Abstract
High costs of production and relatively short serum half-life of mammalian cell-derived recombinant human erythropoietin (rHuEpo) necessitate finding and developing superior hosts/technologies for more efficient production of longer-acting erythropoietic agents. With these aims, we provide the first report on reductive alkylation of low-cost P.pastoris-expressed rHuEpo (PPEpo) with PEG-aldehyde. The PCR-amplified cDNA of native rHuEpo was cloned into the pPICZαA vector and transformed into the yeast Pichia pastoris. The best expressing transformant was selected and employed for secreted-expression of PPEpo using the standard protocols. Purified PPEpo was N-terminally PEGylated with 20-kDa mPEG-propionaldehyde in a low pH (5) condition. The in vitro and in vivo biological activities of purified mono-PEGylated PPEpo was evaluated by the UT-7 cells proliferation assay and normocythaemic mice assay, respectively. Pharmacokinetic parameters were determined following intravenous administration of Epo proteins in rabbits. While PPEpo showed a higher in vitro bioactivity compared to rHuEpo, no in vivo efficiency was determined for PPEpo. However, the in vivo activity of PEG-PPEpo conjugate was comparable to that of rHuEpo. Pharmacokinetic studies showed that the terminal half-life and mean residence time of PEG-PPEpo were increased approximately 4-fold and 6.5-fold respectively, compared with those of PPEpo. The results indicate that N-terminal PEGylation of Pichia-expressed Epo could be considered as a promising approach for generating cost-effective and long-acting erythropoiesis-stimulating agents.
Introduction
Erythropoietin (Epo) plays an important role in regulating the level of circulatory erythrocytes through stimulating the proliferation and differentiation of erythroid precursor cells into mature red blood cells (CitationElliott et al., 2008). Recombinant Human Erythropoietin (rHuEpo), produced by Chinese Hamster Ovary (CHO) cells, is used clinically for the treatment of anemia associated with chronic renal failure, chemotherapy and AIDS (CitationMarkham and Bryson, 1995). The main advantage of CHO cells over the other common expression systems such as bacteria and yeasts is the ability of the former to execute many human-like post modifications in rHuEpo particularly the sialylation of terminal galactose residues in sugar moieties. However, the production of recombinant proteins in CHO cells is associated with some intrinsic drawbacks such as high cost and low efficiency. Furthermore, CHO cell-expressed Epo still suffer from a rather short half life (approximately 6 to 8 hours) which necessitates the administration of 2 to 3 times per week in order to obtain an optimum therapeutic response (CitationMacdougall et al., 1991). To address these problems, considerable attempts have been made to find and develop superior hosts/technologies for more efficient production of long-acting erythropoiesis-stimulating agents.
With the advantages of both prokaryotic and eukaryotic systems, the methylotrophic yeast Pichia pastoris has been developed for high yields and low costs production of a large number of therapeutic proteins and could be considered as an appropriate alternative host for more efficient expression of rHuEpo (CitationLi et al., 2007). Although CitationWang et al. (2010) provided promising results of cost-effective production of E.coli-derived rHuEpo, but in contrast to E.coli, P. pastoris often allows the correctly folded recombinant protein to be secreted in large quantities when it does not secrete significant amounts of intrinsic proteins, a phenomenon that facilitates downstream purification steps (CitationDaly and Hearn, 2005). However, the glycosylation pattern of Epo expressed by P.pastoris is highly different with that of mammalian cells and the former does not seem to add terminal sialic acids to sugar residues (CitationCelik et al., 2007) It is known that the serum residency and in vivo activity of Epo is greatly dependent on the presence and extent of terminal sialylation of oligosaccharide residues (CitationEgrie et al., 2003).
The modification of proteins with PolyEthylene Glycol (PEG) is a well-established technique for improving biological and pharmacokinetic characteristics of therapeutic proteins (CitationHamidi et al., 2006; CitationJevsevar et al., 2010) and PEGylation may possibly play the essential role of native glycosylation in establishing the in vivo activity of non-sialilated Epo proteins (CitationWang et al., 2010; CitationYesland, 2008). However, the lack of selectivity in the attachment of PEG chains to Epo (that occurs typically at lysine residues and/or at the N-terminal amino acid) could lead to a heterogeneous product with significant loss in biological activity. It is known that while the N-terminal amino acid of Epo is not involved in protein-receptor interactions (CitationBoissel et al., 1993; CitationLong et al., 2006), four lysine residues, i.e. K20, K45, K97, and K152 are crucial for receptor binding (CitationBoissel et al., 1993; CitationElliott et al., 1997) and modification of these lysine amino acids could give rise to a severe reduction in the in vitro activity (CitationWojchowski and Caslake, 1989; CitationLeist et al., 2004). With the aim of avoiding this major disadvantage of PEGylation we describe the reductive alkylation of Pichia-expressed Epo (PPEpo) with 20-kDa mPEG-propionaldehyde in a low pH condition which almost selectively targets the N-terminal amine over the other amines in the protein (CitationKinstler et al., 1999). We demonstrate the utility of this approach for increasing the in vivo activity and plasma residency of PEGylated PPEpo with minimally reduction in the in vitro biological activity.
