Abstract
The objective of this study was to design a novel artificial bone scaffold for therapy and prevention of refractory bacterial infection. Porous β-tricalcium phosphate (β-TCP) scaffold was combined with liposomal gentamicin (GS) to form a novel complex drug carrier. The liposome combined β-TCP scaffold (LCS) was characterized for its liposome binding rate, drug loading, and micromorphology. The anti-biofilm activity of LCS was evaluated by Staphylococcus aureus biofilm in vitro. The drug release from LCS was recognized as an initial high dose of liposomal GS released from the matrix and a further sustained release of free GS from the liposome, respectively, and it is an ideal release pattern for treatment and prevention of post-operative osteomyelitis. The release kinetics was influenced by variation of particle size of liposome. LCS displayed a potential anti-biofilm activity even in the lowest GS concentration (2.5 μg/mL), and the regrowth time was extended from 5.0 h to 9.5 h. At a higher dosage range, the highest anti-biofilm activity was achieved by LCS with liposomal particle size of 800 nm. In conclusion, the development of LCS showed a new pathway for controlled delivery of liposomal antibiotics for treatment of osteomyelitis caused by persistent bacterial infection.
Introduction
Osteomyelitis is a refractory condition that may occur after bone surgery, potentially leading to amputation or even death; treatment often requires multiple surgical interventions and local or systemic antibiotic therapy (CitationCosterton et al., 1999; CitationGitelis & Brebach, 2002). It involves the persistent bacterial infections which are commonly caused by gram-positive coccus. In the internal environment of bone, antibiotics are difficult to reach the efficient level. In some cases the bacterial proliferation could form biofilms which have a strong protective effect on the bacteria, and the drug-resistant plasmid can be easily transmitted and exchanged among the bacteria (CitationCosterton et al., 1999). As a result, in osteomyelitis growth of bacteria almost always leads to a significant decrease in susceptibility to anti-microbial agents compared with cultures grown in suspension. Therefore, seeking for effective treatment of osteomyelitis by novel drug delivery technologies is needed.
Beta-tricalcium phosphate (β-TCP) is a successfully used biodegradable scaffold for bone substitute with good biocompatibility. Beta-TCP also has the ability to induce bone formation (CitationGrynpas et al., 2002). For its biodegradable and porous properties, calcium polyphosphate scaffolds can be used as carriers of antibiotics for treatment of bacteria-related osteomyelitis (CitationWalenkamp, 2001). They may result in a high level of drug release and obviate the need for removal; they are gradually replaced by ingrowing tissue. Furthermore, secondary release of the antibiotic may occur during the degradation phase of the carrier, this could increase the anti-microbial efficacy compared to non-biodegradable carriers (CitationHumphrey et al., 1998).
Liposome is a biocompatible and biodegradable drug carrier for sustained and targeted delivery of various drugs including antibiotics (CitationSchiffelers et al., 2001). It can modify the biopharmaceutical characters such as absorption and distribution of the drug. It has been proved to be an effective way to enhance the efficacy of antibiotics and to reduce their undesirable effects by incorporation of anti-microbial agents into liposomes. Liposomes have been found to be of use in the delivery of antibiotics to biofilms of various bacteria immobilized on solid supports (CitationCatuogno & Jones, 2003; CitationKim & Jones, 2004; CitationTamilvanan et al., 2008). Liposomal systems have been widely studied, either to target antibiotics to the surface of bacterial biofilms, or by virtue of their property of being taken up cells of the reticuloendothelial system, to target antibiotics towards intracellular bacteria.
Gentamicin sulfate (GS) is a kind of aminoglycoside antibiotic and is the typical treatment for osteomyelitis caused by Gram-positive bacteria (CitationStallmann et al., 2006). The efficacy of aminoglycosides depends on their ability to reach specific bacterial targets in their active form without enzymatic attack. High dosages of aminoglycosides potentially carry drug toxicity risks. Liposomal encapsulation of GS is a hopeful strategy that helps to increase the therapeutic index of the antibiotics by increasing the concentration of the antibiotics at the site of infection and prolong the drug release time, as well as reducing the toxicity (CitationTang et al., 2009).
