). Alginate in drug delivery systems. Drug Dev Ind Pharm. 28:621–30.
- Vassileva, V., Grant, J., De Souza, R., Allen, C., Piquette-Miller, M. (2007). Novel biocompatible intraperitoneal drug delivery system increases tolerability and therapeutic efficacy of paclitaxel in a human ovarian cancer xenograft model. Cancer Chemother Pharmacol. 60:907–14.
- Wallace, D.G., Rosenblatt, J. (2003). Collagen gel systems for sustained delivery and tissue engineering. Adv Drug Deliv Rev. 55:1631–49.
- Wang, P.P., Frazier, J., Brem, H. (2002). Local drug delivery to the brain. Adv Drug Deliv Rev. 54:987–1013.
- Weinberg, B.D., Ai, H., Blanco, E., Anderson, J.M., Gao, J.M. (2007). Antitumor efficacy and local distribution of doxorubicin via intratumoral delivery from polymer millirods. J Biomed Mater Res Part A. 81A:161–70.
- Westphal, M., Hilt, D.C., Bortey, E., Delavault, P., Olivares, R., Warnke,P.C., Whittle, I.R., Jaaskelainen, J., Ram, Z. (2003). A phase 3 trial of local chemotherapy with biodegradable carmustine (BCNU) wafers (Gliadel wafers) in patients with primary malignant glioma. Neuro-Oncology. 5:79–88.
- Yadav, A.K., Mishra, P., Agrawal, G.P. (2008). An insight on hyaluronic acid in drug targeting and drug delivery. J Drug Target. 16:91–107.
- Ye, S.-F., Hou, Z.-Q., Zhou, Z.-M., Zhong, L.-M., Zhang, Q.-Q. (2008). Intratumoral chemotherapy with an implantable collagen film impregnated with epirubicin-loaded polylactic acid microspheres inhibits tumor growth in hepatocellular carcinoma xenografts. Chin J Biochem Eng. 27:590–6.
- Zahedi, P., De Souza, R., Piquette-Miller, M., Allen, C. (2009). Chitosan-phospholipid blend for sustained and localized delivery of docetaxel to the peritoneal cavity. Int J Pharm.
- Zheng, J.P., Wang, C.Z., Wang, X.X., Wang, H.Y., Zhuang, H., De Yao, K. (2007). Preparation of biornimetic three-dimensional gelatin/montmorillonite-chitosan scaffold for tissue engineering. React Funct Polym. 67:780–8.