Abstract
The present investigation was aimed to develop and explore the prospective of engineered PLGA nanoparticles as vehicles for targeted delivery of 5-fluorouracil (5-FU). Nanoparticles of 5-FU-loaded hyaluronic acid-poly(ethylene glycol)-poly(lactide-co-glycolide) (HA-PEG-PLGA-FU) copolymer were prepared and characterized by FTIR, NMR, transmission electron microscopy, particle size analysis, DSC, and X-ray diffractometer measurement studies. The nanoparticulate formulation was evaluated for in vitro release, hemolytic toxicity, and hematological toxicity. Cytotoxicity studies were performed on Ehrlich ascites tumor (EAT) cell lines using MTT cell proliferation assay. Biodistribution studies of 99mTc labeled formulation were conducted on EAT-bearing mice. The in vivo tumor inhibition study was also performed after i.v. administration of HA-PEG-PLGA-FU nanoparticles. The HA conjugated formulation was found to be less hemolytic but more cytotoxic as compared to free drug. The hematological data suggested that HA-PEG-PLGA-FU formulation was less immunogenic compared to plain drug. The tissue distribution studies displayed that HA-PEG-PLGA-FU were able to deliver a higher concentration of 5-FU in the tumor mass. In addition, the HA-PEG-PLGA-FU nanoparticles reduced tumor volume significantly in comparison with 5-FU. Thus, it was concluded that the conjugation of HA imparts targetability to the formulation, and enhanced permeation and retention effect ruled out its access to the non-tumor tissues, at the same time favored selective entry in tumors, thereby reducing the side-effects both in vitro and in vivo.
Introduction
Tumor is a heterogenic, mutagenic architectural unit, a pathological state with properties which are still incompletely comprehended (CitationAgarwal et al., 2008a; Citationb). Targeting of anti-cancer bioactives at the tumor cells is desirable for two reasons: first, to maximize cytotoxic effect throughout the tumor growth phase during which the majority of the cells remains sensitive to pharmacotherapy and, secondly, to protect the surrounding healthy cells from exposure to the cytotoxic agents. In order to obtain better tumor regression, it is also desirable to maintain a steady infusion of the drug into the tumor interstitium.
Various novel drug carrier systems such as liposomes (CitationReddy et al., 1999), polymeric micelles (CitationNasongkla et al., 2004), nanoparticles (CitationXu et al., 2005), and nanoemulsions (CitationYuan Li et al., 2009) have been reported to accomplish the above objectives. Amongst the various drug delivery vehicles, polymeric nanoparticles have drawn major attention because of higher stability and the ability to be freeze-dried for long-term storage and be more chemically and physically stable (CitationLi et al., 2003). They offer suitability for industrial manufacture, and can be loaded with small molecules, proteins, or plasmid DNA with different specific methods (CitationPeracchia et al., 1997; CitationZambaux et al., 2001; CitationPanyam & Labhasetwar, 2003; CitationSwayam & Labhasetwar, 2004). Nanoparticles also prolong drug release (CitationPerez et al., 2001) and present the opportunity for multiple ligand coupling (CitationMohanraj & Chen, 2006; CitationAgarwal et al., 2008a). These multiple ligand coupled polymeric nanoparticles can be exploited to accomplish both controlled drug release and tumor targeting (CitationShenoy & Amiji, 2005).
In an attempt to promote spatial and temporal distribution of bioactive substance, the carrying vehicle should undergo controlled degradation, have entrapment efficiency, efficiently release a variety of pharmacological moieties, and promote easy coupling of ligands at controlled surface densities (CitationRatnam et al., 2003). This can be achieved by developing the new, novel polymeric nanoparticulate system that has properties like versatility in terms of the choice of the polymer core and possess a hydrophilic corona that would promote bypassing of the mononuclear phagocytic system and prevent degradation of either the ligand or the nanoparticles or provoke an uncontrolled release of the entrapped material (CitationHans et al., 2005). In the core-corona type nanoparticles, the core may facilitate high encapsulation, whereas PEG corona for ligand coupling has the advantage of offering a maximal accessibility for the ligand to interact with its target and to maintain a conformation close to that in solution (CitationYadav et al., 2007). Ligands can be covalently linked to the block copolymer containing PEG segments prior to nanoparticle formation by nanodeposition or solvent evaporation. Alternatively, they could be coupled to pre-formed nanoparticles possessing reactive groups at the PEG terminus (CitationGref et al., 2003).
It has been well established that nanocarriers can get concentrated preferentially in the tumor mass by virtue of the enhanced permeation and retention (EPR) mechanism. Once accumulated, they can act as a local drug depot depending upon the make-up of the carrier, thus providing a source for continuous supply of encapsulated therapeutic compound into tumor mass (CitationAgarwal et al., 2009). Active targeting can be accomplished exploiting specific interaction between receptors on the cell surface and tailoring ligands to the polymer backbone. Hence, active therapeutic agents loaded in the polymeric vehicles can be selectively transported to the tumor sites. The active approach consequently takes advantage of the EPR effect, but further increases the therapeutic index through receptor-mediated uptake by target cancer cells.
Hyaluronic acid (HA) is a naturally occurring glycosaminoglycan of alternating D-glucuronic acid (GlcUA) and N-acetyl-D-glucosamine (GlcNAc) units (CitationYun et al., 2004). HA facilitates receptor-mediated effects, via intracellular signaling pathways in which HA binds to, and is internalized by, receptors present on the surface of the extracellular matrix (CitationCulty et al., 1992). Numerous membrane-bound receptors viz, RHAMM and IVd4 that have affinity for HA have been identified (CitationWang et al., 1998; CitationNaor et al., 2002). Augmented levels of HA and enhanced expression of activated HA receptors CD44 and RHAMM on tumor cells suggests that exploiting interactions between HA and its receptors can serve to provide site-specific delivery and may promote a remarkable inhibition of tumor growth.
5-Fluorouracil (5-FU) is a pyrimidine analog that interferes with thymidylate synthesis, and has a broad spectrum of activity against solid tumors. However, 5-FU has limitations that include a short biological half-life due to rapid metabolism, incomplete and non-uniform oral absorption due to metabolism by dihydropyrimidine dehydrogenase (CitationDiasio & Lu, 1994; CitationGamelin et al., 1996; CitationAranda et al., 1998; CitationSchilsky et al., 1998), toxic side-effects on bone marrow and the gastrointestinal tract, and non-selective action against healthy cells (CitationShirasaka et al., 2000).
The present study has been designed to develop and evaluate the potential of hyaluronic acid (HA) conjugated PEGylated nanoparticles for tumor targeting. 5-FU, an anti-cancer drug, has been selected for selective delivery to Ehlich Ascites tumor (EAT). HA-PEG-PLGA triblock copolymer had been synthesized and their core-corona nanoparticles loaded with 5-FU were characterized. The formulation was then extensively studied for its tumor targeting potential. The study reveals that 5-FU-loaded nanoparticles of HA-PEG-PLGA triblock copolymer effectively deliver drug to the tumor cells employing an EPR effect.
