Abstract
Gelatin nanoparticles (GNPs) and aminated gelatin nanoparticles (AGNPs) were prepared and used as an adjuvant to improve the delivery of tetanus toxoid (TT). Nanoparticles were characterized in vitro for their size, shape, entrapment, and release. TT-FITC conjugate was used to determine entrapment and release from nanoparticles. The immune-stimulating activity was studied by measuring anti-TT IgG, IgG1, and IgG2a isotype and cytokine responses following subcutaneous (s.c) injection of nanoparticles in BALB/c mice and was compared with alum-TT vaccine. Gelatin and aminated gelatin (AG) specific IgG response was also determined. Both GNPs and AGNPs demonstrated comparable IgG response and a significantly higher (p < 0.05) cytokine response (IL-2 and IFN γ) as compared to alum-TT vaccine. Nanoparticulate formulations elicited both Th1 and Th2 responses and induced negligible undesirable immunogenicity against the carrier, as demonstrated by lower level of gelatin and AG-specific IgG response as compared to control.
Introduction
The development of new adjuvants for human vaccines has become an expanding field of research in the last 30 years, for generating stronger vaccines capable of inducing protective and long-lasting immunity in humans. In spite of such efforts with several adjuvant strategies approaching to requirements for their clinical application, limitations like adjuvant toxicity remain to be fully surpassed (CitationAguilar & Rodriguez, 2007). The most widely used adjuvants in humans are aluminum salt precipitates, often referred to as ‘alum’ (CitationAllison & Byars, 1991). Unfortunately, alum salts are relatively poor adjuvants in many situations, particularly at inducing cellular immune responses (CitationSchirmbeck et al., 1994; CitationTraquina et al., 1996). Occasionally, these vaccines have been associated with severe local reactions such as erythema (CitationAprile & Wardlaw, 1966; CitationCollier et al., 1979), subcutaneous nodules (CitationFrost et al., 1985), contact hypersensitivity (CitationClemmenson & Knudsen, 1980), and granulomatous inflammation (CitationWhite et al., 1955; CitationErodohazi & Newman, 1971). In animals, as well as in humans, aluminum adjuvants increase the level of antigen-specific and total IgE antibodies (CitationNagel et al., 1977; CitationVassilev, 1978; CitationMatsuhasi & Ikegami, 1982) and may promote IgE-mediated allergic reactions. Aluminum is not ‘biodegradable’ (CitationNixon et al., 1992). Aluminum adjuvants have been found at the site of subcutaneous injection in mice and guinea pigs for up to 1 year (CitationGupta et al., 1996). Certainly, regulatory requirements have been raised since alum development and, arguably, its toxicity and side-effects may preclude its registration for human use by regulatory bodies if it were only newly discovered as an adjuvant today.
In the development process of a nanoparticulate delivery system for in vivo application, biodegradability without toxic by-products is one of the major claims a potential matrix molecule has to fulfil. Gelatin-based delivery systems are biocompatible and biodegradable without toxic degradation products (CitationTabata & Ikada, 1998). The beneficial properties of gelatin contribute to its proven record of safety which is documented by the classification as ‘Generally Recognized as Safe’ (GRAS) excipient by the US Food and Drug Administration (FDA). Gelatin derivatives are even constituent of intravenously administered applications as plasma expanders (CitationKuijpers et al., 2000). Moreover, it has been reported recently that there is a significant uptake of gelatin nanoparticles by murine bone marrow dendritic cells (DCs) and they are suitable for targeting antigens to DCs (CitationCoester et al., 2006). These encouraging results, combined with the biocompatibility, non-toxic properties and cost effectiveness of gelatine, make it a suitable candidate to be evaluated as an immunoadjuvant.
In the present work tetanus toxoid was used to study the adjuvant efficacy of the prepared nanoparticles. Tetanus toxoid represents an ideal protein that would substantially benefit from the microencapsulation technologies due to its soluble nature (CitationJaganathan et al., 2005). Moreover, because of its easy availability and characteristics, TT appears as a suitable antigen to attempt alternative approaches to the existing vaccines.
Therefore, the goal of the present study was first to develop reproducible and homogeneous preparation of TT loaded nanoparticles, which is a first important step for further scale-up and use for any particulate carrier. The second goal was to study the immune stimulating property of the formulations in an animal model.
Materials and methods
Gelatin Type A derived from porcine skin (Bloom 175) and fluorescein isothiocyanate (FITC) were purchased from Sigma Chemical Co. (St Louis, MO). TT (MW ~ 150 kDa), 3000 Lf/ml (Limes flocculation, the international unit for vaccines), protein content 8 mg/ml, was obtained from Serum institute of India Ltd. (Pune, India). Glutaraldehyde was purchased from Spectrochem pvt ltd. Mumbai, India. Aluminium hydroxide gel, i.e. Alhydrogel 2.0% (w/v) (Alum) was from Brenntag Biosector, Frederikssund, Denmark. All other chemicals and reagents used were of analytical grade and purchased from local suppliers.
Preparation of FITC-TT
Before the preparation of antigen-loaded gelatin nanoparticles, FITC-labeled TT was prepared (CitationCopland et al., 2003) for the determination of percentage entrapment efficiency and antigen release. A total of 10 mg of fluorescein isothiocyanate (FITC) was dissolved in 5 ml of carbonate buffer pH 9.5, and 5 ml of TT solution was added. The mixture was gently stirred in the dark at 4°C for 20 h. The solution was then extensively dialysed against Milli-Q™ water using a 10,000 MW cut-off membrane. The resulting FITC-tetanus toxoid (FITC–TT) solution was freeze-dried and subsequently protected from light and stored at 4°C. Prior to use, the protein was analyzed for the presence of free FITC by ultra-centrifugation (10,000 × g for 20 min) of an aqueous solution through a 10,000 MW cut-off filter unit (Millipore India Pvt. Ltd., Bangalore, India). Unbound FITC was found to be less than 1.4% of total fluorescence in all cases.