Materials and methods
Construction of secreted-expression vector
A synthetic cDNA (Bio Basic Inc, Ontario, Canada) encoding the Epo gene for 166 native amino acids (NCBI accession no. NM_000799) in tandem with the DNA sequence of six histidine amino acids at the C-terminal of Epo (C-6xHis-tag) was amplified by polymerase chain reaction (CitationHorton, 1993) and using the designed forward (F:5′ CTCGAGAAAAGAGCCCCACCACGCCTCATC3′) and reverse (R:5′ TCTAGATTATCAATGATGATGATGATGATGTCTGTCCCCTGTCCT3′) primers (5′ and 3′ XhoI and XbaI restriction sites are underlined, respectively) (TAG Copenhagen, Denmark). For secreted-expression of Epo in P.pastoris, the plasmid PICZαA was employed as the shuttle vector. The amplified products were gel-purified (QIAquick Gel Extraction Kit) and ligated separately into XhoI- and XbaI-digested pPICZαA vector (Invitrogen). By using of XhoI and XbaI restriction enzyme sites, the cDNA of Epo was cloned in frame with alcohol oxidase (AOX1) transcription/translational cassette and downstream of the α-factor sequence and immediately after the Kex2 signal cleavage site in order to express and secrete Epo into the culture media with a native N-terminus. Furthermore, two stop codons were placed immediately after the C-6xHis-tag codons for preventing the insertion of any vector derived amino acids in the C-terminal (). The constructed vector was confirmed via PCR, restriction analyses and DNA sequencing reactions.
Figure 1. Schematic diagram of recombinant pPICZαA harboring HuEpo gene (Epo/PICZαA: 4.1kb). XhoI and XbaI denote to the restriction sites employed for directional cloning of Epo gene into pPICZαA under control of AOX1 promoter, down stream of secretion signal (α-factor) and cleavage sequence (Kex2). AOX1 TT, 6xHis and Stop denote to the transcription termination sequence, His tag and stop codons, respectively.
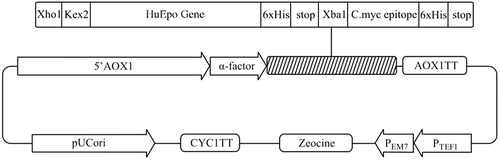
Yeast expression of PPEpo
The purified PPEpo/PICZαA plasmid was linearized with SacI enzyme (Fermentas) and electroporated (Bio-Rad Gene Pulser) into the yeast P. pastoris, wild-type host strain X-33 (Invitrogen) according to supplier’s instructions (Easy Select Pichia Expression manual, Invitrogen). Zeocin-resistant transformants were analyzed by western blot for selecting the best expression clone. A single selected colony was inoculated into 25 ml of BMGY medium [1% (w/v) yeast extract, 2% (w/v) peptone, 1.34% (w/v) YNB, 4 × 10-5 % (w/v) biotin, 1% (v/v) glycerol and 0.1M potassium phosphate, pH 6.0] in a 250 ml Erlenmeyer flask and growth allowed to proceed at 28–30°C (200 rpm) for 16–18 hours. Subsequently, cells were harvested by centrifugation (3000 g for 4 minutes) and the pellet resuspended in approximately 200 ml BMMY medium [1% (w/v) yeast extract, 2% (w/v) peptone, 1.34% (w/v) YNB, 4 × 10-5 % (w/v) biotin, 0.5% (v/v) methanol and 0.1M potassium phosphate, pH 6.0] in a 1 liter baffled erlenmeyer flask to an OD600 = 1 and incubated at 28–30°C (250 rpm) in a floor shaking incubator for 72 h. To maintain induction, sterile 100% methanol was added to a final concentration of 0.5 % every 24 hours during the expression. After 72 h, the medium was centrifuged at 10000 g for 10 min and the pellet was discarded.
