Abstract
A novel three-dimension micro-device was formulated to control delivery of 5-fluorouracil (5-FU) for the treatment of solid tumors. The poly-(lactic-co-glycolic) acid (PLGA), which is both biocompatible and biodegradable, was used as carrier material. The characteristics of drug release in vitro and in vivo and the performance of the micro-device after implantation in tumor bearing mice were evaluated. A constant release profile from in vitro test was obtained for a period of 7 days, and it correlated well with the in vivo release profile. In the distribution experiment of 5-FU micro-device, it was demonstrated that 5-FU remained in the tumor tissues for more than 7 days after implantation. Likewise, we found that the 5-FU concentration in tumor correlated well with the in vivo release. Tumors treated with 5-FU loaded micro-device of three different dosages showed significant tumor reduction (P < 0.05) compared with empty control micro-device 7 days after administration. Moreover, the implantation treatment showed enhanced efficacy compared with the intraperitoneal administration with the same dosage. These results suggested that the three-dimensional micro-device may provide a promising local and controlled release drug delivery system, which may enable delivery of multiple drugs for post-surgical chemotherapy against solid tumor.
1 Introduction
Despite of the clinicians’ and pharmacists’ efforts, cancer is still the most life-threatening disease. Surgical resection is the mainstay of curative treatment for solid tumor, but it can be performed on a small subgroup of patients (Ozer et al., Citation2009). Chemotherapy became the most common approach for cancer treatment. However cancer chemotherapy, the use of cytotoxic drugs, is not always effective. Generally, cytotoxic drugs are highly toxic but poorly specific, and do not differentiate between normal and cancer cells (Corrie, Citation2008). Therefore, conventional chemotherapy administration or systemic administration is unable to target a specific area of the body. To reach an acceptable therapeutic level at the desired site, high dosages or repeated injections are often required, which can lead to toxic side effects at the non-target organs and inconvenience. In addition, most of the conventional administration may cause drug levels in the body to rise rapidly and/or decline sharply, leading to toxic side effects at the peaks and inadequate therapy at the troughs. Due to these obstacles, controlled and targeting or localized release approches is desired.
In this regard, implantable drug delivery systems based on biodegradable polymer have received tremendous attention (Langer, Citation1991). Regional chemotherapy via localized administration can deliver drug to the tumor, which is expected to enhance the efficacy of antineoplastic agents and decrease the incidences of their side effects commonly observed with systemic therapies (Mishra, Citation2009). Controlled drug delivery systems have achieved long-term maintenance of therapeutic drug levels and received increased medical and public acceptance. Biodegradable polymers have been the most attractive merit, because they do not require removal after the drug has been released (Wei et al., Citation2009). As described previously, the biodegradable fiber implants have been developed for the favorable release profile. The filament like suture morphology allowed for easy implantation with a surgical needle and removal surgery was unnecessary due to the controlled biodegradability (Ranganath and Wang, Citation2008; Mack et al., Citation2009). A novel mixed micelle system, composed of poly(ethylene oxide)/poly(propylene oxide) (Pluronics) block copolymers, was constructed to treat gynecological solid cancers through localized administration (Yang et al Citation2009). And local drug delivery through mill-rod implants has shown efficacious anti-tumor response (Qian, Citation2003). Considerable research has been conducted on drug release in vitro (Kim et al., Citation2005; Alexis, Citation2005; Jones et al., Citation2009; Kasperczyk et al., Citation2009) and drug transport in vivo (Rabin et al., Citation2008; Santoveña et al., Citation2010).
Biodegradable polymers are widely researched and used in implantable drug delivery system (Xiaosong and Roland, Citation2006; Martin and Roland, Citation2008; Kumari et al., Citation2010). Amongst them, the thermoplastic aliphatic poly(esters) like polylactic acid (PLA) (Nampoothiri et al., Citation2010), poly(glycolide) (PGA) (Wang, Citation2004), and especially the copolymer of lactide and glycolide, poly(lactide-co-glycolide) (PLGA) (Yadav et al., Citation2010; Kim et al., Citation2005; Klose et al., Citation2010) have attracted most attention due to their biodegradability, biocompatibility, and physical properties which enable them to be prepared and shaped easily. Most of the polymeric implants were prepared in the forms of micro/nano-particles, milli/micro discs, wafers or rod by a variety of methods, such as microencapsulation (Kim et al., Citation2005;Nagarwal et al., Citation2009), double (multiple) emulsion (Balmayor et al., Citation2009) and compression (Dorta et al., Citation2002). Recent advances in microelectromechanical systems (MEMS) technology have provided a unique opportunity to fabricate miniature biomedical devices for implantable drug delivery (Grayson et al., 2008; Yang et al., Citation2005). Here we used biodegradable polymers, PLGA, and developed an implantable device with micro-honeycomb structures by MEMS technology (). This device can control drug delivery for long periods of time, provide targeting performance by on-spot implantation, and realize simultaneous delivery of multiple drugs due to large array of micro-chambers which is the most exceptional advantage.