Materials and method
Fluorouracil (5-FU) was obtained as a benevolent gift sample from Otto (Mumbai, India). Sodium salt of hyaluronic acid (HA, Mw 5740 Da) was received as a generous gift sample from Cadila Health Care (Ahmedabad, India). PLGA (40–75 KDa), stannous octoate, PEG 2000 (2000 Da), MPEG 2000 (2000 Da), and dialysis membrane (MWCO 2000 Da) were procured from Sigma-Aldrich (Germany). Pluronic F-68, EDAC [1-ethyl-3-dimethylamino-propyl) carbodiimide] and dialysis membrane (MWCO 12,000–14,000 Da) were purchased from Himedia (Mumbai, India). N, N’-dicyclohexylcarbodiimide (DCC), N-hydroxyl succinamide (NHS), methanol, and toluene were purchased from Spectrochem (Mumbai, India). Dimethyl sulfoxide (DMSO) and acetone were procured from Qualigen Fine Chemicals (Mumbai, India). All other chemicals used were of analytical reagent grade and were used as received.
Synthesis of hyaluronic acid-PLGA conjugate (HA-PEG-PLGA) using PEG-bis amine as spacer
PEG-bis-amine was synthesized as per the method reported by CitationZalipsky et al. (1983). One gram of PLGA was dissolved in 25 mL of methylene chloride. Fifty milligrams of DCC and 21 mg of NHS were added to this solution at room temperature under nitrogen (N2) atmosphere (PLGA:NHS:DCC in stoichiometric molar ratio of 2:5:5). The resulting mixture was stirred for 24 h and then filtered. The filtrate was added to ice-cold diethyl ether so as to precipitate the activated PLGA, which was then completely dried under vacuum. Subsequently, the activated PLGA (500 mg) dissolved in 8 mL of DMSO was added slowly to PEG-bis-amine solution (1.5 g of PEG–bis-amine dissolved in 10 mL of DSMO) in a drop-wise manner with gentle stirring. The reaction was carried out for 6 h under N2 atmosphere. The precipitated product, i.e. amine-terminated di-block copolymer, PLGA–PEG–NH2, was dried.
Further, HA-conjugated di-block copolymer was synthesized by coupling PLGA–PEG–NH2 di-block copolymer to an activated HA (0.574 g). HA was activated as mentioned earlier for PLGA. Diblock copolymer (PLGA–PEG–NH2) and activated HA were dissolved in 75 mL DMSO, and added to the reaction flask. The reaction was performed at room temperature for 7 h and then the resultant solution was mixed with 50 mL of distilled water and centrifuged at 3000 rpm (Remi, Mumbai, India). The supernatant was dialyzed using dialysis membrane (MWCO 12,000–14,000 Da). The obtained polymer named HA-PEG-PLGA was then vacuum-dried. The synthesized copolymer was characterized by IR and 1H-NMR spectroscopy. FTIR spectroscopic studies were done using a KBr pellet method after adsorption of a smaller amount of HA-PEG-PLGA on KBr in an IR spectroscope (Perkin-Elmer, USA). NMR spectroscopy was performed at 300 MHz after dissolving the sample in D2O (Bruker DRX, USA).
Preparation of 5-FU loaded HA anchored PLGA nanoparticles (HA-PEG-PLGA-FU)
5-FU loaded nanoparticles were prepared by the nano-precipitation method. HA-PEG-PLGA (10 mg) was dissolved in 10 mL of acetone. Methanolic solution of 5-FU (1 mg/mL) was added to the copolymer solution with stirring. This solution of drug and copolymer was drop-wise added to 20 mL distilled water (with 0.05% w/v Pluronic F-68) under magnetic stirring (Remi, Mumbai, India). Nanoparticles were formed immediately and the solvent was removed via overnight evaporation at room temperature. The resulting suspension was filtered through 0.45 µm membrane filter (Millipore) and then centrifuged for 30 min at 10,000 g and 37 ± 1°C. The supernatant was discarded and nanoparticles were resuspended in distilled water and subsequently freeze-dried. Plain unloaded HA-PEG-PLGA nanoparticles were prepared using a similar method without incorporating the drug.
Characterization of prepared nanoparticles
Morphology
The shape and morphological examination of nanoparticles prepared using HA-PEG-PLGA copolymer was observed by transmission electron microscopy (TEM). TEM of the nanoparticles was carried out at various magnifications (Morgagni 268 D, Fei, The Netherlands) in aqueous medium using 3 mm Forman (0.5% plastic powder in amyl acetate) coated copper grid (300 mesh) at 60 KV and negatively staining with 2% phosphotungastic acid.
Particle size, polydispersity index (PDI), and zeta potential
A suitably diluted suspension of HA-PEG-PLGA nanoparticles and HA-PEG-PLGA-FU nanoparticles was filled in the chamber of a laser diffraction particle size analyzer (DTS Ver. 4.10, Malvern Instruments, UK) and the average particle size and PDI were determined. The zeta potential of the nanoparticles was determined by laser Doppler anemometry using a Malvern Zetasizer (DTS Ver. 4.10; Malvern Instruments). Both HA-PEG-PLGA and HA-PEG-PLGA-FU nanoparticles were dispersed in PBS (pH∼7.4) and the zeta potential was measured.
Differential scanning calorimetry
Transition temperature of 5-FU, HA-PEG-PLGA copolymer, and HA-PEG-PLGA-FU nanoparticles were measured by DSC (TA Instrument 2910 MDSC V4.4E). Each of the samples (5 mg) were separately sealed in aluminium pans and heated at a temperature programmed at 10°C/min under flow of N2. The thermograms were obtained in the range from 0–400°C.
X-ray diffraction (XRD)
X-ray diffractograms of 5-FU, HA-PEG-PLGA, and HA-PEG-PLGA-FU nanoparticles were obtained with a diffractometer (Rigaku D/Max-1200, Rigaku Denki Co. Ltd) using Ni-filtered CuKa radiation (35 kV, 15 mA).
Entrapment efficiency
Ten milligrams of freeze-dried HA-PEG-PLGA-FU nanoparticles were dissolved in acetone. The mixture was centrifuged and supernatant was diluted with methanol-PBS (pH 7.4) mixture. Further, the quantity of entrapped drug was spectrophotometrically (UV-1601 Shimadzu, Japan) analyzed.
In vitro drug release
Ten milligrams of HA-PEG-PLGA-FU nanoparticles were suspended in 2 mL of PBS (pH 7.4) and subsequently put into a dialysis tube (MWCO 2000 Da). The dialysis tube was placed into 50 mL of aqueous recipient medium of PBS. The release media was stirred at 100 rpm at 37 ± 2°C. A whole-media change method was used to avoid drug saturation in the drug release study. At specific time intervals, the whole medium (50 mL) was taken and replaced with the same volume of fresh PBS (50 mL). The samples were then spectrophotometrically analyzed for in vitro drug release.