Preparation of gelatin nanoparticles
Gelatin nanoparticles were prepared by a two-step desolvation method, as described by CitationCoester et al. (2000). In brief: 250 mg gelatin was dissolved in 5 ml MilliQ™ water (conductivity max. 0.05 µS/0.05 µMho) under constant heating (40°C); 5 ml acetone was added to the gelatin solution as a desolvating agent to precipitate the high molecular weight (HMW) gelatin. The supernatant was discarded and the HMW gelatin was re-dissolved by adding 5 ml distilled water and stirred at 600 rpm under constant heating. pH of the solution was adjusted to 3; 1 mg of FITC-TT or TT was added to the gelatin solution before the second desolvation step. In situ gelatin nanoparticles were formed during a second desolvation step by drop-wise addition of 15 ml acetone under stirring (600 rpm). After 10 min, 50 µl of glutaraldehyde (25% w/v) was added to the reaction vessel to cross-link the nanoparticles and it was further stirred for 3 h at 600 rpm. The remaining organic solvent was evaporated using a rotary evaporator (Strike steroglass, Campo Perugia Italy) and, finally, the particles were purified by 3-fold centrifugation (20,000 g for 20 min, Hitachi Preparative Ultracentrifuges, Tokyo, Japan) and nanoparticles were stored as dispersion in highly purified water at 2–8°C. Aseptic conditions were maintained during the manufacturing steps. The sterile working area was regularly monitored by sampling the air and surface of the working area. The sterility tests were performed using fluid thioglycollate medium and soyabean-casein digest medium.
Synthesis of aminated gelatin and preparation of aminated gelatin nanoparticles
Gelatin was made to react with 1, 2-ethylenediamine to obtain aminated gelatins in the presence of 1-ethyl-3-(3-dimethylaminopropyl)-carbodiimide hydrochloride (CitationSeki et al., 2005). In brief, gelatin (10 g) was dissolved in 0.1 M phosphate buffer (pH 5.0, 250 ml) at 45°C. 1, 2-ethylenediamine (28 g) was added to the solution and the pH of the solution was adjusted to 5.0 with 0.1 M HCl. The resulting solution was mixed with 1-ethyl-3-(3-dimethylaminopropyl)-carbodiimide hydrochloride (5.35 g) and the total volume were adjusted to 500 ml using phosphate buffer (pH 5.0). The reaction was allowed to take place at 37°C for 1 h. The generated aminated gelatin was purified by dialysis (10,000 MW cut-off membrane, Himedia laboratories pvt. Ltd, Mumbai, India) for 48 h in MilliQ water. Eventually, the aminated gelatin powder was obtained by lyophilization. The further particle preparation was performed according to the procedure for gelatin nanoparticles.
Quantification of free amino groups after cross-linking
Free amino groups were determined using the reaction of 2, 4, 6-trinitrobenzenesulfonic acid (TNBS) with amino groups of the particles as described by CitationFields (1971). The nanoparticles were washed and centrifuged four times (Hitachi Preparative Ultracentrifuges, Tokyo, Japan) at 100,000 g for 5 min, followed by re-dispersion in water. Two-hundred microliters of the nanoparticle dispersion was diluted with water to a final volume of 400 µl; 400 µl of 4% sodium hydrogen carbonate solution (pH 8.5) and 400 µl aqueous 0.1% TNBS solution was added. The mixture was stirred at 500 rpm for 2 h at 40°C in the dark. The nanoparticles were separated from the supernatant by centrifugation at 100,000 g for 15 min and 125 µl of the supernatant was diluted with 500 µl of water. The samples were assayed at 349 nm for un-reacted TNBS using an UV/VIS spectrophotometer (Shimadzu, Kyoto, Japan). The free amino group content of the nanoparticles was calculated relative to a TNBS control, which was treated in the same manner as described above: using water instead of the nanoparticle dispersion.
Determination of percentage entrapment efficiency
FITC-TT loaded nanoparticles were prepared and purified as described above. For determining entrapment, nanoparticles were dispersed in 2 ml (20 mg/ml) and were centrifuged at 20,000 g for 30 min and the sedimented particles were mixed with 2.0 ml of 0.2 M NaOH solution until a clear solution was observed (~ 48 h) at 4°C. The solution was again centrifuged at 20,000 g for 30 min. Amount of the protein was determined by measuring fluorescence (excitation wavelength: 488 nm, emission wavelength: 520 nm) in the supernatant using a fluorescence spectrophotometer (RF-5301PC spectrofluorophotometer, Shimadzu). All fluorescence readings were analyzed using a standard curve of FITC-TT in PBS (pH 7.4). To correct for interferences, background fluorescent intensity reading was subtracted (i.e. fluorescent intensity readings for the unloaded nanoparticles treated in the same way as FITC-TT loaded nanoparticles).
In vitro release and degradation profile of nanoparticles
The 48 h release of FITC-TT loaded nanoparticles was carried out in PBS (pH 7.4, 0.05 M) at 37°C in a shaking water bath in dark. Degradation profile of gelatin nanoparticles was studied by enzymatic digestion of the nanoparticles using collagenase; 20 mg of FITC-TT loaded gelatin nanoparticles were resuspended in 0.8 mg/ml of collagenase in 2 ml PBS (pH 7.4, 0.05 M) and vortexed. At different time intervals, the suspension was centrifuged (40,000 g for 20 min) to get supernatants and the amount of FITC-TT released was determined by measuring the fluorescent intensity of the supernatant. Enzyme solution was replaced each time in the respective vial containing nanoparticles to maintain a constant volume. The fluorescent intensity of the supernatant obtained at different time intervals was measured under the same conditions as described above for the determination of FITC-TT entrapment and the cumulative amount released was calculated from the appropriate calibration curves. For both the studies, background fluorescent intensity reading was subtracted (i.e. fluorescent intensity readings for the unloaded nanoparticles treated in the same way as FITC-TT loaded nanoparticles).