Figure 1. PLGA micro-device with micro-honeycomb structures containing 5-FU with the size of the micro-chambers on the right side.
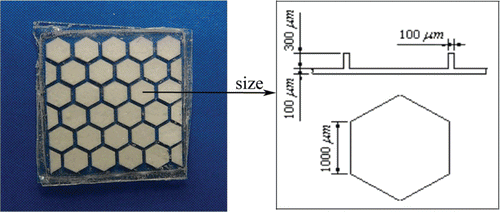
The 5-fluorouracil (5-fluoro-2,4-pyrimidinedione) (5-FU) is an antimetabolite of the pyrimidine analog class which is widely used in the treatment of gastrointestinal, breast, and head and neck malignancies alone or in combination chemotherapy regimens (Poorter et al., Citation1995; Berruti et al., Citation2005). The conventional method of delivering 5-FU is mainly through intravenous perfusion or oral administration (Shukla et al., Citation1977). However, these forms of therapy may lead to severe side effects, including haematological, mucosal and gastrointestinal toxicity due to the toxicity of the drug (Atilla et al., Citation2009). Furthermore, when given intravenously, 5-FU has a plasma half-life of only 8–20 min and a rapid distribution and elimination (Zinutti et al., Citation1998), which further limits its efficacy after systemic application. An alternative approach to overcome these disadvantages is the localized and controlled delivery of 5-FU using biodegradable polymeric device implanted in the tumor bed.
In the present study, we selected 5-FU as model drug, then fabricated and evaluated PLGA micro-device with micro-honeycomb structure. The release of 5-FU from the implanted micro-device in vitro/in vivo and its distribution in the tumor were detected. The efficacy and the safety of the micro-device were also evaluated in vivo by peritumoral implantation in tumor bearing mice.
2 Materials and Methods
2.1 Materials
Poly(lactic-co-glycolic acid) (PLGA) (50:50) with an inherent viscosity of 0.2 dL/g (molecular weight, Mw is 17,000) was supplied by Daigang Biological Technology Co., Ltd.. (Shandong, China). 5-fluorouracil was purchased from Nantong Pharmaceutical Company (Jiangsu, China). 5-Bromouracil (in internal standard with content of 99.7%) was donated by Rudong County Fengli Medical Chemical Plant (Jiangsu, China). HPLC grade methanol solvents were from Fisher (Hampton, NH, USA). Ammonium sulfate was purchased from Tianjing Yaohua Chemical Reagent Company (Tianjing, China). All other analytical grade chemicals were obtained from Xi’an Chemical Regent Factory (Shannxi, China).
2.2 Preparation of micro-device
The polymer micro-device was fabricated as previously reported (Yang et al., Citation2005). Briefly, an optical mask was used to lithographically pattern the SU-8 insert-mold. A thin layer of PDMS (polydimethylsiloxane) was coated on the SU-8 micro patterns to obtain the final mold. The PDMS mold was mechanically peeled off and then used to fabricate the PLGA structures. PLGA with a molar ratio of 50:50 (MW 17,000) was dissolved in acetone at 15% (w/v) concentration to form a clear solution. Then, the PLGA solution was poured on the PDMS molds. The molds covered with PLGA solution was baked by a hot-plate for 3-4 h at 60 °C, and then cooled down. Subsequently, the PLGA microstructures are peeled from the PDMS model. The 5-FU was ground as to make the particle size small to fasten dissolution in the release process and fine powders of 5-FU was filled into the micro-chamber of the PLGA structure using an injector. Finally, the PLGA micro-device was successfully fabricated when the membrane composed of the same PLGA was sealed on the PLGA microstructure. The final micro-device dimensions are approximately 10 × 10 mm in length×width and 1 mm in thickness ().
2.3 In vivo implantation
Healthy male ICR mice (weighing 18-20 g) were purchased form Laboratory Animals Center of Xi’an Jiaotong University. Animals were maintained in a clean room at the temperature of 25 ± 1°C and a relative humidity of 50%. Mice were housed in cages with free access to water and standard rat chow. After a 3-day period of acclimation, they were used for experiments. The protocol of animal study was approved by the Institutional Animal Care and Use Committee of Xi’an Jiaotong University.
Murine sarcoma S180 cells were obtained from the Laboratory Animals Center of the Fourth Military Medical University (Xi’an, China) and reproduced in our laboratory. Solid tumors in the mice were produced by subcutaneously injection of 5 × 105 viable murine sarcoma S180 cells on the left flank of the mice. Three days after inoculation, the mice were randomly divided into 6 groups and received the following treatments: 1) control with no treatment (n = 31), 2) PLGA micro-device without 5-FU (n = 6), 3) PLGA micro-device containing 4.3 mg 5-FU (n = 6), 4) PLGA micro-device containing 7.2 mg 5-FU (n = 31), 5) PLGA micro-device containing 10.7 mg 5-FU (n = 6), 6) intraperitoneal administration of 0.4 mL of 5-FU injection with the concentration of 6 mg/mL on day 1, 3 and 5 respectively (n = 6). Equal amount of 5-FU was administered into animals of 5-FU injection group and 7.2 mg 5-FU loaded PLGA micro-device group for sensible comparison of the treatments.