Hemolytic toxicity
Hemolytic studies were carried out following a reported procedure (CitationSinghai et al., 1997; CitationBhadra et al., 2003). Whole human blood was collected in HiAnticlot blood collection vials (Himedia Labs, India). The red blood cells were separated by centrifugation and resuspended in normal saline solution (10% hematocrit). One milliliter of the RBC suspension was separately incubated with 5 mL of distilled water (taken as 100% hemolytic standard) and 5 mL of normal saline (taken as blank for spectrophotometric estimation). Further, 1 mL of adequately diluted HA-PEG-PLGA polymer (negative control), HA-PEG-PLGA-FU formulation, and plain 5-FU was added to 5.0 mL of n-saline and interacted with RBC suspension The above suspensions were centrifuged for 15 min at 3000 rpm, and the absorbance of supernatant were measured at 540 nm, which was used to estimate percentage hemolysis using distilled water as 100% hemolytic standard.
Hematological study
Healthy male albino rats (Sprague-Dawley strain) of uniform body weight (100 ± 20 g) with no prior drug treatment were used for in vivo studies. The rats were maintained on standard diet and water ad libitum. The Institutional Animal Ethics Committee of Dr. Hari Singh Gour University, Sagar (M.P.) (Registration Number 379/01/ab/CPCSEA, India) had duly approved the protocol for in vivo studies.
The animals were divided into three groups having six rats in each group. Plain 5-FU solution and HA-PEG-PLGA-FU nanoparticles were administered intravenously to the first and second groups of animals, respectively, every day for 7 days in a dose equivalent to 10 mg/kg of 5-FU (CitationHe et al., 2003). The third group was kept as control, which was maintained on the same regular diet for 7 days. For hematological evaluations, red blood cell and platelet counts were made at the 7th day. In addition, plasma concentration of blood urea level (BUL) and creatinine were also determined.
Cytotoxicity study
Cellular cytotoxicity was assessed by tetrazolium dye-based MTT assay following a previously reported procedure (CitationMiglietta et al., 2000; CitationZhang et al., 2004). EAT cells were maintained in RPMI-1640 medium supplemented with 10% heat-inactivated fetal bovine serum and antibiotics at 37°C in a humidified incubator containing 5% CO2. The cells were treated with plain 5-FU and HA-PEG-PLGA-FU nanoparticles in various concentrations (0.01, 0.1, 1.0, 10.0, and 100 µM) for 72 h. The amount of formulation needed to prepare molar equivalents of 5-FU was calculated, based on the drug content in the formulation. Control was taken without any drug treatment. Subsequently, MTT was added and plates were then incubated for another 3 h, the media was pippetted off, and 250 µL DMSO was added. The absorbance of individual wells was noted at 570 nm via an ELISA plate reader at 25.8°C. Average values from triplicate were subtracted from average value of control and the survival fraction of cells was calculated by the formula.
Radiolabeling of nanoparticulate formulation
In the present study, 5-FU and HA-PEG-PLGA-FU nanoparticles were labeled with 99mTc by direct labeling method reported by CitationRichardson et al. (1977). Briefly, 0.1 mL of 99mTc (5 mCi/mL; obtained by solvent extraction method from molybdenum) was mixed with 0.1 ml of stannous chloride solution in 10% acetic acid solution (1 mg/mL), and the pH was adjusted to 7.0 using sodium bicarbonate solution (if required). To this solution, 1 mL of 5-FU solution (1 mg/mL) was added and incubated for 15 min at room temperature. HA-PEG-PLGA-FU nanoparticles were labeled in a similar way. The labeled formulations, thus obtained, were stored in sterile evacuated sealed vials for subsequent studies. Radiolabeling efficiency was determined by ascending instant thin layer chromatography (ITLC) using silica gel-coated fiber sheet (Gelman Science Inc., Ann Arber, MI). ITLC was performed using 100% acetone as a mobile phase. A measured amount of 2–3 µL of the radiolabeled complex was applied at a point 1 cm from one end of an ITLC-SG strip. The strip was developed in acetone and the solvent front was allowed to reach 8 cm from the origin. The strip was cut into two equal halves and the radioactivity in each segment was determined in a well type gamma ray counter (Gamma ray scintillation counter, Type CRS 23C, Electronics Corporation of India Ltd; Mumbai, India). The free 99mTc, which moved with the solvent (Rf = 0.9), was calculated. By subtracting the activity moved with solvent front using acetone from the net amount of 99mTc with 5-FU, 99mTc with 5-FU-loaded nanoparticle (e.g. HA-PEG-PLGA-FU) was calculated.
Biodistribution study of 99mTc labeled 5-FU-loaded nanoparticulate formulations
Permission of the Institutional Animal Ethics Committee, Dr. H. S. Gour University, Sagar (M.P.), India was obtained for all animal experiments. Ehrlich ascites tumor (EAT) cells were grown in the ascites fluid of mice by injecting 1 × 107 cells intra-peritoneally. The cells were harvested after the 7th day of administration. These ascite cells were subcutaneously inoculated in soft tissue of mice to produce solid tumor. The tissue distribution of 5-FU and HA-PEG-PLGA-FU nanoparticles labeled with 99mTc were studied following intravenous administration. The tumor-bearing mice were divided into three groups consisting of six animals in each group. Animals of the first and second groups were injected with plain 5-FU and HA-PEG-PLGA-FU intravenously in the tail vein at a dose equivalent to 10 mg/kg body weight. The third group was kept as control. After 1, 2, and 4 h of administration, the mice were sacrificed by cervical dislocation and their tissues viz. tumor, liver, spleen, lungs, kidney, intestine, heart, stomach, and muscles were excised, washed quickly with cold water to remove surface blood, and the radioactivity was counted. Blood samples (1 mL) were also obtained in duplicate by cardiac puncture in pre-weighed heparinized tubes. The biodistribution of 99mTc-labeled 5-FU and HA-PEG-PLGA-FU in each organ was calculated as a percentage of the injected dose per gram of the tissue (%ID/g). The radioactivity remaining in the tail at the end was also measured and taken into consideration in the calculation of total radioactive dose administered to the mice (CitationRichardson et al., 1977; CitationIba et al., 1997; CitationHe et al., 2003).