Purification of expressed PPEpo
The supernatant was concentrated by exploiting Pellicon XL50 ultrafiltration cassette (Millipore, USA) equipped with Ultracel membrane (MW Cut-off of 10 kDa). Subsequently, the sample was loaded on an ion metal affinity chromatography (IMAC) column (His Trap HP columns, GE Healthcare Inc.) charged with Ni2+ ion and equilibrated with 20 mM sodium phosphate buffer containing 0.5 M sodium chloride, pH 7. The bound proteins were eluted with equilibration buffer plus 250 mM imidazole and then the eluate passed through a size exclusion chromatography column (HiLoad Superdex 75 prep grade, GE Healthcare Inc.) equilibrated with 50 mM sodium phosphate buffer containing 150 mM sodium chloride, pH 7. Purified PPEpo fractions were pooled and stored at - 80 °C for further analyses.
N-terminal PEGylation of PPEpo
The reductive alkylation with 20-kDa mPEG-propionaldehyde (SunBio Inc.) was carried out with a PPEpo concentration of 1mg/ml and a 15-fold molar excess of PEG in 50 mM sodium phosphate, pH 5.0, with sodium cyanoborohydride added to a final concentration of 20 mM. The reaction was stirred at 4°C under nitrogen for 16 hours. The reaction mixture was stopped by diluting the mixture with 10 volumes of buffer A (20 mM Tris buffer, pH 8.0) and was loaded on a pre-packed HiTrap Q-Sepharose column (GE Healthcare Inc.) that was previously equilibrated with Buffer A. The column was washed with buffer A for removing unreacted PEGs and then, bound proteins were eluted with a linear NaCl gradient (0–500 mM) in Buffer A. The fraction containing mono-PEGylated PPEpo was then applied to a Superdex 200 10/30 column, pre-equilibrated with 50 mM sodium phosphate buffer containing 150 mM NaCl, pH 7.0. Pure mono-PEGylated conjugates were collected by elution of the column using the equilibration buffer, sterile filtered and stored at -80 °C.
The extent of protein modification and purity were analyzed by Size Exclusion HPLC employing a Shodex PROTEIN KW- 802.5 SEC column (Shodex, Japan) equilibrated with 50 mM sodium phosphate buffer containing 150 mM NaCl, pH 7.0.
In vitro bioassay
The in vitro biological activity of PPEpo and its PEGylated conjugate was determined by the UT-7 cells proliferation assay (CitationKomatsu et al., 1991). UT-7 cell lines (DSMZ, German Collection of Microorganisms and Cell Cultures, Germany) were maintained in αMEM medium containing 10% FBS, 40 mg/ml gentamycin, 2 mM glutamine and 5ng/ml GMCSF (Sigma-Aldrich). Prior to sample addition, the cells were rinsed twice with growth medium lacking GMCSF. Serial 2-fold dilutions of each sample were prepared in the media and a volume of 50 μl of each diluted sample was added in triplicate to appropriate wells of a 96 well microtiter plate containing 5000 cells/well. The plate incubated for 3 days at 37° C in a humidified 5% CO2 incubator. The positive control contained 200 ng/ml rHuEpo (R&D Systems Inc., USA) while the negative control contained no forms of Epo. Cell growth was evaluated on the basis of the colorimetric MTT assay of CitationMosmann (1983). In brief, 20 μl of sterilized 5 mg/ml MTT (Sigma-Aldrich) solution was added to each culture well. After 1-5 h incubation at 37°C, MTT solubilization solution was added to each well and the absorbance was read at 570 nm. For each test, a blank containing complete medium without cells was included to measure the background absorbance.
In vivo bioassay
The in vivo bioactivity of PPEpo, PEGylated PPEpo and rHuEpo were determined using the normocythaemic mice assay. Healthy B6D2F1 mice, weighing approximately 16-18 g, were obtained from Pasteur Institute of Iran. All animal experiments were performed with the approval of the ethical committee of Pasteur Institute of Iran. Groups of four animals were injected subcutaneously with 10 µg/kg of each sample. Blood samples were drawn by puncture of the tail vein over a period of 8 days. For each collected sample, 3 μl of whole blood was mixed thoroughly with 2 ml of thiazole orange (0.1 µg/ml) in Dulbecco’s Phosphate-Buffered Saline (Invitrogen) and incubated at room temperature for 30 minutes, protected from the light. After this time, the reticulocyte count was determined microfluorometrically in a Beckman Coulter flow cytometer and the percentage of reticulocyte count in red blood cells was quantified by analysis of the red fluorescence histogram according to supplier’s instructions.