On the day of implantation (three days after inoculation), PLGA micro-device containing 5-FU and drug-free PLGA micro-device were sterilized under UV light using the medical purifying worktables (YJ-875, Suzhou HuaYuan Purifying and Filtering Equipment CO.,LTD) for 3 h prior to implantation. Animals were anesthetized with diethyl ether and a single incision 1 cm long was made on their backs near the tumor. Sterilized drug-free or 5-FU-containing PLGA micro-device was inserted into the cavity beneath the skin and the incision was sutured.
2.4 In vitro/in vivo release
To characterize the in vitro release kinetics, the PLGA micro-device loaded with 7.2 mg 5-FU was placed in a conical flask with 50 mL of normal saline. The sample flask was incubated at 37°C in a gas bath constant-temperature oscillator (THZ-82, Jiangsu Jintan zhengji Instruments CO., LTD) with a speed of 40 ± 10 rpm. At predetermined time points, the release medium was exchanged completely. The concentration of released 5-FU was determined by the HPLC method. The percentage of cumulative release was obtained by normalizing the released 5-FU to the amount that was loaded in the PLGA micro-device (n = 5).
To evaluate the in vivo drug release, five tumor bearing mice of the group of PLGA micro-device containing 7.2 mg 5-FU were sacrificed at each of the following time intervals: 2, 3, 4, 5, 6 and 7 days after drug administration. The micro-device were removed from the implantation site and separated from the tissue. Each micro-device was placed in 0.5 mL chloroform, which led to complete dissolution of the PLGA polymer. Then the solution was diluted 100 times with distilled water and centrifuged to remove the precipitated PLGA polymer. The supernatant was collected and analyzed according to the HPLC method. The released 5-FU amount in vivo was calculated by subtraction of the drug content in the degraded micro-device from the total drug loaded into the micro-device.
The HPLC system consisted of an LC10ATvp pump and a SPD10Avp detector (Shimadzu, Japan). Samples were analyzed on a C18 Diamonsil ODS column (150mm × 4.6mm i.d., 5μm). The eluting solvent consisted of methanol and water (5:95, v/v). The detection wavelength was set at 270 nm and the flow rate was 0.7 mL/min. The volume injected was 10μL.
2.5 Anti-cancer efficacy and toxicity in vivo
Besides five tumor bearing mice from the group of PLGA micro-device containing 7.2 mg 5-FU were sacrificed as described above, five mice from the control with no treatment group were also sacrificed on day 2, 3, 4, 5 and 6 after drug administration. And on day 7 post administration, all the remaining mice were sacrificed. The tumors of the sacrificed mice were all excised and weighed. The inhibition rate (IR) of tumor growth was calculated by the following formula: IR (%) = [(A-B)/A] ×100, where A is the average tumor weight of the no treatment group, and B is that of the other groups with treatment.
All the mice were weighed and observed every day for signs of distress (e.g. weight loss, color, lethargy) during the experimental period. And the liver, spleen, and kidney were taken out and weighed after the mice were sacrificed, and the relative weights (g/100 g B.W.) were calculated.
2.6 Determination of residual 5-FU in tumor masses
The tumor bearing mice from the 5-FU-loaded PLGA micro-device groups (4.3mg, 7.2mg, 10.7mg) and the intraperitoneal injection group were used to perform the residual 5-FU determination in vivo. After the tumor from these groups were exercised and weighted, they were wiped with filter paper, and then frozen at -20°C for 24 h prior to lyophilization. 10 mg of lyophilized tissue powders were mixed with 0.2 mL phosphate buffer (pH6.0) as disperse medium and 20 μL of a 5-Bromouracil (8.0 mg/mL) solution as internal standard. The residual 5-FU was extracted with a mixture organic solvent of isopropanol-ethyl acetate (15:85). The content of 5-FU was determined by the same HPLC–UV system under the same analysis condition as above, except that the analytic column was a Ecosil C18 column (250 mm × 4.6 mm i.d., 5 μm), protected by a guard column (15 mm × 4.6 mm i.d.).
2.7 Statistical analysis
All data are expressed as mean ± standard deviation. Significances were analyzed by one-way analysis of variance (AVONA) and P < 0.05 was considered statistically significant. All statistical analyses were performed using commercially available statistical software.
3 Results
3.1 In vitro and in vivo release
The developed HPLC method for determination of 5-FU content was validated by sensitivity, linearity, precision and accuracy. No significant interference was observed in chromatogram. The intra- and inter-day precision of 5-FU at three different concentration levels were acceptable with RSD values below 0.8% and 1.9%. The accuracy ranged from 99.1% to 101.0%. Good linearity was found over the 0.05~10.0 µg/ml concentration range.