Tumor growth inhibition study
Inhibitory effect of HA-PEG-PLGA-FU on the growth of EAT was also studied. EAT cells (1 × 107 cells/mice) were inoculated, intra-peritoneally, into the subcutaneous tissue of the mice. When tumors grew to ∼50–100 mm3 size, mice were divided into three groups. The first and second group of mice were injected with 5-FU and HA-PEG-PLGA-FU nanoparticles, respectively, in a dose equivalent to 10 mg/kg. The third group was kept as control (normal saline). Doses were injected once a day for 12 days. The 5-FU dose administered to mice was 10 mg/ kg (CitationRichardson et al., 1977). Tumor volume was measured daily using a vernier caliper. The tumor volume was calculated using the following equation (CitationPeer & Margalit, 2004);
The results, obtained by in vivo studies, were statistically analyzed by using Student’s t-test with a 95% confidence level (p ≤ 0.05), and were reported as mean standard deviation (SD).
Results and discussion
Synthesis of PLGA-hyaluronic acid conjugate (HA-PEG-PLGA) using PEG- bis-amine as spacer
The present study was designed as an attempt to facilitate spatial delivery of 5-FU from nanoparticles prepared using HA-PEG-PLGA polymer by nanoprecipitation method. HA-PEG-PLGA conjugate was synthesized following the procedure reported by CitationZalipsky et al. (1993) and CitationYadav et al. (2007) using PEG- bis-amine as a spacer. The HA-PEG-PLGA conjugates were prepared via reaction of one of the amino groups of PEG-bisamine with carboxylic group of HA and reacting second free amino group with PLGA (). The percent practical yield of the final synthesized compound HA-PEG-PLGA was found to be 61.4%. Synthesis of HA-PEG-PLGA conjugate was confirmed by FTIR peaks. The FTIR spectrum depicted a typical absorption band of N-H stretch of amide at 3312.3 cm−1 and C=O stretch absorption at 1623.6 cm−1. In addition, aliphatic C–H stretch at 2919.5 cm−1 and O–H stretch of alcohol at 3646.7 cm−1 of HA verified the formation of HA-PEG-PLGA conjugate (supplementary data in the online version of this paper).
In addition, the synthesis of HA-PEG-PLGA copolymer was confirmed by 1H-NMR. In D2O, the occurrence of peaks at δ = 1.2 ppm depict methyl group (–CH3 groups of HA), and δ = 3.4 ppm depict methylene proton peaks (–CH2 groups of PEG). Moreover, an apparent N-acetylate proton peak (NCOCH3) in HA was detected at 2.5 ppm. This study suggested the formation of amide bonds between carboxylic groups of HA and one amine of bis-amine-PEG. In 1H-NMR spectra in CDCl3, the presence of proton peaks showed in 4.8–5.0 ppm depicted the formation of glycolide (supplementary data in the online version of this paper). The results obtained were similar to those obtained previously by our group (CitationYadav et al., 2007).
Preparation of 5-FU-loaded HA anchored PLGA nanoparticles (HA-PEG-PLGA-FU)
HA-PEG-PLGA-FU nanoparticles were prepared by nanoprecipitation method. The addition of drug and copolymer solution to the aqueous phase promotes rapid diffusion of solvent across the solvent–polymer into the aqueous phase and results in an immediate formation of nanoparticles. The nanoparticles were freed from impurities via membrane filtration (0.54 µm) and then resuspended in distilled water.
Characterization of nanoparticles
Morphology
The shape of nanoparticles was visualized under TEM and photomicrograph was obtained. The nanoparticles were found to be spherical and of nanometric-size range ().
Particle size, PDI, and zeta potential determination
The average particle size of HA-PEG-PLGA and HA-PEG-PLGA-FU nanoparticles was found to be 152 ± 3 nm and 165 ± 2 nm with a PDI of 0.054 and 0.065, respectively (). The results are in agreement with TEM studies which also depicted the nanometric size of nanoparticles. Moreover, the zeta potential of HA-PEG-PLGA and HA-PEG-PLGA-FU nanoparticles was 3.41 ± 0.05 mV and 2.95 ± 0.04 mV, respectively. Thus, upon drug loading there was only a small increase in particle size and PDI and zeta potential was only slightly decreased (but still positive). The data clearly indicated that nanoparticles are stable on drug loading and hence may be suitably used as vehicles for ferrying 5-FU.
Table 1. Characterization of HA-PEG-PLGA-FU nanoparticles for percent drug entrapped, particle size, and PDI.
Differential scanning calorimetry
DSC is a very helpful technique for the investigation of thermal properties of nanoparticles, providing both qualitative and quantitative information about physicochemical state of drug inside the nanoparticles. There is no detectable endotherm if the drug is present in a molecular dispersion or solid solution state in the polymeric microspheres/nanoparticles (CitationDubernet, 1995).
In the present investigation, DSC thermograms of pure 5-FU, blank nanoparticles and HA-PEG-PLGA-FU nanoparticles were taken (). The peaks that occurred at temperature above 325.5°C represent the decomposition of HA-PEG-PLGA nanoparticles. HA showed a broad endothermic peak at 80°C. PEG-bisamine illustrated an endothermic peak at 20°C, which is the melting point (Tg) of PEG-bisamine. When the DSC thermogram of HA-PEG-PLGA-FU nanoparticles was compared with HA-PEG-PLGA nanoparticles, a sharp new peak of 5-FU at 262°C and other peaks similar to plain HA-PEG-PLGA were observed. indicates 5-FU is present in a crystalline state. Plain 5-FU showed an exothermic peak at 285°C, as already reported (CitationLee et al., 2003). These findings are in line with those reported by CitationLuo et al. (2000) and CitationLee et al. (2003).
X-ray diffraction
XRD were recorded in order to investigate the physiochemical characteristics of HA-PEG-PLGA-FU (). PEG has characteristic crystalline peaks (supplementary data in the online version of this paper) and PLGA do not show if any peak confirms its amorphous nature. Upon conjugation of HA and PEG, the number of peaks observed in the XRD were increased that illustrated the increased crystalline nature of copolymer (HA-PEG-PLGA). Thus, HA-PEG-PLGA copolymer may perhaps control the degradation rate and accomplish improved physicochemical properties and processibility.
Figure 4. XRD diffractogram of 5-FU (a), HA-PEG-PLGA copolymer (b), and 5-FU-loaded HA-PEG-PLGA nanoparticles (c).
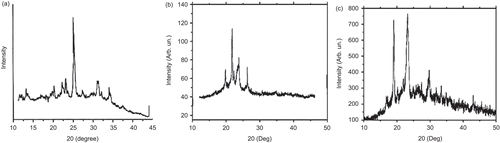
The XRD patterns showed typical sharp peaks of drug crystals, while empty nanoparticles showed relatively simple peaks. In general, free drug exhibits sharp specific peaks when it exists as drug crystals inside the nanoparticles, but the same drug after entrapment show no sharp peaks when it resides as a molecular dispersion. Hence, sharp peaks observed in the case of HA-PEG-PLGA-FU nanoparticles reveal that the crystalline nature of 5-FU is retained even when the drug is encapsulated in the nanoparticles. These findings are in line with those reported by CitationLee et al. (2003).