Pharmacokinetic studies
Four groups each of 3 male New Zealand White rabbits (Pasteur Institute of Iran), weighing 1.3 to 1.5 kg, received a single I.V. bolus of PPEpo, PEGylated PPEpo or rHuEpo, each at a dose of 15µg/kg. Blood samples were withdrawn via marginal ear vein at 0, 5, 15 min, 1, 8, 24, 48 and 72 h post injection and serums were isolated and stored at -70°C. Appropriate dilutions of serum samples were analyzed in duplicate using human Epo ELISA kits (Roche Diagnostics GmbH, Germany). Standard curves ranged from 0 to 200 mIU/ml. The base line value of erythropoietin in rabbits was determined at the zero time (before the injection of samples) and this value (6 ± 1 mIU/ml) was subtracted from all the determined values. The serum concentration versus time profile was conducted up to 72 h.
Protein analysis
SDS-PAGE analysis was carried out by the standard Laemmli SDS-PAGE method (CitationLaemmli, 1970) in a 12.5% gel and developed using the Silver staining method. Concentration of purified Epo or PEG-PPEpo was measured by the Bradford method (CitationBradford, 1976). N-terminal amino acid sequencing was performed by Edman’s degradation method on the purified sample electroblotted onto PVDF membrane (Institut fur Chemeie, Universitat fur Bodenkultur Wien, Austria).
Statistical analysis
The data were statistically analyzed using Student’s t test and one-way multivariate ANOVA to calculate P value. P < 0.05 was considered statistically significant.
Results and Discussion
Secreted-expression of PPEpo
PPEpo was expressed as a secreted protein by P.pastoris and migrated on SDS-PAGE gel with an apparent mass of 33 kDa (, lane 2). In the same conditions, CHO-derived rHuEpo migrated with an apparent mass of about 38-39kDa (, lane 1). The higher apparent MW of CHO-derived rHuEpo may be caused by negative charged sialic acid residues which are absent in PPEpo. Amino acid sequencing of PPEpo indicated that the first 10 N-terminal residues were identical to the mature amino acid-deduced from cDNA sequence of rHuEpo (i.e.: APPRLICDSR) and pointed to complete removal of α-factor sequence in PPEpo.
Figure 2. Silver-stained SDS-PAGE analysis of Epo proteins and PEG-PPEpo. Lanes: (1) CHO-derived rHuEpo (R&D Systems); (2) PPEpo; (3) PEGylated PPEpo; (M) Protein size marker SM0431 (Fermentas). The higher apparent MW of CHO-derived rHuEpo (38–39kDa) compared to Pichia-derived rHuEpo (33 kDa) is the result of different glycosylation patterns between two hosts.
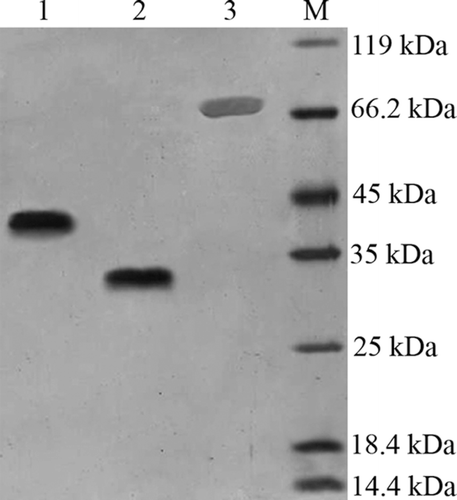
Under the defined conditions in Materials and Methods section, expression levels of 20–30 mg/l were obtained for PPEpo. The expression levels surely could be enhanced using exploring the high expressing clones (potentially containing more than one copy of the integrated vector) as well as improving the fermentation conditions such as pH/buffering conditions, methanol post-induction regime, temperature and induction mass (CitationLi et al., 2007). Moreover, by employing bioreactors, where many important parameters such as methanol, pH and oxygen levels can be tightly controlled, even higher yields of expression may be achieved (CitationCelik et al., 2009).
To facilitate purification of PPEpo by affinity chromatography, 6xHis-tag was added at the C-terminal of the protein. The final purity of PPEpo at the end of two purification steps was more than 98% as determined by SE-HPLC ().