In this study we have investigated the effectiveness of delivery of GS by liposome combined β-TCP scaffold (LCS), with the aim of inhibiting the bacterial infection in the early stage of post-operative period which is a major cause of the refractory osteomyelitis.
Materials and methods
Materials
1, 2-Dipalmitoyl-sn-glycerol-phosphatidylcholine (DPPC) was supplied by Avanti Polar Lipids (Alabaster, AL, USA). Cholesterol was produced by Shanghai Guoyao Corp. Gentamicin sulfate (GS) was obtained from Asianchem Corp., (Nanjing, China). β-TCP scaffold was supplied by Bio-lu Corp., (Shanghai, China). Triton X-100 was a product of Sigma. All other reagents were of certified standard.
Methods
Preparation and characterization of GS liposomes
Liposome containing GS was prepared using a thin-film method (CitationRukholm et al., 2006). Briefly, a mixture of DPPC and cholesterol in the molar ratio of 3:1 was dissolved in a mixed solvent composed of methanol and chloroform (volume ratio 1:4). The lipid solution was dried in a round-bottom flask using a rotatory evaporator (Model RE 52, Shanghai, China), at 40°C under reduced pressure, to form a dry lipid film. The lipid film was dried in vacuum for 12 h. Afterwards, the lipid film was hydrated with a 2% (w/v) GS solution at 55°C under magnetic stirring to form a homogeneous suspension. The suspension was applied to a probe-type sonicator (Scientz JY92-2D, Ningbo, China) for homogenization and reduction of the particle size, under different sonicating power and time. For separation of the unencapsulated GS, liposome was ultracentrifugated under 60,000 g and 4°C for 2 h. The precipitate was re-suspended in an appropriate amount of deionized water. The encapsulation efficiency (EE) of liposome was calculated by determining the unencapsulated GS concentration in the supernatant after ultracentrifugation using an AxSym System (Abbott Laboratories, IL, USA), and compared with the initial GS amount added to the formulation.
The particle size of GS liposomes prepared above was analyzed in a Nano-ZS zeta sizer (Malvern Instruments, Malvern, UK) at 25°C. The liposomes were diluted with distilled water prior to analysis, and each measurement was carried out in triplicate.
Preparation of liposome combined β-TCP scaffold (LCS)
Medical grade β-TCP scaffold (0.5 mm granules) was weighted and immersed in GS liposome sample and sonicated in a bath-type sonicator (KQ-100DB, Kunshan, China) for 20 min, and further maintained in reduced pressure (0.09 MPa) for another 20 min to allow the liposome permeating into the porous scaffold. Afterwards, samples were transferred into glass bottles and freeze-dried. For determination of the GS content in LCS, the granules were ground in 1% Triton X-100 solution and then sonicated for 10 min to completely release the liposomal GS. The resulting suspension was centrifuged at 4000 rpm for 10 min, and the GS content in the supernatant was determined by AxSym System.
Scanning electron microscopy
The morphology of LCS was examined by scanning electron microscopy (SEM). The freeze-dried scaffolds were fractured, then attached to metal stubs and coated with gold under vacuum. The morphology was observed by a scanning electron microscope (SSX-550, Shimadzu, Japan).
In vitro release study
For evaluating the release of liposomal GS from LCS, 0.2 g of the granules was introduced into a polyethylene tube with 1.0 mL of PBS solution (pH 7.4) as release medium and kept in a shaking water bath (ZS501-A, Liaoning, China) at 37°C, 30 rpm. At designated time intervals, the release medium was withdrawn and replaced with an equal volume of fresh medium. The withdrawn medium was ultracentrifugated under 60,000 g and 4°C for 2 h. Afterwards the GS content in the supernatant was analyzed by AxSym System. The sediment was added to 1.0 mL of 1% Triton X-100 solution and sonicated for 10 min for liposome rupturing, and then the liposomal GS content was analyzed.