Characterization of nanoparticles
Particle size determination was performed by dynamic light scattering (DLS). Scanning electron microscopy (SEM) was applied to image the surface of nanoparticles and its distribution.
Dynamic light scattering (DLS)
Samples were analyzed with a Zetasizer Nano ZS 90 (Malvern Instruments Ltd, Worcestershire, UK) using NIBS™ technology. NIBS (non-invasive back-scatter) technology extends the range of sizes and concentrations of samples that can be measured. The scattering information was gathered at an angle of 173°. The nanoparticles were diluted in MilliQ™ water (conductivity max 0.05 µS/0.05 µMho) and measured at concentrations between 40–100 µg/ml. Due to these low concentrations, the nanoparticles did not influence the viscosity of the dispersion; therefore, the viscosity was set to be that of pure water (i.e. 0.8872 cP at 25°C). Polydispersity index (PDI), an indicator of particle size homogeneity, was also obtained from DLS experiments.
SEM analysis of nanoparticles
Size and surface morphology of nanoparticles were studied by scanning electron microscopy (SEM). The pictures were taken using a field emission scanning electron microscope (Philips CM12, Eindhoven, Netherlands) at 5.0 kV and a working distance of 9.7 mm. For sample preparation gelatin nanoparticles were dispersed in acetone at a concentration of 40 µg/ml and applied on a specifically polished sample grid. The samples were vacuum-dried for 18 h and finally sputter-coated with a 20 nm gold layer before microscopical analysis.
Antigen stability in simulated production conditions
In order to assess the stability of antigen in simulated production conditions, antigen was subjected to an acetone-to-water ratio of 3:1 used during the preparation of nanoparticles at 40°C and the conformation of TT (0.1 mg/ml of water) was studied with circular dichroism spectroscopy (CD) and fluorescent λmax scan by spectrofluoremetry. TT in the same concentration in PBS pH 7.4 was taken as control. Antigen in an acetone-to-water ratio of 3:1 at temperature of 40°C and pH of 3 was incubated for 5 h before spectroscopic measurements. Measurements were performed on a spectrofluorometer (RF-5301PC spectrofluorophotometer, Shimadzu) using 2 ml sample volume in a 1 cm path length quartz cell. Fluorescence spectra were corrected by subtracting a baseline spectrum of PBS pH 7.4, incubated under the same conditions as required during the preparation of GNPs. An excitation wavelength of 280 nm was used. CD measurements were performed to gain more specific insight into conformational changes of TT under stressed conditions. Typically, 0.5 ml of 200 μg/ml toxoid in a 10 mM saline-free phosphate buffer (PB) at pH 7.4 was analyzed (Jasco J-720, Japan Spectroscopic, Tokyo, Japan).
In vivo immunological response
Female BALB/c mice of 7–9 weeks age were housed in groups of six, with free access to food and water, and were withdrawn of any food intake 3 h before immunization. The study protocol, as approved by Institutional Animals Ethics Committee of Dr Hari Singh Gour University, was followed. The studies were carried out as per the guidelines of the Council for the Purpose of Control and Supervision of Experiments on Animals (CPCSEA), Ministry of Social Justice and Empowerment, Government of India. To evoke an immune response, a single dose of 5 Lf of nanoparticulate antigen was injected subcutaneously (s.c) followed by a booster dose at the 4th week. The control group, however, received an equivalent dose of alum-adsorbed TT (positive control), plain TT, and blank nanoparticles subcutaneously and a booster after 4 weeks of primary immunization. Immunization volume in each case was 200 µl.
Sample collection
Blood was collected by retro-orbital puncture (under mild ether anesthesia) at 0, 2, 4, 6, and 8 weeks. Here 0 week represents the sampling prior to the booster dose and subsequent weeks (2, 4, 6, and 8) represents the time of sample collection following the booster dose. This was done to compare primary and secondary immunization. Sera were stored at −40°C until tested by ELISA for antibody. Animals were sacrificed at 0 and 8 weeks of booster immunization and spleens were removed for the determination of endogenous cytokine levels (interferon-γ (IFN-γ) and interleukin-2 (IL-2)).
Measurement of specific IgG
Anti-TT IgG antibodies in mice sera were estimated as reported previously (CitationRaghuvanshi et al., 1998) with slight modification by commercially available ELISA Kit (Bangalore Genei, India). IgG1 and IgG2a isotypes were determined using sigma isotyping kit (Sigma-Aldrich Pvt. Ltd., St. Louis, MO, USA). Briefly, micro titer plates (Nunc Immuno Plate® Fb96 Mexisorp, NUNC) were coated with 1 µg of TT in 100 µl of PBS (50 mM, pH 7.4) and the plate was incubated for 90 min at 37 ± 1°C. The plate was then washed with PBS containing 0.05% Tween 20 (PBS-T) and blocked with 2% (w/v) BSA solution for 1 h at 37°C and washed thrice with PBS-T. Then 100 μl of serum samples (diluted with blocking buffer) of different dilutions were added to the wells in duplicate and the plate was incubated for 90 min at 37 ± 1°C. Anti-TT IgG present in sera was estimated using 1/100 dilution as the first dilution of serum. After 2 h incubation and six times washing with PBS-T, 100 μl of horseradish peroxidase conjugated goat anti-mouse IgG was added to each well and incubated again for 2 h. The plate was then washed six times and anti-IgG antibodies were detected using 100 μl of 3,3′,5,5′-tetramethylbenzidine (TMB) as substrate. After 15–20 min, 5N H2SO4 (50 µl/well) was added to stop the reaction and the color produced was measured at 450 nm using ELISA plate reader (Sigma-Aldrich Pvt. Ltd., St. Louis, MO, USA). Further, gelatin and aminated gelatine-specific IgG response were assessed for GNPs and AGNPs were compared with alum adsorbed gelatin as a positive control in a similar manner wherein gelatin or aminated gelatin was used to coat the wells instead of TT.