Entrapment efficiency
The entrapment efficiency HA-PEG-PLGA-FU was determined spectrophotometrically and expressed as the percentage of drug loaded in the nanoparticles vs the total amount of drug added. The efficiency was found to be 80.58 ± 1.42% (). Since 5-FU is hydrophilic it may possibly have partitioned more in the hydrophilic domains than into the hydrophobic domains. Similar results were obtained by CitationCho et al. (2004). The group reported the preparation of long circulating nanoparticles of poly (d,l-lactide-ran-ϵ-caprolactone)-poly(ethylene glycol) poly (d,l-lactide-ran-ϵ-caprolactone) as parenteral drug-delivery systems for 5-FU delivery.
Further, an important factor that may have yielded high encapsulation efficiency is the proper selection of stabilizer that can efficiently reduce the leakage of the drug molecules from the nanoparticles and hence improve drug loading (CitationMu & Feng, 2002).
In vitro 5-FU release
The nanoparticles prepared using amphiphilic block copolymer HA-PEG-PLGA were able to sustain the release of 5-FU only up to 48 h (). This may be attributed to rapid partitioning of hydrophilic drug present in the outer hydrophilic shell of the nanoparticles. The faster release of drug may possibly be due to increased hydration and opening of the hydrophilic framework with increased penetration of water and consequent dissolution of drug as well as hydrophilic block. The results correlate well with similar studies (CitationJeon et al., 2000).
Hemolytic toxicity
The hemolytic toxicity of soluble 5-FU depends on the 5-FU concentration. The 5-FU concentration for nanoparticulate formulation was calculated on the basis of the drug content (data not shown). HA-PEG-PLGA-FU nanoparticles were found to be more hemocompatible. Hence, these can be proposed to serve as suitable candidates for drug delivery application. This may be attributed to the presence of negatively charged functional groups of HA. HA-PEG-PLGA polymer was used as a negative control to observe the hemolytic effect only of the drug when used as plain solution or encapsulated inside the nanoparticles, and cancels out the effect of polymer on RBCs. Moreover, the HA-PEG-PLGA polymer was found to be very minimally hemolytic. The suppression of hemolytic toxicities of drug may be correlated well with other similar studies of PCL-PEG micelles and dendrimers reported earlier (CitationMalik et al., 2000; CitationShuai et al., 2004).
Hematological study
Hematological toxicity study of HA-PEG-PLGA-FU nanoparticles was performed to evaluate the suitability of these nanoparticles as potential drug carriers (). In the case of plain 5-FU and HA-PEG-PLGA-FU nanoparticles, the hemoglobin (Hb) content varied from 20.7 gm/dl to 12.4 gm/dl and 20.0 gm/dl, respectively. Moreover, the platelets count of plain 5-FU and HA-PEG-PLGA-FU nanoparticles diminished from 4.0 × 10−1 millions/cu mm to 1.25 × 10−1 millions/cu mm and 3.34 × 10−1 millions/cu mm, respectively. Similar results were obtained for leucocytes count and RBC count. This may be due to the cytotoxic and hemolytic nature of free drug. Encapsulation of drug in the nanoparticles followed by pegylation prevents the possible interaction of the drug with various blood cells and thereby reduces its toxicity.
Table 2. Hematological parameters in albino rats after administration of HA-PEG-PLGA-FU.
In addition, blood urea level (BUL) of plain 5-FU and HA-PEG-PLGA-FU nanoparticles increased from 23.6 mg/dl to 42.0 mg/dl and 24.5 mg/dl, respectively. Moreover, the serum creatinine content altered from 0.34 mg/dl to 1.2 mg/dl and 0.40 mg/dl in the case of 5-FU and HA-PEG-PLGA-FU nanoparticles, respectively (). The studies on creatinine and BUN content indicate that the liver and renal functions were normal and not affected by injection of nanoparticulate formulations. This implies that nano-particulate formulation did not cause unexpected side-effects and may be judged as a safe carrier for drug delivery. The results are in accordance to the results obtained previously by CitationBhadra et al. (2003).
Cytotoxicity study
The growth inhibition of HA-PEG-PLGA-FU on EAT cells was investigated by MTT assay. The results clearly suggested a dose-dependent cytotoxicity that is reduced cellular viability upon increasing the concentration of 5-FU (both plain 5-FU and HA-PEG-PLGA-FU nanoparticles). The survival fraction of cells upon incubation of plain 5-FU and HA-PEG-PLGA-FU nanoparticles in varying concentration is shown in .
After 72 h of incubation, plain 5-FU as well as HA-PEG-PLGA-FU nanoparticles did not illustrate any inhibitory effect on cell growth, when the concentration of 5-FU was below 0.01 µM/mL. Further the cell viability diminished when the concentration of 5-FU either in free form or inside the nanoparticles was increased. Almost all the cells were killed when the concentration of 5-FU was 100 µM/mL, indicating internalization of adequate 5-FU inside nuclei during this incubation period. In the concentration range of 0.01–100 µM/mL, HA-PEG-PLGA-FU nanoparticles were cytotoxic to a greater degree in comparison to plain 5-FU. This is concordant with HA receptor-binding capacity exhibited by HA-PEG-PLGA-FU nanoparticles. The study suggests that ligand conjugated nanoparticles exert greater cytotoxicity to the tumor cells. This may have an important in vivo bearing where conjugating the nanoparticles with ligand would ferry them to the tumor cells and sparing non-tumor cells, thus with the interception of minimal side-effects. The results are in line with those observed by CitationMiglietta et al. (2000) for solid lipid nanoparticles using doxorubicin and paclitaxel as model drugs
Radiolabeling of nanoparticulate formulation
99mTc has been used to directly label preformed nanoparticles based on stannous chloride as a reducing agent. 99mTc is easily available, cost effective, and a low radiation dose (CitationLove et al., 1989). Hence, it is widely used for radiolabeling study. Since the half-life of 99mTc is 6 h as compared to 60 days of 125I, it presents less radiation hazard. Reduced 99mTc is added to the nanoparticles, which can easily bind to the nanoparticles surface. This surface labeling approach is technically simple and good for the production of nanoparticles in a pharmaceutically acceptable form, and was therefore used for the present study. In the present study the radiolabeling efficiency on nanoparticles was always more than 90%. Since the labeling yield as determined by ITLC was high enough, further separation before the animal experiment was not needed.
Biodistribution study of 99mTc labeled 5-FU-loaded nanoparticulate formulation
The biodistribution of 5-FU with 99mTc either as free drug or encapsulated in HA-PEG-PLGA nanoparticles were performed in EAT-bearing mouse models. The percentage of injected dose/g distribution to tissue in different organs at different time intervals for plain 99Tc-5-FU and HA-PEG-PLGA-FU nanoparticles labeled with 99Tc are shown in . The studies were performed to evaluate the delivery at various tissues. As expected, HA-PEG-PLGA-FU had enhanced access to the tumor mass and reduced entry in the non-target organs as compared to plain 5-FU. Plain drug was found to have greatest access to blood and secondarily to liver ().