Bacteriology and biofilm preparation
Staphylococcus aureus biofilm was incubated in vitro according to a previously reported method (CitationTamilvanan et al., 2008). A strain of Staphylococcus aureus (kindly gifted by Department of Microbiology and Immunology of Kunming Medical College) was planted to blood agar plates (composed of brain heart infusion, bacterial agar, defibrinated horse blood, and water) (CitationKim & Jones, 2004). After incubation at 37°C overnight, the bacteria were then transferred from the solid agar plate to Falcon tubes containing a nutrient broth (made by mixing brain heart infusion (3.7 g) and yeast extract powder (0.3 g) in 100 mL of water) using a sterile disposable plastic loop. The Falcon tubes were left overnight on an agitator at 37°C. The tubes were then centrifuged at 2000 rpm for 10 min. The supernatant was removed and the pellet washed with 20 mL sterile PBS. The process was repeated three times. The bacterial suspension was then diluted in PBS in order to give an optical density (OD) of 0.5 at 550 nm. Aliquots (200 μL) of the above bacterial suspension were planted into a 96-well flat-bottomed microtitre plate and incubated at room temperature for 18 h. Prior to use the supernatant was removed and the wells were washed three times with PBS.
Evaluation of anti-biofilm activity
Liposomal GS released from LCS were applied to the biofilms to assess its anti-biofilm activity. Briefly, after in vitro release for a certain interval as described earlier, the release medium was withdrawn, ultracentrifugated, and re-suspended to form an appropriate concentration, and aliquots of 200 μL were incubated with the biofilms for 1 h and then removed. As control, biofilms were exposed to GS aqueous solution and blank PBS, respectively, at identical overall drug concentrations. After exposure to test samples, the wells were washed with PBS three times to remove the free drug and the bacteria were re-dispersed in liquid growth medium to give an OD value of 0.1 at 550 nm. The OD of the bacteria suspension was measured by a DIAS Dynex plate reader under 630 nm at intervals of 30 min for an overall period of 24 h. The time necessary to reach the OD value of 0.5 (OD0.5) was recorded.
Statistical analysis
Experiments were run in triplicate per sample and the data were expressed as means ± standard deviation (SD). Statistical analysis was using one-way analysis of variance (ANOVA), using a statistical software SPSS 11.0. p-values < 0.05 were considered to be significant.
Results and discussion
Characterization of GS liposome
The particle size and EE of GS liposomes (GL) prepared is shown in . Liposomes with particle size varying from ∼ 100 nm to 5 μm were prepared for combination with β-TCP scaffold. The derivation of liposome particle size was obtained under different sonicating conditions, as are shown in . The average particle size of liposome decreased when the sonicating power raised or sonicating time prolonged. The EE of liposome slightly decreased with the decrease in particle size, because the inner aqueous phase of liposomes had a decrease in volume after particle size reduction and thereby the drug loading was reduced. In order to obtain an equivalent drug content, the volume of liposome samples were adjusted using distilled water.
Table 1. Particle size and EE of GS liposomes (GL) prepared under different sonicating conditions.
Characterization of LCS
Liposomes with different particle size (samples GL1∼5) were combined with β-TCP scaffold to form LCS. The GS content in LCS with different liposome particle size is shown in . The GS liposome formula GL1∼5 correspond to LCS1∼5, respectively. GS content reached the highest level in the case of LCS3, ∼ 7.05 mg/g, while GL1 resulted in the lowest GS level in LCS1, ∼ 4.95 mg/g. When there was derivation in EE of liposomes of different size, the liposome content (ML, mg/g) in LCS was also calculated, as follows:
where CL is lipid concentration in the liposome aqueous suspension (40 mg/mL) and CGS is GS concentration in aqueous phase (20 mg/mL) in the liposome preparation process. shows ML of different LCS samples, and it can indicate the effect of particle size on the liposome combination to LCS. The highest ML appeared in LCS3 and 2, which were 162.07 mg/g and 160.49 mg/g, respectively (no significant difference, p > 0.1). When the particle size reduced from 5 μm to 800 nm, the liposome combination efficiency increased. This might be because liposomes with smaller particle size can permeate into the porous structured scaffold more easily and sufficiently. Although the macro-pores of the β-TCP scaffold are big enough (∼ 100 μm) for liposome entry, there are also some pores that are much smaller and therefore lead to difficult permeation for larger particles. On the other hand, liposome with particle size of 110 nm showed reduced combination efficiency, which might be due to the lower mass of individual liposome vesicle.
shows the SEM morphology of LCS before (a) and after (b) combination with GS liposome. Before combination, β-TCP scaffold had a relative smooth surface with micropores of ∼ 100 μm (). In a more magnified view (), the specific morphology of the surface was demonstrated. There were ultra-fine pores on the surface of the scaffold. After loading with GS liposome, the morphology of surface of the scaffold changed (). It can be observed that liposome particles are adsorbed on the surface of scaffold. The vesicle structures of liposome can be observed in a magnified image ().This indicates that the liposome structure was well preserved in LCS after lyophilization.