The cumulative release of 5-FU from the PLGA micro-device in vitro was measured for 21 days. The profile showed that the release had three distinct phases, which included an initial burst release in the first day, followed by a second release phase and then a plateau (). Approximately 30% of the drug from PLGA micro-device containing 7.2 mg 5-FU was released during the initial first day. The burst release was followed by an approximate zero-order release the next 7 days. And then a much slower release continued up to 21 days, at which time the cumulative release of the drug was only 86.6 ± 5.6 %. However, a zero-order release equation with an excellent correlation (r = 0.9824) was obtained from the release profile from day 1 to day 7 ( inset), which indicated that the in vitro release of 5-FU from the PLGA micro-device was a constant release.
Figure 2. The cumulative release-time curve of 5-FU from the PLGA micro-device. (A)In vitro release of 5-FU from PLGA micro-device in 50mL of normal saline at 37°C. Error bars are expressed as standard error (n = 3). Inset: Correlation (r = 0.9928) in vitro release of 5-FU from day 1 to day 7. (B) Linear correlation of the in vitro cumulative release-time and in vivo cumulative release-time.
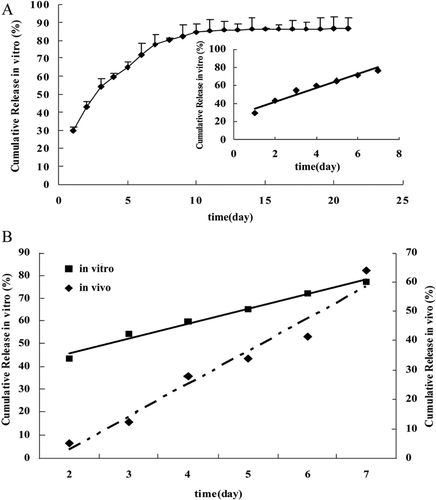
The release profile of the 5-FU in vivo was also assessed on tumor bearing mice. The in vivo release profile showed an initial release of 5.2% 2 days after administration, and a final cumulative release of 64.1% at the last day of the in vivo experiment. Similarly, the micro-device provided a zero-order release in vivo over the one week study. Different from in vitro release, the initial release of 5-FU in vivo was less than 6% on day 1 after implantation. As shown in , the linear relationship of the in vitro cumulative release-time and in vivo cumulative release-time was obtained where the time scaling parameters (slope) were 7.5248 and 11.083 and the time shifting parameters (intercept) were 27.331 and 19.091, respectively. It was recommended that the in vitro and in vivo release data was used to establish a relationship. Consequently, a relationship between in vitro and in vivo release data was established by plotting the in vitro release data on the x-axis and the in vivo release data on the y-axis on the same scale (from day 2 to day 7). An excellent correlation was established (r = 0.9620).
3.2 In vivo tumor inhibition
A study was performed to evaluate the tumoricidal activity of 5-FU in S180 sarcoma bearing mice after surgical implantation. showed the changes of average tumor weight as a function of time in the control with no treatment group and the 7.2 mg 5-FU micro-device group. It was observed that the tumor grew unabatedly (more than 2.0 g) till 10 days after inoculation for the control group with no treatment. For 5-FU micro-device group, the tumor weight increased gradually to achieve the summit on 6 days after administration. And then it began to decline. Comparing the tumor weights 2 and 3 days after implantation, there was no significant difference between the 5-FU micro-device group and the no treatment group (P > 0.05). On the day 3 after administration, the 5-FU micro-device produced a significant inhibition effect against the tumor when compared with the no treatment group. The tumor weight of 5-FU micro-device group compared to the nontreatment group was 0.35g smaller on day 4, 0.59g smaller on day 5, 0.97 g smaller on day 7, respectively. The tumor weight inhibition results positively show that the PLGA micro-device was able to provide sustainable 5-FU release in vivo. The representative photograph of the excised tumors from the S180 sarcoma bearing mice after administration is presented in . The similar results as in were obtained.
Figure 3. Inhibitory effects of 5-FU from the PLGA micro-device at a dose of 7.2mg. (A)The 5-FU micro-device showed tumor reduction compared with no treatment (P < 0.05) on day 4, 5, 6 and 7 after implant administration. And the tumor weights were not statistically different between 5-FU micro-device group and control group on day 2 and 3 after administration (P > 0.05). All data are expressed as mean ± S.D. (n = 5). (B) Representative photographs of S180 cell xenografts tumor in bearing tumor mice of control group and micro-device group loaded 7.2 mg 5-FU on day 2, 5 and 7 after administration.