N-terminally PEGylated PPEpo
PPEpo was PEGylated with 20-kDa mPEG-propionaldehyde to obtain mono-N-terminally-PEGylated PPEpo. Previous studies clearly indicated that the amino terminus of Epo is not important for the bioactivity (CitationBoissel et al., 1993; CitationElliott et al., 1997) and many attempts have been made for N-terminal PEGylation of rHuEpo (CitationBurg et al., 2006; CitationWei et al., 2000; CitationKapre et al., 2007). Among these creative approaches, reductive alkylation of PEG aldehydes to the N-terminus of proteins has a well-known chemistry that takes advantage of the lower pKa of the N-terminal α-amine compared to other amine groups in the protein under acidic condition (around pH 5) (CitationKinstler et al., 1996). As determined by SE-HPLC analysis (data not shown), under our defined conditions, a percentage of approximately 50% and 10% was obtained for mono-PEGylated- and di-PEGylated-PPEpo conjugates, respectively.
Purification and analysis of mono-PEGgylated PPEpo
The mono-PEG-PPEpo molecules, produced in the PEGylation reaction, were separated from di-PEG-PPEpo and/or the unreacted PPEpo molecules by anion-exchange chromatography. Considering the protein’s charge shielding effect of the PEG chain (CitationMcGoff et al., 1988) and also the pI of non-sialilated PPEpo, which expects to be less acidic compared to the pI of the sialilated CHO-derived rHuEpo, the pH value of the mobile phase was adjusted to 8.0, to ensure complete coupling of the PEG-PPEpo proteins with positive charged groups of the column.
Silver stained SDS-PAGE analysis of purified mono-PEG-PPEpo is shown in (, lane 3). The molecular weight of Pichia-expressed rHuEpo is approximately 30 kDa as determined by MALDI-TOF mass spectrometer analysis (CitationCelik et al., 2007). 20-kDa mono-PEG-PPEpo, with a MW of approximately 51 kDa, migrated with a highly increased apparent molecular mass of around 70 kDa. The increased hydrodynamic size of the hydrated PEG chains in PEG-PPEpo conjugates could possibly results in their decreased electrophoretic mobility of PEG-PPEpo that is also known for other proteins (CitationKurfurst, 1992; CitationKusterle et al., 2008), Moreover, the lower electrophoretic mobility of PEG-PPEpo may be partially ascribed by the entanglement and/or the charge masking effect of the PEG shell surrounding the protein (CitationKurfurst, 1992; CitationMcGoff et al., 1988). Size exclusion HPLC analysis showed a purity of more than 97% for purified mono-PEG-PPEpo ().
In vitro bioactivity
The induction of proliferative responses on UT-7 cells by cumulative concentrations of rHuEpo, PPEpo and mono-PEG-PPEpo were evaluated in separate assays. The bioassay results are summarized in and it shows that PPEpo had a higher in vitro activity compared to rHuEpo. This result in agreement with previous reports (CitationTakeuchi et al., 1990; CitationWasley et al., 1991) indicated that removing of sialic acid residues could increase the in vitro bioactivity of erythropoietin possibly as the result of increasing affinity to its receptor. Furthermore, the mono-PEG-PPEpo conjugate was biologically active and could keep supporting the proliferation of UT-7 cells as it is shown in . However, mono-PEGylation of PPEpo resulted in a small loss of bioactivity that may point to a slight modification of essential lysine residues apparently as the result of incomplete selectivity in reductive alkylation approach (CitationKinstler et al., 1999).
Table 1. The in vitro bioassay results
In vivo bioactivity
The in vivo efficacy was determined by counting the average reticulocytes in the normocythaemic mouse after s.c. injecting a single dose of Epo proteins. No in vivo bioactivity could be determined for PPEpo under the test conditions as it is shown in . However, our results indicated that the in vivo activity of Pichia-derived Epo could be significantly (P < 0.05) increased through PEGylation. Within 96 h, PEG-PPEpo could successfully increase the percentage of reticulocyte count in red blood cells (Ret %) to maximal levels and showed comparable in vivo activity to rHuEpo using the same dose (10 µg/kg). Afterward, the Ret % decreased slowly to the base line level on day 8.
Figure 4. In vivo activities of rHuEpo (R&D Systems), PPEpo and PEG-PPEpo in the normocythaemic mice. The percentage of reticulocyte count in red blood cells (Ret %) was determined microfluorometrically after subcutaneous administration of each sample at a dose of 10 μg/kg. Data are means ± SD for 4 mice per group.