For IgG1 and IgG2a antibody sub-classes, a similar protocol which is described above was followed with OPD (Sigma-Aldrich Pvt. Ltd., St. Louis, MO, USA) as substrate and reading was obtained at 492 nm. The end-point titre was expressed as the logarithm of the reciprocal of the last dilution, which gave an optical density at 450 or 492 nm above the optical density of negative control.
Estimation of cytokine levels
Endogenous levels of IL-2 and IFN-γ in mouse spleen homogenates were measured by using separate ELISA. Spleen homogenates were prepared by the method reported by CitationNakane et al. (1992) with slight modifications. Briefly, spleen was weighed and homogenized in ice-cold PBS containing 1% CHAPS (Sigma) and 10% (w/v) homogenates were obtained with the help of tissue homogenizer (York, New Delhi, India). Homogenates were incubated in an ice-bath for 1–2 h at a temperature below 0°C and the insoluble matter was allowed to settle down. The supernatants were centrifuged at 2000 g for 20 min and the clear solution was used for cytokine estimation by selected ELISA method.
Statistical analysis
Statistical analysis was performed on the data obtained in the in vitro and in vivo studies by one-way analysis of variance (ANOVA) with Tukey–Kramer multiple comparisons post-test using GraphPad InStat™ software (GraphPad Software Inc., San Diego, CA). For comparison between two groups, Student’s t-test was applied. Throughout, the level of significance was chosen as less than 0.05 (i.e. p < 0.05). The post-hoc test was performed only if findings of the ANOVA were significant.
Results and discussion
Preparation of plain gelatin nanoparticles
In the present study gelatin nanoparticles were prepared by a two-step desolvation technique. In the two-step desolvation technique the higher molecular weight (HMW) fraction is separated by a first desolvation step and the particles are prepared in a second desolvation step. The procedure produced homogeneous gelatin nanoparticles which were spherical and in the size range of 150–300 nm. The preparation of GNPs using a two-step desolvation procedure showed much less aggregation as compared to the earlier described one-step desolvation method (CitationCoester et al., 2000). The sediment collected following the first desolvation step showed an accumulation of the high molecular weight fraction of the gelatin, whereas in the supernatant the lower molecular weights prevailed. Therefore, it is concluded that the removal of the low molecular weight gelatin fraction in the supernatant after the first desolvation step not only reduced the formation of aggregates during cross-linking because of an enhanced stability of particles formed before cross-linking, but also prevented further secondary aggregation and flocculation of particles during storage (CitationCoester et al., 2000). TT-loaded GNPs had the size of 255 ± 11 nm, percentage entrapment efficiency of 91.4 ± 5.17, and PDI of 0.0819 ± 0.012 ().
Table 1. The average particle size, EE, PDI, and percentage amine group content of unlabeled gelatin nanoparticles and FITC-TT loaded GNPs and AGNPs. Values are expressed as mean ± SD (n = 3).
Preparation of aminated gelatin nanoparticles and quantification of surface-free amine groups
For the preparation of aminated gelatin nanoparticles, amination of gelatin was carried out with ethylene diamine. Investigations regarding the reaction time revealed that the TNBS protein coupling reaction reached the maximal level after 6 h. However, the increase between 2 and 6 h was insignificant. As a result, the reaction time was fixed to 2 h. Aminated gelatin nanoparticles (AGNPs) were prepared by using the optimized parameters for GNPs. AGNP had the size of 242 ± 15 nm and percentage entrapment efficiency of 92.5 ± 5.21. Surface-free amine groups of the nanoparticles were determined by a TNBS colorimetric assay in order to confirm the increase in amine groups after reaction. TNBS reacts with the amine groups at a pH of 8.5 under formation of a colored TNBS-protein conjugate. After incubation of the particulate system with TNBS, the nanoparticles were separated from unreacted TNBS by centrifugation, and the amount of unreacted TNBS was determined spectrophotometrically at 349 nm. The determination of free amino groups on the surface of nanoparticles cross-linked using 250 μl of glutaraldehyde showed that ~ 12 ± 2.3 and 40.5 ± 4.2% of the amino groups were available for GNPs and AGNPs, respectively. This is in accordance with the results reported previously (CitationRubino et al., 1993; CitationWeber et al., 2000). The narrow size distribution of the nanoparticles indicates that the glutaraldehyde has reacted with amino groups on the same particle rather than linking two particles together. This might be due to the zeta potential of the particles (zeta potential for GNPs and AGNPs were found to be +14 and +23, respectively), which prevents them from coming close together. The degree of cross-linking and therefore the degree of free amino groups are important factors because they have an impact on the biodegradability of the particles as described previously (CitationRubino et al., 1993; CitationJameela & Jayakrishnan, 1995).
Characterization of plain and aminated gelatin nanoparticles
Quality control of the resulting nanoparticles was carried out by dynamic light scattering (DLS) measurements. The sizes found were 215, 255, and 242 nm for blank GNPs and antigen-loaded GNPs and AGNPs, respectively, with a narrow polydispersity index of 0.062, 0.0819, and 0.0921, respectively (). Although the polydispersity index obtained was increased on antigen entrapment, still the obtained PDI value was well within the acceptable limit (< 0.1).
We additionally analyzed this nanoparticle batch by SEM to visualize the particles and investigate their morphology. SEM analysis demonstrated that AGNPs prepared by the optimized two-step desolvation procedure are colloidal spheres with a smooth surface morphology ().