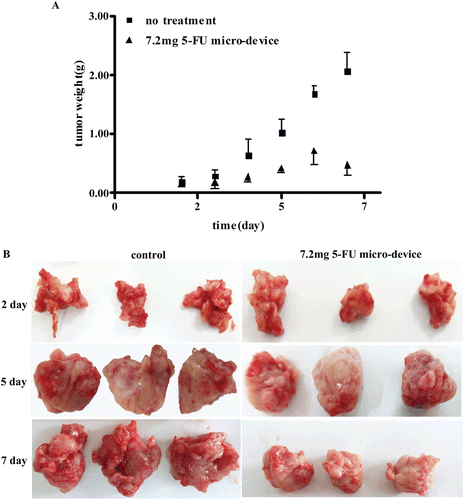
The inhibition rate of three different doses of 5-FU from the micro-device was also investigated in the tumor bearing mice. A side-by-side comparison of 7.2 mg 5-FU micro-device against the injection formulation with the same amount of drug was conducted. The results of the tumor weight for the different treatment groups are shown in . No treatment group and empty micro-device group were used for negative control to account for possible effects on the tumor growth. Overall, the 5-FU treatment groups showed lower tumor weight than the negative control groups. The low, middle and high dose micro-device groups showed significant difference in tumor weight compared to the negative control groups(P < 0.05), which indicated marked inhibition of tumor growth (). The IR in low and middle dose groups was 58.41% and 71.02%, respectively. The mean tumor weight of high dose micro-device group were 73.48% smaller than that of no treatment group, and it is also found that there was significant difference between the high dose micro-device group and the low dose group (P < 0.05).
Table 1. The 5-FU concentration in tumor, IR and relative tissue weight of mice bearing S180 xenograft after administration for 7 days. All data were expressed as mean ± S.D. (n = 6).
Figure 4. Dose response to 5-FU from the PLGA micro-device on S180 cell xenografts growth in bearing tumor mice. a: P < 0.05 compared with no treatment group, b: P < 0.05 compared with empty micro-device group, c: P < 0.05 compared with 7.2mg 5-FU injection group, d: P < 0.05 compared with 4.3mg 5-FU micro-device group. All data are expressed as mean ±S.D. (n = 6)
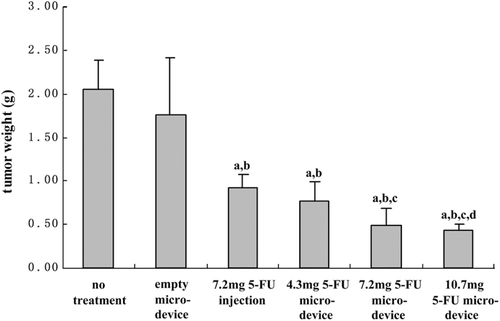
The drug administration was performed in two different ways, intraperitoneal administration and implantation administration. Both of the formulations produced significant inhibitory effects against the tumors when compared with no treatment group (P < 0.05). The IR shown in was 51.58% followed by the injection administration. In comparison, the inhibitory effect of 5-FU micro-device at the same dose was significantly different (P < 0.05). Moreover, the high dose micro-device showed significant inhibition effects on tumor growth as compared to the 5-FU injection group (P < 0.05). Although the 5-FU injection group could inhibit the tumor significantly, it could not extend the effect for long because 5-FU had a low terminal half life of less than 20 min in plasma. Once the drug cleared off, the tumor growth was beyond that of the control group. Therefore, the repeat administration was required. In the present experiment, and the treatment program of intraperitoneal administration of 5-FU injection was implemented on day 1, day 3 and day 5.
3.3 Toxicity in vivo
Toxicity of 5-FU formulations was evaluated by measuring the changes in body weight as a function of time as shown in . The body weights of the mice in no treatment, empty micro-device, 5-FU injection and 7.2mg 5-FU micro-device groups were monitored every day throughout the experiment. At the beginning of administration, the body weights showed no significant difference among 4 groups (P > 0.05). During the period of observation, there was no mortality in any group whereas 5-FU injection caused the mice hair to matt and locomotor activity reduction. The body weights of mice in every group increased gradually during the experiment except that body weights of the mice in 5-FU injection group was slightly reduced on day 4 and day 5 after administration. At the end of the experiment, the increase of body weight of 5-FU injection group was the lowest, and there were significant differences between the 5-FU injection group and no treatment group in the day 4~7 period ( P <0.05). The body weight of 5-FU micro-device group exhibited a relatively slow increase. However, no significant differences were observed between treated and untreated groups (P > 0.05).
Figure 5. Body weight changes of mice bearing S180 xenograft for one week. *P < 0.05 compared with no treatment group. All data are expressed as mean ± S.D. (n = 6)
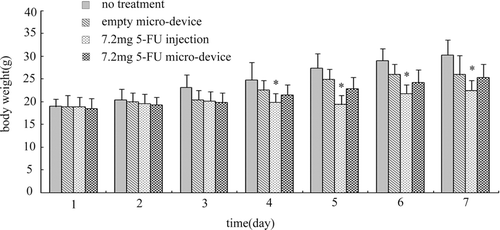
The relative weights of the organs, liver, spleen and kidneys of no treatment, empty micro-device, 5-FU injection, low, middle and high dose 5-FU micro-device groups are depicted in . It was noted that there was an appreciable loss in the spleen weights of mice after treatment with 5-FU injection (P < 0.05 compared with no treatment group). The results for 5-FU micro-device groups didn’t show any loss in the body weight and the relative weights of the organs. In other words, there was no significant toxicity in 5-FU micro-device groups.