Figure 3. SEM morphology of LCS before (a) and after (b) combination with GS liposome. (a1) The surface of β-TCP scaffold, and (a2) its enlarged image. After liposome combination, the surface morphology of LCS (b1) and more magnified image of GS liposome (indicated by arrows) combined on the scaffold surface (b2) were demonstrated.
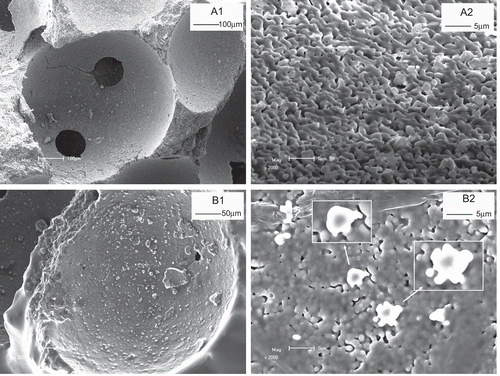
In vitro GS release from LCS
The in vitro release behaviors of LCS with different liposome formulations were investigated, as is shown in The release of GS form LCS was mainly in the form of liposome, rather than release as free drug. The cumulative release of free GS was only ∼ 5% in 24 h. This suggests that LCS has the potential to release and transport liposome encapsulated drug to target tissue. Theoretically, interception of the development of bacterial biofilm in the early stage can largely reduce the risk of refractory bone infections such as osteomyelitis. In this study LCS released a high dose of the liposomal antibiotic in the initial several hours and further induced a sustained release of free GS from the liposome. Therefore, LCS might be a hopeful drug delivery system with an ideal release pattern for treatment and prevention of post-operative osteomyelitis.
Figure 4. Release profiles of liposomal GS (a) and free GS (b) from LCSs formulated with different liposome particle size. The GS release percentage (ratio to the total GS content) was plotted vs time, each value represents the mean ± SD (n = 3).
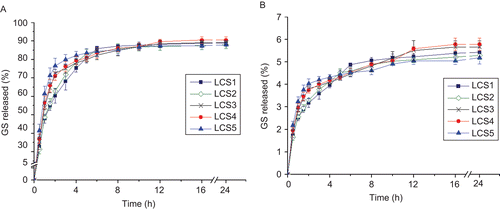
For liposomal GS, in the first hour the release profiles of various formulations were similarly in a fast release pattern. Afterward, the release decelerated and showed differences among groups. For comparison of release kinetics of the formulations, the time-release plots of liposomal GS from LCS were fitted by release models as shown in , and the fitting results are shown in .
According to correlation coefficient (R2), the release profiles of LCS with different liposome particle size all fitted best to Ritger-Peppas model. This model can be used to elucidate the release mechanism of formulations of different types. In the case of LCS, it can be regarded as a ‘slab-shaped’ formulation for its characteristic of multi-porosity. For the slab system, the release constant k has the limiting values of 0.50 in the case of Fickian diffusion (CitationRitger & Peppas, 1987). Therefore, the general release of liposomal GS from LCS can be regarded as mostly related to Fickian diffusion. According to , the release constant k had a descending trend with the increase of liposomal particle size. This suggests that there might be difference in the release mechanism of LCS between large vesicles and small vesicles.
Table 2. In vitro release models used to fit the release profiles of liposomal GS with different particle size from LCS.
Table 3. Fitting results of in vitro release of liposomal GS from LCS to various models.
In order to optimize the modeling and give a better understanding to the release mechanism, we selected two representative fractions from the release profile: from 0.5–2 h and from 2–6 h, in which two intervals over 80% of the drug was released. They were fitted by Ritger-Peppas model respectively, and the results were displayed in .
Table 4. Segmental modeling of release profiles of LCS in different intervals by Ritger-Peppas model.