Figure 7. Biodistribution pattern of 99mTc labeled 5-FU (a) and 99mTc labeled 5-FU-loaded HA-PEG-PLGA (b) nanoparticles in EAT tumor-bearing mice.
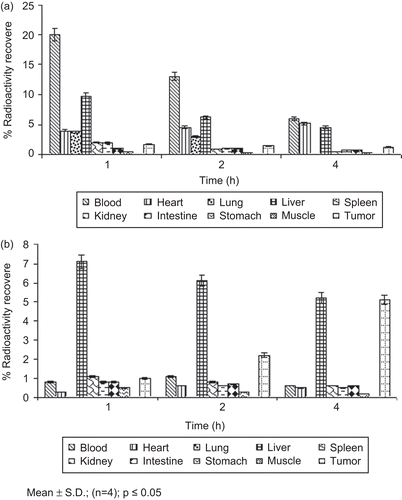
In contrast the drug in the formulation was found to have greatest entry in the tumors and liver (). The percent radioactivity recovered in the case of free drug and HA-PEG-PLGA-FU was 1.2 ± 0.05% and 5.1 ± 0.09%, respectively, after 4 h. This may be explained on the basis of tumor-specific targeting of HA-conjugated formulation. Activated HA receptors, that is CD44 and RHAMM, are over-expressed on tumor cells and are absent on non-tumor cells (CitationAsplund & Heldin, 1994, CitationWang et al., 1998; CitationNaor et al., 2002). Receptor mediated endocytosis by CD44 may possibly be responsible for greater uptake of HA anchored nanoparticles (CitationCulty et al., 1992; CitationYadav et al., 2007). Further, enhanced permeation and retention (EPR) effects prevented the entry of the formulation in the normal cells at the same time favored selective entry in tumors (CitationZeng et al., 1998; CitationLesley et al., 2000; CitationShenoy & Amiji 2005). This elucidated that while a very small amount was found in tumor tissues after 4 h when plain 5-FU was administered, an ∼4-fold higher concentration of 5-FU was delivered via HA-PEG-PLGA-FU. This specifies the enhanced bioavailability and localized action of the formulation at tumor sites, subsequently augmenting the therapeutic potential by endowing with the prospective to lower the dose of drug requisite for its delivery. The above outcomes may be accredited to the altered vasculature of the tumor mass (CitationAgarwal et al., 2009). Thus, the present formulation utilizes targeting potential and EPR effect for greater accessibility and prolonged retention to these highly leaky and porous architectures.
Tumor growth inhibition study
In vivo tumor growth inhibition study in mice was tested in an EAT tumor model with established tumors using a single dose of the formulation. Initially, the tumor size of treated groups was not statistically significantly (p ≥ 0.05) different from the control group (that is 97.9 ± 1.2 mm3) (). The size of tumor mass in the case of control (injected with normal saline) was increased to 104.6 ± 1.1 mm3. While plain 5-FU dose was efficient in restraining further tumor growth, diminution of tumor size was not seen, and the activity was short lived. The volume of the tumor mass was found to be reduced to 93.7 ± 1.1 mm3 after 15 days, and thus the tumor reduction rate was only 4.3 ± 0.06%. More importantly, treatment of the animals with plain 5-FU at the dose level of 10 mg/kg induced significant signs of toxicity; that is, a pronounced weight loss. HA-PEG-PLGA-FU (targeted) nanoparticles were found to be more active than 5-FU in solution, and lead to a significant reduction in tumor size. The tumor volume was found to be reduced to 78.52 ± 0.9 mm3 and thus the tumor inhibition rate was 19.8 ± 0.1%. It is clear from the observation of that 5-FU-loaded HA anchored PLGA nanoparticulates suppressed the tumor growth more significantly (4.6-times) than the animals treated;p ≤ 0.001 was the level of significance achieved, which clearly indicates significant tumor regression by HA-PEG-PLGA-FU nanoparticles as compared to plain 5-FU.
Figure 8. In vivo tumor growth inhibition study of 5-FU-loaded HA-PEG-PLGA nanoparticles in EAT tumor-bearing mice.
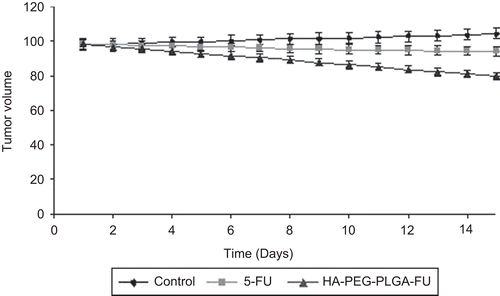
Significant reduction in tumor volume was probably due to the acid mucopolysaccharide present in the ascitic fluid supernatant, i.e. hyaluronic acid (HA). Hyaluronic acid has been reported to be of extracellular origin, and is bound to proteins of the cell membrane. The ascites cells show evidence of vigorous production of sulfated mucopolysaccharides, particularly in the mitochondria and at cell membrane level (CitationPark et al., 2005). HA-PEG-PLGA nanoparticles might have led to an augmented HA level, and this may attribute to exogenous oligomeric HA mediated inhibition of tumor progression, most probably by competing with endogenous polymeric HA (CitationAnghileri, 1976; CitationZeng et al., 1998; CitationLesley et al., 2000; CitationYadav et al., 2007).
In the animal group receiving HA-PEG-PLGA-FU, all of the animals appeared lively throughout the study, and no considerable weight loss was detected. There were no signs of decreased activity, which would have indicated general toxicity of the formulation. As a result, the formulation may be considered to be safe at the dosing schedule used. Similar findings have been reported by CitationMo and Lim (2005), who used wheat germ agglutinin anchored PLGA nanoparticles for SCID mice model engrafted with A549 tumor nodule and found no substantial loss in body weight of the animals.
Conclusion
In order to deliver 5-FU in the tumor cell, HA-PEG-PLGA copolymers were synthesized and used to prepare 5-FU-loaded nanoparticles. The nanoparticulate system was found to be safe for intravenous administration, more hemo-compatible, and biocompatible. On the basis of the above studies, it can be concluded that HA-PEG-PLGA nanoparticles can serve as efficient tools to ferry large doses of anti-cancer drug to tumor sites, with reduced access to non-tumor tissues. Thus, optimal therapeutic response may be achieved to provide effective tumor regression with the interception of minimal side-effects.