Entrapment efficiency and in vitro release of FITC-TT from nanoparticles
For entrapment and release studies FITC-TT was used. It is easy to analyze the drug entrapment and release with this fluorescent dye using fluorescence spectrophotometry since conventional colorimetric assays such as BCA and Bradford’s methods would give erroneous results due to the presence of soluble gelatin which would also give color in the dye binding assay. shows the percentage entrapment efficiency (EE) of FITC-TT in the prepared formulations. The EE of FITC-TT in gelatin nanoparticles might be due to the formation of a strong inter-penetrating network between gelatin and TT. FITC-TT would, therefore, be encapsulated during the coacervation phase at an acidic pH in the last desolvation step. The release of FITC-TT was performed in PBS alone as well as in the presence of collagenase at 37°C (). Release studies of GNPs and AGNPs in PBS showed a very low release of ~ 0.6% and 1.5%, respectively, after 48 h. The slow diffussional release may be attributed to glutaraldehyde cross-linking which forms an inter- and intra-molecular cross-linking mostly on the surface of the gelatin nanoparticles, leading to hardening of the nanoparticles that causes a lower swelling and possibly the decrease in TT-FITC release. In contrast, a release of 78.2% (± 3.6%, n = 3) and 61.1% (±3.6%, n = 3) after 48 h was achieved with the addition of collagenase to GNPs and AGNPs, respectively. The lower degradation and release obtained with AGNPs in comparison to GNPs (in the presence of enzyme) may be attributed to a greater cross-linking due to the availability of more amine groups in comparison to GNPs. Biodegradation profile may have greater implications in the in vivo fate of the nanoparticles (CitationJameela & Jayakrishnan, 1995). A complete release of FITC-TT was not seen even after 72 h, as there might be some antigen that would have still linked with parts of the gelatin/gelatin nanoparticles due to protein–protein interaction. Similar results were obtained by CitationCoester et al. (2006) when trypsin was used. The release characteristics observed were, therefore, biphasic. Since the core is not cross-linked, the gelatin present in the core gets solubilized in the buffer, but due to the mechanical barrier provided by cross-linking, the antigen and the matrix are released slowly. The initial burst release is suppressed due to cross-linking. The rapid release observed with gelatin (in the presence of enzyme) could be due to the uncoiling of its triple helical structure (CitationTzafriri et al., 2002).
Antigen stability in simulated production conditions
Fluorescence spectroscopy was used to assess the conformation of tetanus toxoid. The purified toxoid starting material had a fluorescence maximum (Fmax) of 330–331 nm, but tetanus toxoid at an acetone-to-water ratio of 3:1 at temperature of 40°C had a red-shifted emission peak at 337–338 nm (). This indicates that the toxoid was partly unfolded in the given set of conditions.
Figure 3. Far-UV CD spectra of tetanus toxoid in acetone to water ratio of 1:3 at 40oC after 5h of incubation period.
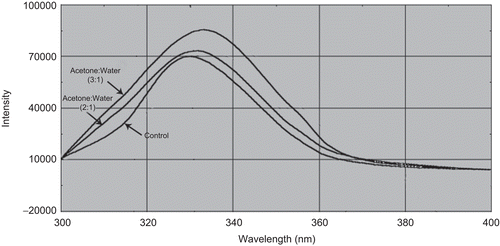
Conformational stability of TT solution was further studied by far UV CD (). After 5 h of incubation at 40°C, the ellipticity minimum shifted from 208 to 213 nm, indicating a slight increase in β-sheet structure. It has been observed that α-helices may undergo structural transitions in acidic solutions and at elevated temperatures, whereas β-sheets are more stable (CitationJohnson, 1995).
Immunological response
The enhancement of immune responses by encapsulated HGG, a model antigen in gelatin microparticles prepared by emulsification method, has been reported previously by CitationNakaoka et al. (1995). Our work is different from Nakaoka et al. in the following respect. A double desolvation method was used in our study, which is proposed as a protein-friendly method due to the use of lower temperature and shear. Exceptional size homogeneity can be obtained by double desolvation method due to removal of low molecular weight gelatine, which is crucial from a pharmaceutical perspective for further batch-to-batch uniformity, scale-up, and commercialization of nanoparticles. Washing and purification steps in emulsification method make it a tedious process. Further, surface modification of gelatin was carried out to prepare aminated gelatin nanoparticles with slow release and reduced biodegradation profile.
The antigen delivery ability of the gelatin and aminated gelatin nanoparticles administered subcutaneously for the induction of systemic antibody responses was evaluated. The gelatin nanoparticles (single and booster injections) and aminated gelatin nanoparticles (single and booster injections) were compared with alum adsorbed TT. Further, gelatin and aminated gelatine-specific IgG response were assessed for GNPs and AGNPs and were compared with alum adsorbed gelatin and aminated gelatine, respectively, as a positive control. The serum anti-TT titre obtained after subcutaneous (s.c) administration of both formulations were comparable with titre recorded after alum-TT was administered subcutaneously (p > 0.05) (). It can be inferred from the data that all the nanoparticulate formulations effectively elicited systemic immune response (IgG level). AGNPs and GNPs in booster dose demonstrated comparable immune response to alum-TT (booster) after 8 weeks post-immunization. In contrast, antibody titres recorded for single dose of GNPs and AGNPs were significantly lower as compared to both the booster dose formulations after 8 weeks. Blank particles did not show any detectable antibody titre. Antibody titre recorded for nanoparticulate formulations were significantly higher than plain antigen, which demonstrates that microencapsulation within gelatin matrices has a significant effect on the immune response against TT.