3.4 5-FU tumor distribution
The drug concentrations in tumor of 7.2 mg 5-FU micro-device group at different time intervals are shown in . The PLGA micro-device loaded with 5-FU was implanted around tumor. Therefore, the drug was expected to be directly delivered to the tumor. The 5-FU concentration in the tumor was 1.32 ± 0.19 µg/g on day 2 after administration, and then the drug amount in tumor tissue gradually increased and reached 4.14 ± 0.89 µg/g on day 7 after administration. Accordingly, the inhibition rate increased gradually. The IR was 10.03% on day 2 after administration and increased to 71.02% on day 7 after administration. To a certain extent, the pharmacokinetic behavior of 5-FU in tumor tissues explained the inhibition effect of the micro-device loaded with 5-FU. In addition, the concentrations of 5-FU in the tumor for micro-device containing 4.3mg, 7.2mg and 10.7mg were also determined on day 7 after administration. Average 5-FU concentrations in tumor for the three micro-device groups were 1.56, 4.14 and 5.14µg/g respectively. 5-FU concentration gradually increased with the 5-FU dose in micro-device as is shown by the IR data ().
Table 2. The 5-FU concentration in tumor tissue and IR of mice bearing S180 xenograft after administration 7.2mg 5-FU micro-device. Data were expressed as mean ± S.D. (n = 5 or 6)
4. Discussions
Previously, much research about the in vitro activity of chemotherapeutic polymer micro-spheres has been reported (Xie et al., Citation2006; Moreno et al., Citation2009), but few in vivo results of the polymer micro-device has been demonstrated. This study fabricated a PLGA micro-device for the delivery of 5-FU against a tumor model, which showed significant inhibition of tumor growth. Tumors treated with micro-device of different doses showed marked diminished growth compared to empty control micro-device (P < 0.05). A dose-dependent behavior was also observed. The inhibiting effect of micro-device on the tumor growth improved with the increase dosage of 5-FU.
In vitro results indicated that the release of 5-FU from the micro-device started on the 1st day and continued for 3 weeks. When PLGA micro-device was immersed in the release medium, water was absorbed by the matrix, resulting in the matrix swelling and the formation of pores. Thus, the diffusion of the drug from matrix to aqueous medium was facilitated. Lemaire et al proposed the release mode for biodegradable matrix, whose mechanism was governed by diffusion and erosion (Lemaire et al., Citation2003). The drug release rate was greatly dependent on erosion rate of the polymeric matrix and the diffusion coefficient of the drug in the swollen matrix. So we could gain an understanding of constant release of 5-FU from the PLGA micro-device in vitro, when the polymer composition and surrounding environment were relatively fixed. The results of in vitro release indicated the release of 5-FU was an approximate zero-order process from day 1 to day 7. However, the initial burst release may be caused by the drug adhered on the surface of the micro-device. After 10 days, the swollen phenomenon of PLGA micro-device was observed, and then the release rate of the drug from the micro-device decreased. The cumulative release of 5-FU increased from 82.3% on day 10 to 86.6% on day 21.
To confirm in vitro release results, the release profile of the drug was assessed in vivo in bearing-tumor mice. The content of 5-FU in degraded micro-device showed 5-FU was released on day 2 after implantation in vivo, as was expected from in vitro data. More than half of initial loading was released on day 7 after implantation. However, the cumulative release in vivo was slower than the cumulative release in vitro, which might be explained by the swell characteristics of PLGA micro-device. In the case of a biodegradable and expandable micro-device system, the effect of swelling on the diffusivity of the drug was an important parameter, especially in early degradation. In this experiment, the micro-device is surrounded by 50 ml release medium in vitro, while the body fluid in vivo around the place where the device was implanted is little. So the absorption of water in vivo is slower than that in vitro. Therefore, the swelling rate of the matrix was slower in vivo, which had a significant influence on drug release in the early period after the micro-device was implanted in mice.
The drug release was also indicated by the distribution of 5-FU in tumor tissues. 5-FU was detected in the tumor on day 2 after implantation and reached the highest content on day 7, which indicated a constant in vivo release of 5-FU from the micro-device. Also the concentration of 5-FU in tumor correlated well with the cumulative release in vivo as shown in . Interestingly, we observed that the results of inhibition effect were in good agreement with the 5-FU concentration in tumor tissues at different time intervals (). Accumulation of drug in tumor tissue enhanced the inhibition effect of 5-FU micro-device after implantation around tumor. And there was no significant difference between the average body weight and the relative organs weight of 5-FU micro-device group and those of no treatment group, which indicated no significant toxicity of 5-FU micro-device.
Figure 6. Correlation analysis between the cumulative release of 5-FU in vivo and the 5-FU concentration in tumor.