Declaration of interest
One of the authors, Mr Awesh K. Yadav, expresses his sincere thanks to ICMR, New Delhi, India, for financial support. The authors also thank SAIF-CDRI (Lucknow, India) for providing the IR and NMR facility and Dr S. C. Sharma of All India Institute of Medical Sciences (New Delhi, India) for providing TEM facilities. The authors acknowledge Dr Choudhary, Dr Awasthi, and Mr Bhardhwaj of IUC-DAE (Indore, India) and SAIF (Nagpur, India) for providing the DSC and XRD facility.
Supplementary Material
Download PDF (127 KB)References
- Agarwal, A., Asthana, A., Gupta, U., Jain, N.K. (2008b). Tumour and dendrimers: a review on drug delivery aspects. JPP. 60:671–88.
- Agarwal, A., Gupta, U., Asthana, A., Jain, N.K. (2009). Dextran conjugated dendritic nanoconstructs as potential vectors for anti-cancer agent. Biomaterials. 30:3588–96.
- Agarwal, A., Saraf, S., Asthana, A., Gupta, U., Gajbhiye, V., Jain, N.K. (2008a). Ligand based dendritic sytems for tumor targeting. Int J Pharm. 350:3–13.
- Anghileri, L.J. (1976). In vivo synthesis of acid mucopolysaccharides by Ehrlich ascites tumor cells. J Cancer Res Clin Oncol. 88:17–24.
- Aranda, E., Diaz-Rubio, E., Cervantes, A., Anton-Torres, A., Carrato, A., Massuti, T., Tabernero, J.M., Sastre, J., Tres, A., Aparicio, J., Lopez-Vega, J.M., Barneto, I., Garcia-Conde, J. (1998). Randomized trial comparing monthly low-dose leucovorin and fluorouracil bolus with weekly high-dose 48-hour continuous-infusion fluorouracil for advanced colorectal cancer: a Spanish Cooperative Group for Gastrointestinal Tumor Therapy (TTD) study. Ann Oncol. 9:727–31.
- Asplund, T., Heldin, P. (1994). Hyaluronan receptors are expressed on human malignant mesothelioma cells but not on normal mesothelial cells. Cancer Res. 54:4516–23.
- Bhadra, D., Bhadra, S., Jain, S., Jain, N.K. (2003). PEGylated dendritic nanoparticulate carrier of fluorouracil. Int J Pharm. 257:111–24.
- Cho, H., Chung, D., Jeongho, A. (2004). Poly(D,L-lactide-ran-ϵ-caprolactone)-poly (ethylene glycol)-poly(D,L-lactide-ran-ϵ-caprolactone) as parenteral drug-delivery systems. Biomaterials. 25:3733–42.
- Culty, M., Nguyen, H.A., Underhillm, C.B. (1992). The hyaluronan receptor (CD44) participates in the uptake and degradation of hyaluronan. J Cell Biol. 116:1055–62.
- Diasio, R.B., Lu, Z. (1994). Dihydropyrimidine dehydrogenase activity and fluorouracil chemotherapy. J Clin Oncol. 12:2239–42.
- Dubernet, C. (1995). Thermoanalysis of microspheres. Thermochim Acta. 248:259–69.
- Gamelin, E.C., Danquechin-Dorval, E.M., Dumesnil, Y.F., Maillart, P.J., Goudier, M.J., Burtin, P.C., Delva, R.G., Lortholary, A.H., Gesta, P.H., Larra, F.G. (1996). Relationship between 5-fluorouracil (5-FU) dose intensity and therapeutic response in patients with advanced colorectal cancer receiving infusional therapy containing 5-FU. Cancer 77:441–51.
- Gref, R., Couvreur, P., Barratt, G., Mysiakine, E. (2003). Surface-engineered nanoparticles for multiple ligand coupling. Biomaterials. 24:4529–37.
- Hans, M., Shimoni, K., Danino, D., Siegel, S.J., Lowman, A. (2005). Synthesis and characterization of mPEG-PLA prodrug micelles. Biomacromolecules. 6:2708–17.
- He, Y.C., Chen, J.W., Cao, J., Pan, D.Y., Qiao, J.G. (2003). Toxicities and therapeutic effect of 5-fluorouracil controlled release implant on tumor-bearing rats. World J Gastroenterol. 9:1795–98.
- Iba, T., Yagi, Y., Kidokoro, A., Fukunaga, M., Momose, F., Nagakari, K. (1997). Intermittent low-dose cisplatin infusion as a possible modulator of 5-fluorouracil. Int J Clin Oncol. 2:197–201.
- Jeon, H., Jeong, Y., Jang, M., Park, Y., Nah, J. (2000). Effect of solvent on the preparation of surfactant-free poly (D,L-lactide-co-glycolide) nanoparticles and norfloxacin release characteristics. Int J Pharm. 207:99–108.
- Lee, J.S., Chae, G.S., An, T.K., Khang, G. (2003). Preparation of 5-fluorouracil-loaded poly(L-lactide-co-glycolide) wafer and evaluation of in vitro release behavior. Macromol Res. 11:183–88.
- Lesley, J., Hascall, V.C., Tammi, M., Hyman, R. (2000). Hyaluronan binding by cell surface CD44. J Biol Chem. 275:26967–75.
- Li, Y.P., Ogris, M., Wagner, E., Pelisek, J., Ruffer, M. (2003). Nanoparticles bearing polyethylene glycol-coupled transferrin as gene carriers: preparation and in vitro evaluation. Int J Pharm. 259:93–101.
- Love, W.G., Amos, N., Williams, B.D., Kellaway, I.W. (1989). Effect of liposome surface charge on the stability of technetium (99mTc) radiolabelled liposomes. J Microencap. 6:105–13.
- Luo, Y., Kirker, K.R., Prestwich, G.D. (2000). Cross-linked hyaluronic acid hydrogel films: new biomaterials for drug delivery. J Control Rel. 69:169–84.
- Malik, N., Wiwattanapatapee, R., Klopsch, R., Lorenz, K., Frey, H., Weener, J.W., Meijer, E.W., Paulus, W., Duncan, R. (2000). Dendrimers: relationship between structure and biocompatibility in vitro, and preliminary studies on the biodistribution of 125I-labelled polyamidoamine dendrimers in vivo. J Contr Rel. 65:133–48.
- Miglietta, A., Cavalli, R., Bocca, C., Gabriel, L., Gasco, M.R. (2000). Cellular uptake and cytotoxicity of solid lipid nanospheres (SLN) incorporating doxorubicin or paclitaxel. Int J Pharm. 210:61–7
- Mo, Y., Lim, L.Y. (2005). Paclitaxel-loaded PLGA nanoparticles: potentiation of anticancer activity by surface conjugation with wheat germ agglutinin. J Control Rel 108:244–62.
- Mohanraj, V.J., Chen, Y. (2006). Nanoparticles–a review. TJPR. 5:561–73.
- Mu, L., Feng, S.S. (2002). Vitamin E TPGS used as emulsifier in the solvent evaporation/extraction technique for fabrication of polymeric nanospheres for controlled release of paclitaxel. J Contr Rel. 80:129–44.