Figure 5. Serum anti-TT profile of mice immunized subcutaneously with different formulations. Values are expressed as mean±S.D. (n = 6) 55x35mm (300 × 300 DPI)
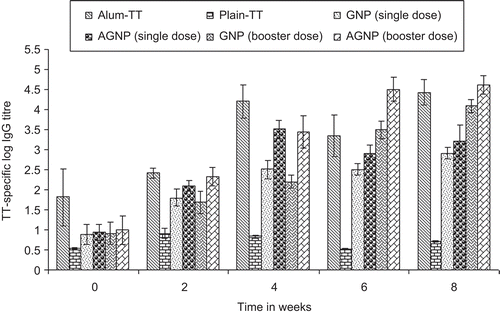
The IgG1 and IgG2a anti-TT titres determined by ELISA are shown in , and are consistent with previous data demonstrating that alum induces a Th2 response, characterized by very low IgG2a responses. A weak IgG2a antibody titre was elicited by alum-TT after a booster dose. In contrast, mice injected with TT entrapped GNPs and AGNPs produced high levels of IgG2a. The consistently higher IgG2a anti-TT titres obtained, in both single (p < 0.001 and p < 0.01, respectively) and booster dose (p < 0.001) responses, in mice treated with AGNPs and GNPs than in mice treated with alum-TT was demonstrated after 8 weeks. Alum produced high IgG1 titres after the boost and was found to be comparable with booster dose of both the nanoparticulate formulations. These results suggest that both adjuvants modulate differentially the type of immune response to immunization with TT. Alum induces almost only a Th2 response, while both the nanoparticulate formulations elicit both Th1 and Th2 responses, as demonstrated by the strong IgG2a and IgG1 anti-TT antibody responses.
Figure 6. Log IgG1 and Log IgG2a titre of different formulations after s.c injection at 0 and 8 weeks. Values are expressed as mean±S.D. (n = 6) 177×105mm (72 × 72 DPI)
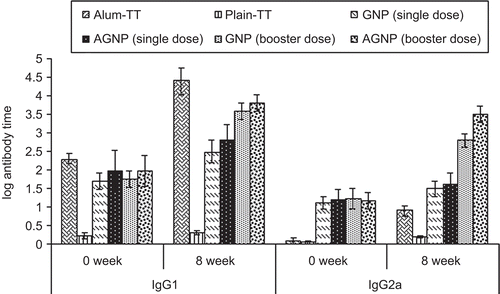
Further, as depicted in , all serum samples of immunized mice showed insignificant anti-gelatin and anti-aminated gelatin IgG titres compared to alum-gelatin and alum-AG (positive control) at 0 weeks and at 8 weeks for both the booster dose formulations. Hence, no undesired immunogenicity against the carrier matrix, i.e. gelatin, was induced. The gelatin nanoparticles therefore have no intrinsic mitogenicity and little (if any) ability to enhance the antibody response to an antigen unless it is incorporated into the gelatin matrix. Moreover, significant endogenous cytokine levels (IL-2 and IFN-γ) in spleen homogenates were found when AGNPs and GNPs (p < 0.001 and p < 0.01) were administered s.c in comparison to alum-TT administered subcutaneously at 0 weeks and at 8 weeks of booster dose immunization with different formulations ( and ). Aminated nanoparticles demonstrated significant IgG levels as compared to their plain counterparts after 8 weeks. IL-2 and IFN-γ levels were significantly higher in the case of AGNPs as compared to GNPs (p < 0.001 and p < 0.05). Both Th1-dependent cytokines and their high levels are evidenced for the strong cell-mediated immune response elicited by gelatin-based formulations administered subcutaneously which substantiates the data obtained for anti TT IgG2a titre. The endogeneous cytokine levels demonstrated by the nanoparticles were not due to the presence of endotoxins as the formulations did not have any impurity with pyrogens (confirmed by endotoxin assay).
Figure 7. Gelatin and aminated gelatin specific IgG antibody response with selected formulation loaded with TT at 0 and 8 week following s.c administration. Values are expressed in terms of log10 IgG antibody titers 73 × 48mm (200 × 200 DPI)
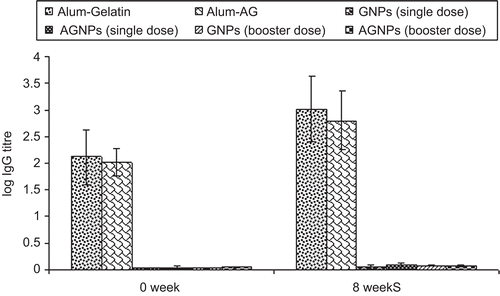
Figure 8. Interferon-γ levels in the spleen of mice immunized with different formulations. Values are expressed as mean±S.D. (n=6) 47 × 31mm (300 × 300 DPI)
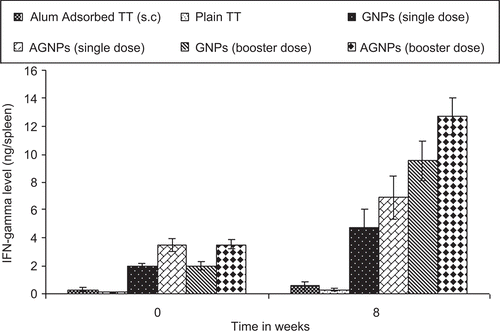
Figure 9. IL-2 level (ng/spleen) in spleenocytes supernatant at 0 and 8 weeks after s.c injection 49×32mm (300 × 300 DPI)
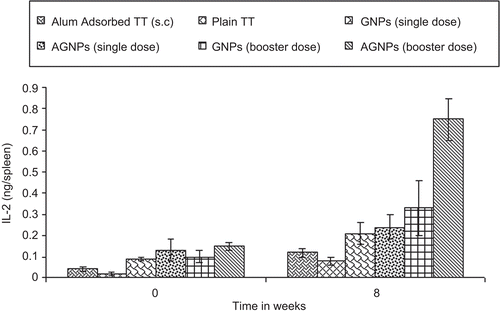
Booster dose significantly increased the immune response (both antibody and cytokine levels recorded at 2, 4, 6, and 8 weeks) compared to primary immunization (recorded at 0 weeks).