In the segmental fitting, both of the two fractions from the release profiles complied with Ritger-Peppas model for each formulation. However, the trend of release constant k was diverse from the case of the gross fitting. As is shown in , in the interval of 0.5∼2 h, the release constant k of the five formulations were similar, and two of them (LCS2 and 3) exceeded the limiting values of 0.50 for Fickian diffusion. In the definition of Ritger-Peppas model the constant k in the range of 0.5∼1.0 was the mixed effect of Fickian diffusion and relaxation of the slab (CitationRitger & Peppas, 1987). In the case of LCS, this could be explained that in the initial stage of liposome release the relatively abundant liposome layer on the surface of the scaffold entered fast release through the macro-pores after hydration. This can be regarded as similarity of the drug release from the open structure of relaxed polymer chains. After this stage, the liposome release transferred into pure Fickian diffusion and lead to significant decrease in k. The decrease of k with the rising particle size can be explained as the larger particles had more steric hindrance when passing through the micropores in the scaffold.
Anti-biofilm activity evaluation
The anti-biofilm activity of LCS was investigated in vitro against Staphylococcus aureus biofilm. In order to simulate the different stages of liposomal GS release from LCS, the GS content was ranging from 2.5–800 μg/mL in the anti-biofilm study, and was compared with equivalent free GS solution. shows the effect of LCS3, which had the highest combination level with liposome GS, on the regrowth of bacteria of the biofilm. Even in the lowest GS concentration, LCS displayed a significant antibacterial activity compared with the control (p < 0.01). With the increase in GS concentration, its activity got to a higher level, but the difference between 400 μg/mL and 800 μg/mL was not significant (p > 0.05). In the case of free GS, no significant anti-biofilm activity appeared below 100 μg/mL, and it had lower activities than LCS at different concentrations. This might be because liposomes can accumulate around the biofilm by the interaction of lipid bilayer, in which case the drug can be retained as a liposomal form for a longer time before elimination, and provide a sustained drug release. On the other hand, liposomes can improve the drug penetration into the shielded biofilm by membrane fusion and increase the drug transportation towards the bacteria. This suggests that LCS is a hopeful tool to intercept the development of bacterial biofilm in the initial stage.
Figure 7. Effect of LCS released liposomal GS on the time necessary to reach OD0.5 in bacterial regrowth of Staphylococcus aureus biofilm, when taking free GS as control, n = 4, bars represents the SD.
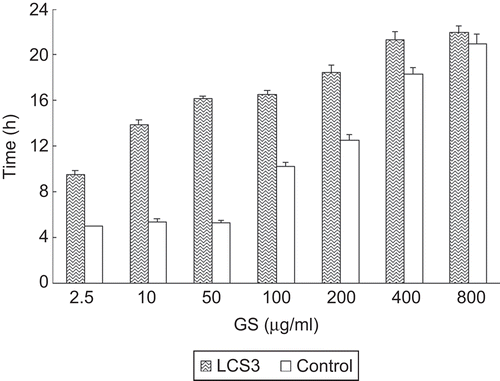
shows the comparison of inhibition on bacterial regrowth among LCS with different liposome particle size, in which the natural logarithm of particle size (φ) and liposomal GS concentration (C), respectively, were plotted with the time necessary to reach the maximum growth rate. In general, in the lower concentration range, LCS with smaller particles of liposome had greater activity compared with the larger particles. For example, at the lowest drug concentration (2.5 μg/mL for GS), LCS1 (particle size ∼ 100 nm) showed the highest inhibiting effect. This might be because the small liposome vesicles had larger surface area and were more facilitated to get contact with the surface of bacteria. On the other hand, at high concentrations the trend was different. At both 400 μg/mL and 800 μg/mL, the highest antibacterial activity appeared in LCS3, suggesting that liposomes in a specific particle range could transport drug more efficiently to the therapeutic targets.
Figure 8. Response surface diagrams showing the changing of bacterial biofilm regrowth after exposure to LCSs with different liposome particle size for various concentrations. z-axis is the time necessary to reach OD0.5 (h); x-axis is the natural logarithm of particle size (φ); y-axis is the natural logarithm of liposomal GS concentration (C).