- Naor, D., Nedvetzki, S., Golan, I., Melnik, L., Faitelson, Y. (2002). CD44 in cancer. Crit Rev Clin Lab Sci, 39:527–79.
- Nasongkla, N., Shuai, X., Ai, H., Weinberg, B.D., Pink, J., Boothman, D.A., Gao, J. (2004). cRGD-functionalized polymer micelles for targeted doxorubicin delivery. Angew Chem Int Ed. 43:6323–27.
- Panyam, J., Labhasetwar, V. (2003). Biodegradable nanoparticles for drug and gene delivery to cells and tissue. Adv Drug Deliv Rev. 55:329–47.
- Park, E.K., Lee, S.B., Lee, Y.M. (2005). Preparation and characterization of methoxy poly- (ethylene glycol)/poly (ϵ-caprolactone) amphiphilic block copolymeric nanospheres for tumor-specific folate-mediated targeting of anticancer drugs. Biomaterials. 26:1053–61.
- Peer, D., Margalit, R. (2004). Tumor-targeted hyaluronan nanoliposomes increase the antitumor activity of liposomal doxorubicin in syngeneic and human xenograft mouse tumor models. Neoplasia. 6:343–53.
- Peracchia, M.T., Gref, R., Minamitake, Y., Domb, A., Lotan, N., Langer, R. (1997). PEG-coated nanospheres from amphiphilic diblock and multiblock copolymers: investigation of their drug encapsulation and release characteristics. J Control Release. 46:223–31.
- Perez, C., Sanchez, A., Putnam, D., Ting, D., Langer, R., Alonso, M.J. (2001). Poly(lactic acid)–poly(ethylene glycol) nanoparticles as new carriers for the delivery of plasmid DNA. J Contr Rel. 75:211–24.
- Ratnam, M., Hao, H., Zheng, X., Wang, H., Qi, H., Lee, R., Pan, X. (2003). Receptor induction and targeted drug delivery: a new antileukemia strategy. Expert Opin Biol Ther. 3:563–74.
- Reddy, J.A., Dean, D., Kennedy, M.D., Low, P.S. (1999). Optimization of folate conjugated liposomal vectors for folate receptor-mediated gene therapy. J Pharm Sci. 88:1112–8.
- Richardson, V.J., Jeyasingh, K., Jewkes, R.F. (1977). Properties of [99mTc] technetium-labeled liposomes in normal and tumour-bearing rats. Biochem Soc Trans. 5:220–9.
- Schilsky, R.L., Hohneker, J., Ratain, M.J., Janisch, L., Smetzer, L., Lucas, V.S., Khor, S.P., Diasio, R., Von Hoff, D.D., Burris, H.A. (1998). 3rd Phase I clinical and pharmacologic study of eniluracil plus fluorouracil in patients with advanced cancer. J Clin Oncol. 16:1450–7.
- Shenoy, D., Little, S., Langer, R., Amiji, M. (2005). Poly (ethylene oxide)-modified poly (β-amino ester) nanoparticles as a pH-sensitive system for tumor-targeted delivery of hydrophobic drugs: part 2. In vivo distribution and tumor localization studies. Pharm Res. 22:2101–14.
- Shenoy, D.B., Amiji, M.M. (2005). Poly (ethylene oxide)-modified poly (epsilon caprolactone) nanoparticles for targeted delivery of tamoxifen in breast cancer. Int J Pharm. 293:261–70.
- Shirasaka, T., Yamamitsu, S., Tsuji, A., Terashima, M., Hirata, K. (2000). Conceptual changes in cancer chemotherapy–biochemical modulation of 5-FU. Gan To Kagaku Ryoho. 27:832–45.
- Shuai, X., Ai, H., Nasongkla, N., Kim, S., Gao, J. (2004). Micellar carriers based on block copolymers of poly (ϵ-caprolactone) and poly (ethylene glycol) for doxorubicin delivery. J Control Rel. 98:415–26.
- Singhai, A.K., Jain, S., Jain, N.K. (1997). Evaluation of aqueous suspension of ketoprofen. Pharmazie. 52:149–51.
- Swayam, P., Labhasetwar, V. (2004). Critical determinants in PLGA/PLA nanoparticles-mediated gene expression. Pharm Res. 21:354–64.
- Wang, C., Thor, A.D., Moore, D.H., Zhao, Y., Kerschmann, R., Stern, R., Watson, P.H., Turley, E.A. (1998). The overexpression of RHAMM, a hyaluronan-binding protein that regulates ras signalling, correlates with over-expression of mitogenactivated protein kinase and is a significant parameter in breast cancer progression. Clin Cancer Res. 4:567–76.
- Xu, Z., Gu, W., Huang, J., Sui, H., Zhou, Z., Yang, Y., Yan, Z., Li, Y. (2005). In vitro and in vivo evaluation of actively targetable nanoparticles for paclitaxel delivery. Int J Pharm. 288:361–8.
- Yadav, A.K., Mishra, P., Mishra, A.K., Mishra, P., Jain, S., Agrawal, G.P. (2007). Development and characterization of hyaluronic acid-anchored PLGA nanoparticulate carriers of doxorubicin. Nanomed Nanotechnol Biol Med. 3:246–57.
- Yuan Li, W.G., Li, Z.S., Zhang, S.H., Sun, Y.J., Hu, P.Z., Wang, X.M., Huang, Y., Si, S.Y., Zhang, X.M., Sui, Y.F. (2009). The antitumor immune responses induced by nanoemulsion-encapsulated MAGE1-HSP70/SEA complex protein vaccine following peroral administration route. Cancer Immunol Immunother. 58:201–8.
- Yun, Y.H., Goetz, D.J., Yellen, P., Chen, W. (2004). Hyaluronan microspheres for sustained gene delivery and site-specific targeting. Biomaterials. 25:147–57.
- Zalipsky, S., Gilon, C., Zilkha, A. (1983). Attachment of drugs to polyethylene glycols. Eur Polym J. 19:1177–83.
- Zambaux, M.F., Bonneaux, F., Gref, R., Dellacherie, E., Vigneron, C. (2001). Protein C-loaded monomethoxypoly(ethylene oxide)–poly(lactic acid) nanoparticles. Int J Pharm. 212:1–9.
- Zeng, C., Toole, B.P., Kinney, S.D., Kuo, J.W., Stamenkovic, I. (1998). Inhibition of tumor growth in vivo by hyaluronan oligomers. Int J Cancer. 77:396–401.
- Zhang, Y., Guo, L., Roeske, R.W., Antony, A.C., Jayaram, H.N. (2004). Pteroyl-γ-glutamate-cysteine synthesis and its application in folate receptor-mediated cancer cell targeting using folate-tethered liposomes. Anal Biochem. 332:168–77.