The prepared nanoparticulate formulations are, therefore, advantageous as an immunological adjuvant in magnitude and duration and were found to be comparable to conventional alum adsorbed formulations. The incorporated TT cannot leach out unless enzymes like collagenase are present. Professional antigen presenting cells and dendritic cells (DCs) in particular, naturally phagocytose bacteria, viruses, and other micro-organisms and process their proteins for antigen presentation to T-cells for the induction of antigen-specific immune responses. Nanoparticles are within the same size range as micro-organisms and thus are preferentially phagocytosed by these cells. Hence, particulate vaccine delivery systems have proven to be advantageous for sub-unit vaccines based on proteins, peptides, and DNA (CitationRaychaudhuri & Rock, 1998; CitationSingh & O’ Hagan, 2002).
Subcutaneous administration is considered as the most appropriate route for delivering an antigen to secondary lymphoid organs such as lymph nodes where the adaptive immunity is initiated (CitationOussoren & Storm, 2001). Therefore in the present study subcutaneous route was used for administration. Particle size of nanoparticles was found to be optimal for uptake of particles by professional antigen presenting cells (APCs) which include macrophages and dendritic cells. Particle diameters of 0.5 µm and below are optimal for DC uptake (CitationFoged et al., 2005). However, particles in the size range of 1–15 µm are only phagocytosed by a minority of the DC as reported in the literature (CitationReece et al., 2001). Moreover, in a recent finding it has been shown that gelatin nanoparticles are efficiently taken up by dendritic cells in vitro (CitationCoester et al., 2006). As the gelatin nanoparticles are degraded by lysosomal enzymes (e.g. collagenase) after uptake by antigen-presenting cells, TT will be released intracellularly after the enzymatic degradation of nanoparticles. However, there is a possibility that antigenicity of TT released could be different from that of native molecules due to the glutaraldehyde cross-linking. Incorporation of the antigen into the gelatin nanoparticles could deliver a large amount of antigen to APCs, leading to an antigen-specific immune response. Targeting of antigen carrier complexes to APCs may result in an enhancement of specific humoral and cellular immune responses. In addition, the gelatin nanoparticles could induce a strong secondary response. The secondary response is dependent on a population of memory lymphocytes. In case of AGNPs, cationization of particles (CitationKwon et al., 2005) due to surface amine groups might have contributed to the enhanced adjuvant activity. All the above-mentioned factors would contribute to the immunopotentiation property of the nanoparticles in a time-dependent fashion. At this time it is difficult to say conclusively which of the factors is dominant in improving the immune response. Although the reason for the effective generation of the immune response by the nanoparticles is not clear at present, the above findings indicate that both gelatin and aminated nanoparticles are promising adjuvants to enhance both humoral and cellular responses to antigen.
Safety of carrier formulations is essential to justify the further development of TT-loaded gelatin nanoparticles. A major concern is the possible development of an immune response against the carrier that would render subsequent applications of the same carrier ineffective. In this study we have shown that the application of TT-loaded gelatin and aminated gelatin nanoparticles do not result in the generation of an antigen-specific immune response to gelatin and AG, the matrix material of the nanoparticles, demonstrated by the low level of gelatin and AG-specific IgG response.
Conclusion
Gelatin and aminated gelatin nanoparticles could be a highly valuable carrier system for immunization against TT. It can be concluded that the prepared vaccine formulations produced both Th1 and Th2 type of immunity and could be extended to human use as future vaccines. However, toxicity issues have to be addressed in future experimental set-ups before gelatin nanoparticles get due recognition as a carrier adjuvant for the development of vaccines for other diseases.
Declaration of Interest
The author MSS is indebted to Head, Department of Pharmaceutical Sciences, Dr H. S. Gour University, Sagar for providing all the facilities for the research work, Serum Institute of India, Pune for the gift sample of TT, Director, National Institute of Pharmaceutical Education and Research (NIPER), Mohali for CD spectroscopy studies and AIIMS (All India Institute of Medical Sciences), New Delhi for SEM studies.
References
- Aguilar, J.C., Rodriguez, E.G. (2007). Vaccine adjuvants revisited. Vaccine. 25:3752–62.
- Allison, A.C., Byars N.E. (1991). Immunological adjuvants: desirable properties and side-effects. Mol Immunol. 28:279–84.
- Aprile, M.A., Wardlaw, A.C. (1966). Aluminum compounds as adjuvants for vaccine and toxoids in man: a review. Can J Pub Health. 57:343–54.
- Clemmenson, O., Knudsen, H.E. (1980). Contact sensitivity to aluminium in a patient hyposensitized with aluminium precipitated grass pollen. Contact Dermatitis. 6:305–8.
- Coester, C., Nayyar, P., Samuel, J. (2006). In vitro uptake of gelatin nanoparticles by murine dendritic cells and their intracellular localization. Eur J Pharm Biopharm. 62:306–14.
- Coester, C.J., Langer, K., Von Briesen, H., Kreuter, J. (2000). Gelatin nanoparticles by two step desolvation - a new preparation method, surface modifications and cell uptake. J Microencapsul. 17:187–93.
- Collier, L.H., Polakoff, S., Mortimer, J. (1979). Reactions and antibody responses to reinforcing doses of adsorbed and plain tetanus vaccines. Lancet. 1:1364–8.
- Copland, M.J., Baird, M.A., Rades, T., McKenzie, J.L., Becker, B., Reckd, F., Tyler, P.C., Davies, N.M. (2003). Liposomal delivery of antigen to human dendritic cells. Vaccine. 21:883–90.
- Erodohazi, M., Newman, R.L. (1971). Aluminium hydroxide granuloma. Br Med J. 3:621–3.
- Fields, R. (1971). The measurement of amino groups in proteins and peptides. Biochem J. 124:581–90.
- Foged, C., Brodin, B., Frokjaer, S., Sundblad, A. (2005). Particle size and surface charge affect particle uptake by human dendritic cells in an in vitro model. Int J Pharm. 298:315–22.