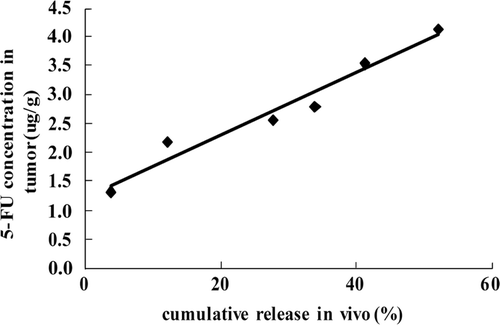
Although 5-FU is a potent anticancer agent for malignant tumor, its side effects are extensive and can not be ignored. Localized delivery of anticancer drug has been developed as an effective administration method of chemotherapeutic agents formulated into biodegradable polymeric microspheres (Babu et al., Citation2006), injectable hydrogels (Chung et al., Citation2009) and polymer implants (Denkba et al., Citation2000). But these drug delivery systems are only capable of delivering one drug. Thus, the combination chemotherapy and the synergistic action of potent combinations using additional drug will be expected in the future. PLGA micro-device was a self-contained degradable drug delivery system capable of loading multiple drug, whose separated chambers can reduce the possible interactions between drugs and increase drug stability. So the novel degradable micro-device was desired as a localized delivery system for combination chemotherapy, which can exert its anti-tumor activity by improving local drug concentration around tumor and reduce its adverse effects.
Conclusions
In this study, we developed a PLGA micro-device for the controlled release and local targeting of 5-FU. A honeycomb-structure micro-device was manufactured using the biocompatible and biodegradable materials. According to experimental data, the release of 5-FU from the micro-device in vitro and in vivo was sustained for more than 7 days. Moreover, a good correlation was obtained between the cumulative release in vitro and the cumulative release in vivo. In addition, the anti-tumor activities were also investigated in tumor bearing mice, which were enhanced by loading 5-FU in micro-device compared with 5-FU injection. Localizing administration improved the drug concentration, thereby enhanced the therapeutic effects and reduced the normal tissues toxicity. As a result, implantable drug delivery systems provided a good location targeting approach for post-surgical chemotherapy against solid tumor. At the same time, the structure of honeycomb provided the potential for delivery of a combination of synergistically acting chemotherapeutic agents.
Acknowledgments
This study is supported by a grant from the National High Technology Research and Development Program of China (863 Program, No:2003AA404170), and National Natural Science Foundation of China (Grant Number: 30730110).
Declaration of Interest
We have no financial and personal relationships with other people or organizations that can inappropriately influence our work. There is no professional or other personal interest of any nature or kind in any product, service and/or company that could be construed as influencing the position presented in, or the review of, the manuscript entitled.
References
- Atilla A., Francis L., Albert G., 2009. Identifying mechanisms of chronotolerance and chronoefficacy for the anticancer drugs 5-fluorouracil and oxaliplatin by computational modeling. Eur. J. Pharm. Sci. 36,20–38.
- Alexis, F., 2005. Factors affecting the degradation and drug release mechanism of poly(lactic acid) and poly[(lactic acid)-co-(glycolic acid)]. Polym. Int. 54, 36–46.
- Babu, V.R., Sairam, M., Hosamani, K.M., Aminabhavi, T.M., 2006. Development of 5-fluorouracil loaded poly(acrylamide-co-methylmethacrylate) novel core-shell microspheres: In vitro release studies. Int. J. Pharm. 325, 55–62.
- Balmayor, E.R., Tuzlakoglu, K., Azevedo, H.S., Reis, R.L., 2009. Preparation and characterization of starch-poly-ϵ-caprolactone microparticles incorporating bioactive agents for drug delivery and tissue engineering applications. Acta Biomater. 5, 1035–1045.
- Berruti, A., Bitossi, R., Bottini, A., Bonardi, S., Donadio, M., Nigro, C., Bertetto, O., Danese, S., Bertone, E., Sarobba, M.G., Farris, A., Katsaros, D., Castiglione, F., Volpe, T., Lattuada, S., Mancarella, S., Dogliotti, L., 2005. Combination regimen of epirubicin, vinorelbine and 5-fluorouracil continuous infusion as first-line chemotherapy in anthracycline-naïve metastatic breast cancer patients. Eur. J. Cancer. 41, 249–255.
- Chung, T.W., Lin, S.Y., Liu, D.Z., Tyan, Y.C., Yang, J.S., 2009. Sustained release of 5-FU from Poloxamer gels interpenetrated by crosslinking chitosan network. Int. J. Pharm. 382, 39–44.
- Corrie, P.G., 2008. Cytotoxic chemotherapy: clinical aspects. Medicine. 36, 24–28.
- Denkba S. E. B., Seyyal, M., kin, E. P., 2000. Implantable 5-fluorouracil loaded chitosan scaffolds prepared by wet spinning. J. Membr. Sci. 172, 33–38.
- Dorta, M.J., Santoveña, A., Llabrés, M., Fariña, J.B., 2002. Potential applications of PLGA film-implants in modulating in vitro drugs release. Int. J. Pharm. 248, 149–156.
- Grayson, A.C.R., Shawgo, R.S., Li, Y., Cima, M.J., 2004. Electronic MEMS for triggered delivery. Adv. Drug Deliv. Rev. 56, 173–184.