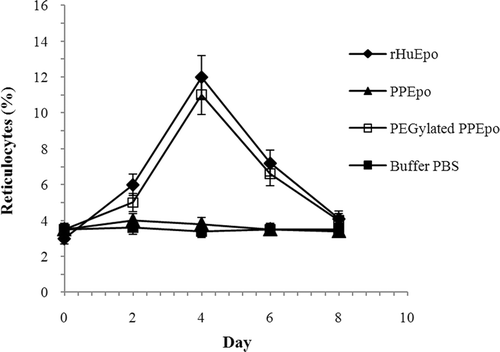
The markedly improved in vivo potency which observed for PEG-PPEpo conjugate could be ascribed to PEGylation-produced changes in PPEpo parent molecule. It is known that some of these physicochemical alterations particularly the increased hydrodynamic size and modified electrostatic binding properties could increase the in vivo residence time of PEG-Epo conjugates by a number of mechanisms such as decreases in the renal filtration, reducing the cellular protein clearance and increasing the resistance to proteolytic degradation (CitationBailon and Berthold, 1998; CitationHarris et al., 2001; CitationGross and Lodish, 2006) Moreover, it has been shown that the desialilated and/or non-sialilated Epo molecules (such as Pichia-expressed Epo) are rapidly eliminated by hepatic asialoglycoprotein receptors (Rs) (CitationSpivak and Hogans, 1989). Therefore, the restored in vivo activities of PEGylated PPEpo can be primarily attributed to the shielding effect and steric hindrance of polymeric chains surrounding the protein and preventing its interaction with the asialoglycoprotein receptors..
In agreement with our results, CitationWang et al. (2010) showed the superior in vivo efficacies for 40k-PEG-rh-ngEpo (40-kDa-PEG conjugate of non-glycosylated E.coli-derived rHuEpo) compared to rHuEpo. The authors indicated that the in vivo activity of PEGylated rh-ngEpo proteins was greatly dependent on size and structure of the attached PEG chains (CitationWang et al., 2010). Accordingly, using branched and larger PEG chains (i.e. 30− 40 kDa) may provide possibilities for even more increase in the in vivo activity of PEGylated Pichia-expressed Epo.
Pharmacokinetics studies
The mean serum concentration versus time profiles of rHuEpo, PPEpo and PEGylated PPEpo in rabbits are shown in . Following intravenous injection of samples, serum levels declined biphasically and therefore, a two compartmental model was found appropriate to describe the pharmacokinetics of Epo proteins and PEG-PPEpo conjugate in rabbits. The pharmacokinetic parameters are summarized in . The elimination rate constants (β) for samples were obtained from the terminal regression line from 24 - 72 hours post injection except for PPEpo that was measured from 1− 24 hours. The distribution rate constants (α) were calculated by the method of residual. The terminal half-life and mean residence time (MRT) of PEG-PPEpo were increased approximately 4-fold and 6.5-fold respectively, as compared with those of PPEpo. Moreover, systemic clearance for PEG-PPEpo was reduced 20-fold relative to the unmodified PPEpo. Multiple mechanisms and pathways are known for the clearance of rHuEpo including renal excretion (CitationDinkelaar et al., 1981), receptor-mediated (hematopoietic and/or non-hematopoietic Epo-Rs) cellular internalization and degradation (CitationGross and Lodish, 2006) and also being captured by the reticuloendothelial or lymphatic systems (CitationAgoram et al., 2008). The decreased clearance of PEGylated PPEpo is attributable to the increased overall size and reduced ligand-receptor binding affinity of the PEG-conjugate which may collectively lead to attenuated efficiency of Epo eliminating mechanisms.
Figure 5. Mean serum concentration vs time profiles of rHuEpo (R&D Systems), PPEpo and PEG-PPEpo following intravenous administration in normal rabbits. Each rabbit received a single 15 μg protein/kg dose. Protein levels were measured using human Epo ELISA kits. Data are means ± SD for 3 rabbits per group.
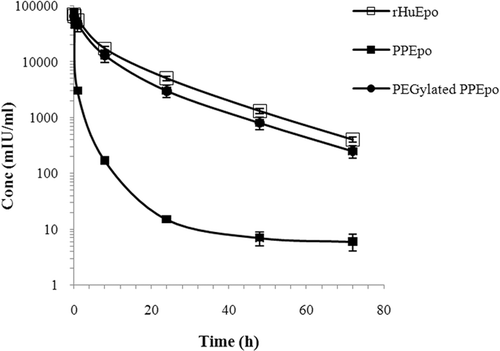
Table 2. A number of pharmacokinetic parameters of rHuEpo, PPEpo and PEGylated PPEpo following intravenous administration in rabbits
The clearance rate and terminal half-life of 20-kDa-PEG-PPEpo were comparable to those of rHuEpo (). In this context, CitationWang et al. (2010) showed similar results for 30k-PEG-rh-ngEpo. It is noteworthy that the carbohydrate portion of rHuEpo has been shown to play an important role in the physico-chemical and biological stability of the protein (CitationNarhi et al., 1991) and therefore, in contrast to non-glycosylated E.coli-derived rHuEpo, the sugar moieties of Pichia-expressed Epo could increase the favorable effects of PEG chain possibly through different mechanism(s).