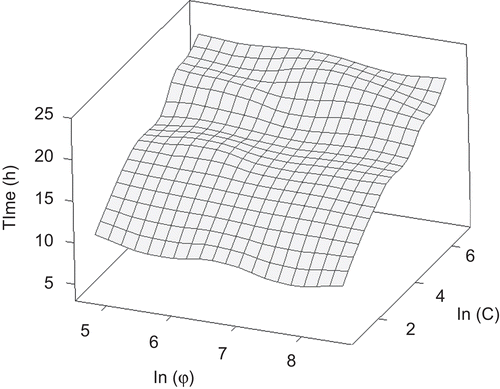
Conclusion
The porous β-TCP scaffolds were successfully combined with liposomes containing GS of different particle size. SEM morphology confirmed the complex structure and the binding rate of liposome was high enough to provide a potential drug delivery. The drug release from LCS can be recognized as an initial high dose of liposomal GS from the matrix and a further sustained release of free GS from the liposome, respectively, which is an ideal release pattern for treatment and prevention of post-operative osteomyelitis. The kinetics of the in vitro release showed identical characteristics among formulations and time segments, which is helpful for further understanding of this kind of release system. In the bacterial study, GS-LCS showed notable anti-biofilm activity compared with the free drug, and influences of liposomal particle size were also observed. The further potential of LCS system for anti-biofilm aimed drug delivery and its in vivo evaluation will be investigated in our undergoing studies.
Declaration of interest
The authors report no conflicts of interest. The authors alone are responsible for the content and writing of the paper.
References
- Catuogno, C., Jones, M.N. (2003). The antibacterial properties of solid supported liposomes on Streptococcus oralis biofilms. Int J Pharm. 257:125–40.
- Costerton, J.W., Stewart, P.S., Greenberg, E.P. (1999). Bacterial biofilms: a common cause of persistent infections. Science. 284:1318–22.
- Gitelis, S., Brebach, G.T. (2002). The treatment of chronic osteomyelitis with a biodegradable antibiotic-impregnated implant. J Orthopaed Surg. 10:53–60.
- Grynpas, M.D., Pilliar, R.M., Kandel, R.A., Renlund, R., Filiaggi, M., Dumitriu, M. (2002). Porous calcium polyphosphate scaffolds for bone substitute applications in vivo studies. Biomaterials. 23:2063–70.
- Humphrey, J.S., Mehta, S., Seaber, A.V., Vail, T.P. (1998). Pharmacokinetics of a degradable drug delivery system in bone. Clin Orthop Relat Res. 349:218–24.
- Kim, H.J., Jones, M.N. (2004). The delivery of benzyl penicillin to Staphylococcus aureus biofilm by use of liposome. J Liposome Res. 14:123–39.
- Ritger, P.L., Peppas, N.A. (1987). A simple equation for description of solute release I. Fickian and non-fickian release from non-swellable devices in the form of slabs, spheres, cylinders or discs. J Contr Rel. 5:23–36.
- Rukholm, G., Mugabe, C., Azghani, A., Omri, A. (2006). Antibacterial activity of liposomal gentamicin against Pseudomonas aeruginosa: a time–kill study. Int J Antimicrob Ag. 27:247–52.
- Schiffelers, R., Storm, G., Bakker-Woudenberg, I. (2001). Liposome-encapsulated aminoglycosides in pre-clinical and clinical studies. J Antimicrob Chemother. 48:333–44.
- Stallmann, H., Faber, C., Bronckers, A., Amerongen, A., Wuisman, P. (2006). In vitro gentamicin release from commercially available calcium-phosphate bone substitutes influence of carrier type on duration of the release profile. BMC Musculoskel Disord. 7:18–26.
- Tamilvanan, S., Venkateshan, N., Ludwig, N. (2008). The potential of lipid- and polymer-based drug delivery carriers for eradicating biofilm consortia on device-related nosocomial infections. J Contr Rel. 128:2–22.
- Tang, H., Xu, Y.Q., Zheng, T.E., Li, G., You, Y.G., Jiang, M.Y., Li, J., Ding, J. (2009). Treatment of osteomyelitis by liposomal gentamicin-impregnated calcium sulfate. Arch Orthop Trauma Surg. 129:1301–8.
- Walenkamp, G.H. (2001). Gentamicin PMMA beads and other local antibiotic carriers in two-stage revision of total knee infection: a review. J Chemother. 13: 66–72.