- Frost, L., Johansen, P., Pedersen, S., Veien, N., Ostergaard, P.A., Nielsen, M.H. (1985). Persistent subcutaneous nodules in children hyposensitized with aluminium-containing allergen extracts. Allergy. 40:368–72.
- Gupta, R.K., Chang, A.C., Griffin, P., Rivera, R., Siber, G.R. (1996). In vivo distribution of radioactivity in mice after injection of biodegradable polymer nanoparticles containing 14C-labeled tetanus toxoid. Vaccine. 14:1412–6.
- Jaganathan, K.S., Rao, Y.U.B., Singh, P., Prabakaran, D., Gupta, S., Jain, A., Vyas, S.P. (2005). Development of a single dose tetanus toxoid formulation based on polymeric microspheres: a comparative study of poly(d,l-lactic-co-glycolic acid) versus chitosan microspheres. Int J Pharm. 294:23–32.
- Jameela, S.R., Jayakrishnan, A. (1995). Glutaraldehyde crosslinked chitosan microspheres as a long acting biodegradable drug delivery vehicle: studies on the in vitro release of mitoxantrone and in vivo degradation of microspheres in rat muscle. Biomaterials. 16:769–75.
- Johnson, W.C. Jr. (1995). Secondary structure of proteins through circular dichroism spectroscopy. Annu Rev Biophys Biophys Chem. 14:145–66.
- Kuijpers, A.J., Engbers, G.H., Krijgsveld, J., Zaat, S.A., Dankert, J., Feijen, J. (2000). Crosslinking and characterisation of gelatin matrices for biomedical applications. J Biomaterials Sci Polym Edi. 3:225–43.
- Kwon, Y.J., Standley, S.M., Goh, S.L., Fréchet, J.M.H. (2005). Enhanced antigen presentation and immunostimulation of dendritic cells using acid-degradable cationic nanoparticles. J Contr Rel. 105:199–212.
- Matsuhasi, T., Ikegami, H. (1982). Elevation of levels of IgE antibody to tetanus toxin in individuals vaccinated with diphtheria-pertussis-tetanus vaccine. J Infect Dis. 146:290.
- Nagel, J., Svec, D., Water, T., Fireman, P. (1977). IgE synthesis in man I. Development of specific IgE antibodies after immunization with tetanus-diphtheria (TD) toxoids. J Immunol. 18:334–41.
- Nakane, A., Numata, A., Minagawa, T. (1992). Endogenous tumor necrosis factor, interleukin-6, and gamma interferon levels during Listeria monocytogenes infection in mice. Infect Immun. 60:523–8.
- Nakaoka, R., Tabata, Y., Ikada, Y. (1995). Potentiality of gelatin microsphere as immunological adjuvant. Vaccine. 13:653–61.
- Nixon, A., Zaghouani, H., Penney, C.L., Lacroix, M., Dionne, G., Anderson, S.A., Kennedy, R.C., Bona, C.A. (1992). Adjuvanticity of stearyl tyrosine on the antibody response to peptide 503–535 from HIV gp160. Viral Immunol. 59:141–50.
- Oussoren, C., Storm, G. (2001). Liposomes to target the lymphatics by subcutaneous administration. Adv Drug Deliv Rev. 50:143–56.
- Raghuvanshi, R.S., Goyal, S., Singh, O., Panda, A.K. (1998). Stabilization of dichloromethane-induced protein denaturation during microencapsulation. Pharm Dev Technol. 3:269–76.
- Raychaudhuri, S., Rock, K.L. (1998). Fully mobilizing host defense: building better vaccines. Nat Biotechnol. 11:1025–31.
- Reece, J.C., Vardaxis, N.J., Marshall, J.A., Crowe, S.M., Cameron, P.U. (2001). Uptake of HIV and latex particles by fresh and cultured dendritic cells and monocytes. Immunol Cell Biol. 79:255–63.
- Rubino, O.P., Kowalsky, R., Swarbrick, J. (1993). Albumin microspheres as a drug delivery system: relation among turbidity ratio, degree of cross-linking, and drug release. Pharm Res. 10:1059–65.
- Schirmbeck, R., Melber, K., Mertens, T., Reimann J. (1994). Antibody and cytotoxic T-cell responses to soluble hepatitis B virus (HBV) S antigen in mice: implications for the pathogenesis of HBV-induced hepatitis. J Virol. 68:1418–25.
- Seki, T., Kanbayashi, H., Nagao, T., Chono, S., Tomita, M., Hayashi, M., Tabata, Y. (2005). Effect of aminated gelatin on the nasal absorption of insulin in rats. Biol Pharm Bull. 28:510–4.
- Singh, M., O’Hagan D.T. (2002). Recent advances in vaccine adjuvants. Pharm Res. 6:715–28.
- Tabata, Y., Ikada, Y. (1998). Protein release from gelatin matrixes. Adv Drug Del Rev. 3:287–301.
- Traquina, P., Morandi, M., Contorni, M., Van Nest, G. (1996). MF59 adjuvant enhances the antibody response to recombinant hepatitis B surface antigen vaccine in primates. J Infect Dis. 174:168–75.
- Tzafriri, A.R., Bercovier, M., Parnas, H. (2002). Reaction diffusion model of the enzymatic erosion of insoluble fibrillar matrices. Biophys J. 83:776–93.
- Vassilev, T.L. (1978). Aluminium phosphate but not calcium phosphate stimulates the specific IgE response in guinea-pigs to tetanus toxoid. Allergy. 33:155–9.
- Weber, C., Coester, C., Kreuter, J., Langer, K. (2000). Desolvation process and surface characterization of protein nanoparticles. Int J Pharm. 194:91–102.
- White, R.G., Coons, A.H., Connolly, J.M. (1955). Studies on anti- body production III - The alum granuloma. J Exp Med. 102:73–82.