Conclusions
Along with other emerged data, our results suggested that the function of PEGylation in increasing the serum residency and in vivo bioactivity of non-sialilated Pichia-derived Epo could be comparable to the essential role of native sialic acid residues for hematopoietic activity of rHuEpo. The results indicated that the linkage of a 20-kDa PEG chain to the N-terminus of PPEpo could significantly increase the in vivo bioactivity and improve most of the pharmacokinetic parameters of the protein toward the increasing of plasma residency whereas minimally reduces the in vitro bioactivity. Moreover, the present study demonstrated that the PEGylation of Pichia-expressed Epo could be considered as a promising strategy for generating cost-effective and long-acting erythropoietic agents with the desired physico-chemical and biological properties.
Declaration of interest
The authors report no declarations of interest.
References
- Agoram B, Aoki K, Doshi S, Gegg C, Jang G, Molineux G, Narhi L, Elliott S. (2008). Investigation of the effects of altered receptor binding activity on the clearance of erythropoiesis-stimulating proteins: nonerythropoietin receptor-mediated pathways may play a major role. J Pharm Sci, 98, 2198–2211.
- Bailon P, Berthold W. (1998). Polyethylene glycol-conjugated pharmaceutical proteins. Pharm Sci Technol Today, 1, 352–356.
- Boissel JP, Lee WR, Presnell SR, Cohen FE, Bunn HF. (1993). Erythropoietin structure-function relationships. Mutant proteins that test a model of tertiary structure. J Biol Chem, 268, 15983–93.
- Bradford MM. (1976). A rapid and sensitive for the quantitation of microgram quantities of protein utilizing the principle of protein dye binding. Anal Biochem, 72, 248–254.
- Burg J, Engel A, Franze R, et al. (2006). Erythropoietin Conjugates. US Patent, No, 7128913.
- Celik E, Calik P, Halloran SM, Oliver SG. (2007). Production of recombinant human erythropoietin from Pichia pastoris; and its structural analysis. J Appl Microbiol, 103, 2084–2094.
- Celik E, Calik P, Oliver SG (2009). Fed-batch methanol feeding strategy for recombinant protein production by Pichia pastoris in the presence of co-substrate sorbitol. Yeast, 26, 473–484.
- Daly R, Hearn MTW. (2005). Expression of heterologous proteins in Pichia pastoris: a useful experimental tool in protein engineering and production. J Mol Recognit, 18, 119–138.
- Dinkelaar RB, Engels EY, Hart AA, Schoemaker LP, Bosch E, Chamuleau RA. (1981). Metabolic studies on erythropoietin (EP): II. The role of liver and kidney in the metabolism of Ep. Exp Hematol, 9, 796–803.
- Egrie JC, Dwyer E, Browne JK, Hitz A, Lykos MA. (2003). Darbepoetin alpha has a longer circulating half-life and greater in vivo potency than recombinant human erythropoietin. Exp Hematol, 31, 290–299.
- Elliott S, Lorenzini T, Chang D, Barzilay J, Delorme E. (1997). Mapping of the active site of recombinant human erythropoietin. Blood, 8(9), 493–502.
- Elliott S, Pham E, Macdougall IC. (2008). Erythropoietins: a common mechanism of action. Exp Hematol, 36, 1573–1584.
- Gross AW, Lodish HF. (2006). Cellular trafficking and degradation of erythropoietin and novel erythropoiesis stimulating protein (NESP). J Biol Chem, 281, 2024–2032.
- Hamidi M, Azadi A, Rafiei P. (2006). Pharmacokinetic consequences of pegylation. Drug Deliv, 13(6), 399–409.
- Harris JM, Martin NE, Modi M. (2001). Pegylation: a novel process for modifying pharmacokinetics. Clin Pharmacokinet, 40, 539–551.
- Horton RM (1993). In Vitro Recombination and mutagnesis of DNA: SOIng together tailor-made genes. In: White, BA. (Eds.), Methods in Molecular Biology, Vol. 15: PCR Protocols: Current Methods and Applications. 251–266.Totawa: Humana Press.
- Jevsevar S, Kunstelj M, Porekar VG. (2010). PEGylation of therapeutic proteins. Biotechnol J, 5, 113–128.
- Kapre V, Shaligram S, Nho K, Bae G. (2007). N-Terminal PEG-Conjugate of Erythropoietin. IPP WO/2007/010552.
- Kinstler OB, Brems DN, Lauren SL, Paige AG, Hamburger JB, Treuheit MJ. (1996). Characterization and stability of N-terminally PEGylated rhG-CSF. Pharmaceut Res, 13, 996–1002.
- Kinstler OB, Gabriel NE, Farrar CE, DePrince RB. (1999). N-terminally chemically modified protein compositions and method. US Patent, No, 5985265.
- Komatsu N, Nakauchi H, Miwa A, Ishihara T, et al. (1991). Establishment and Characterization of a Human Leukemic Cell Line with Megakaryocytic Features: Dependency on Granulocyte-Macrophage Colony-stimulating Factor, Interleukin 3, or Erythropoietin for Growth and Survival. Cancer Res, 51, 341–348.
- Kurfurst MM. (1992). Detection and molecular-weight determination of polyethylene glycol-modified hirudin by staining after sodium dodecyl-sulfate polyacrylamide-gel electrophoresis. Anal Biochem, 200, 244–248.
- Kusterle M, Jevsevar S, Porekar VG. (2008). Size of Pegylated Protein Conjugates Studied by Various Methods. Acta Chim Slov, 55, 594–601.
- Laemmli UK (1970). Cleavage of structural proteins during assembly of head of bacteriophage-T4. Nature, 227, 680–685.
- Leist M, Ghezzi P, Grasso G, et al. (2004). Derivatives of erythropoietin that are tissue protective but not erythropoietic. Science, 305, 239–242.
- Li P, Anumanthan A, Gao XG, Ilangovan K, Suzara VV, et al. (2007). Expression of recombinant proteins in Pichia pastoris. Appl Biochem Biotechnol, 142(2), 105–24.
- Long DL, Doherty DH, Eisenberg SP, et al. (2006). Design of homogeneous, monopegylated erythropoietin analogs with preserved in vitro bioactivity. Exp Hematol, 34, 697–704.
- Macdougall IC, Roberts DE, Coles GA, Williams JD. (1991). Clinical pharmacokinetics of Epoetin (recombinant human erythropoietin). Clin Pharmacokinet, 20, 99–113.
- Markham A, Bryson HM. (1995). Epoetin alpha. A review of its pharmacodynamic and pharmacokinetic properties and therapeutic use in nonrenal applications. Drugs, 49, 232–254.
- McGoff P, Baziotis AC, Maskiewicz R. (1988). Analysis of polyethylene glycol modified superoxide dismutase by chromatographic, electrophoretic, light scattering, chemical and enzymatic methods. Chem Pharm Bull, 36(8), 3079–3091.
- Mosmann T. (1983). Rapid colorimetric assay for cellular growth and survival: application to proliferation and cytotoxicity assays. J mmunol Methods, 65, 55–63.
- Narhi LO, Arakawa T, Aoki KH, Elmore R, Rohde MF, Boone T, Strickland TW. (1991). The effect of carbohydrate on the structure and stability of erythropoietin. J Biol Chem, 266, 23022–6.
- Spivak JL, Hogans BB (1989). The in vivo metabolism of recombinant human erythropoietin in the rat. Blood, 73, 90–99.
- Takeuchi M, Takasaki S, Shimada M, Robata A. (1990). Role of sugar chains in the in vitro biological activity of human erythropoietin produced in recombinant Chinese Hamster Ovary Cells. J Biol Chem, 265, 12127–30.
- Wang Y-J, Hao S-J, Liu Y-D, Hu T, et al. (2010). PEGylation markedly enhances the in vivo potency of recombinant human non-glycosylated erythropoietin: A comparison with glycosylated erythropoietin. J Control Release, 145, 306–313.
- Wasley LC, Timoney G, Murtha P, et al. (1991).The importance of N- and O-linked oligosaccharides for the biosynthesis and in vitro and in vivo biologic activities of erythropoietin.Blood, 77, 2624–2632.
- Wei Z, Menon-Rudolph S, Ghosh-Dastidar P. (2000). Methods and kits for making polypeptides having a single covalently bound N-terminal water soluble polymer. US Patent, No, 6077939.
- Wojchowski DM, Caslake L. (1989). Biotinylated recombinant human erythropoietins: bioactivity and utility as receptor ligand. Blood, 74, 952–958.
- Yesland KD. (2008). Pharmacodynamically enhanced therapeutics proteins. US Patent, gt;No, 2008/0171696.