- Jones, D.S., Bruschi, M.L., Freitas, O.D., Gremião, M.P.D., Lara, E.H.G., Andrews, G.P., 2009. Rheological, mechanical and mucoadhesive properties of thermoresponsive, bioadhesive binary mixtures composed of poloxamer 407 and carbopol 974P designed as platforms for implantable drug delivery systems for use in the oral cavity. Int. J. Pharm. 372, 49–58.
- Kasperczyk Stoklosa, K., Dobrzynski, P., Stepien, K., Kaczmarczyk, B., Dzierzega-Lecznar, A. 2009. Designing bioresorbable polyester matrices for controlled doxorubicin release in glioma therapy. Int. J. Pharm. 382, 124–129.
- Kim, J.H., Taluja, A., Knutson, K., Bae, Y.H., 2005. Stability of bovine serum albumin complexed with PEG-poly(l-histidine) diblock copolymer in PLGA microspheres. J. Control. Release. 109, 86–100.
- Kim, M.S., Seo, K.S., Hyun, H., Kim, S.K., Khang, G., Lee, H.B., 2005. Sustained release of bovine serum albumin using implantable wafers prepared by MPEG–PLGA diblock copolymers. Int. J. Pharm. 304, 165–177.
- Klose, D., Siepmann, F., Willart, J.F., Descamps, M., Siepmann, J., 2010. Drug release from PLGA-based microparticles: Effects of the “microparticle:bulk fluid” ratio. Int. J. Pharm. 383, 123–131.
- Kumari, A., Yadav, S.K., Yadav, S.C., 2010. Biodegradable polymeric nanoparticles based drug delivery systems. Colloids Surf., B. 75, 1–18.
- Langer, B., 1991. Polymer implants for drug delivery in the brain. J. Control. Release. 16, 53–59.
- Lemaire, V., Belair, J., Hildgen, P., 2003. Structural modeling of drug release from biodegradable porous matrices based on a combined diffusion/erosion process. Int. J. Pharm. 258, 95–107.
- Mack, B.C., Wright, K.W., Davis, M.E., 2009. A biodegradable filament for controlled drug delivery. J. Control. Release. 139, 205–211.
- Martin K., Roland B., 2008. Development of an in situ forming PLGA drug delivery system I. Characterization of a non-aqueous protein precipitation. Eur. J. Pharm. Sci. 35, 283–292.
- Mishra, P. K., Gulbake, A., Jain, A., Vyas, S. P., Jain, S. K., 2009. Targeted delivery of an anti-cancer agent via steroid coupled liposomes. Drug Delivery. 16, 437–447.
- Moreno, D., Zalba, S., Colom, H., Trocóniz, I.F., Ilarduya, C.T., Garrido, M.J., 2009. Biopharmaceutic and pharmacodynamic modeling of the in vitro antiproliferative effect of new controlled delivery systems of cisplatin. Eur. J. Pharm. Sci. 37, 341–350.
- Nagarwal, R.C., Kant, S., Singh, P.N., Maiti, P., Pandit, J.K., 2009. Polymeric nanoparticulate system: A potential approach for ocular drug delivery. J. Control. Release. 136, 2–13.
- Nampoothiri, K. M., Nair, N.R., John, R. P., 2010. An overview of the recent developments in polylactide (PLA) research. Bioresour. Technol. 101, 8493–8501.
- Ozer, I., Bostanci, E.B., Orug, T., Ozogul, Y.B., Ulas, M., Ercan, M., Kece, C., Atalay, F., Akoglu, M., 2009. Surgical outcomes and survival after multiorgan resection for locally advanced gastric cancer. Am. J. Surg. 198, 25–30.
- Poorter, R. L., Peters, G. J., Bakker, P. J. M., Taat, C. W., Biermans-van Lecuwe, D. M. J., Codacci-Pisanelli, G., Noordhuis, P., Oosting, J., Veenhof, C. H. N., 1995. Intermittent continuous infusion of 5-fluorouracil and low dose oral leucovorin in patients with gastrointestinal cancer: Relationship between plasma concentrations and clinical parameters. Eur. J. Cancer. 31, 1465–1470.
- Qian, F., Stowe, N., Liu, E.H., Saidela, G.M., Gao, J. 2003. Quantification of in vivo doxorubicin transport from PLGA millirods in thermoablated rat livers. J. Control. Release. 91, 157–166.
- Rabin, C., Liang, Y., Ehrlichman, R.S., Budhian,A., Metzger, K.L., Majewski-Tiedeken, C., Winey, K.I., Siegel, S.J. 2008. In vitro and in vivo demonstration of risperidone implants in mice. Schizophr. Res. 98, 66–78.
- Ranganath, S. H., Wang, C.H., 2008. Biodegradable microfiber implants delivering paclitaxel for post-surgical chemotherapy against malignant glioma. Biomaterials. 29, 2996–3003.
- Santoveña, A., Fariña, J.B., Llabrés, M., Zhu, Y., Dannies, P., 2010. Pharmacokinetics analysis of sustained release hGH biodegradable implantable tablets using a mouse model of human ovarian cancer. Int. J. Pharm. 388, 175–180.
- Shukla, H.S., Hughes, L.E., Davis, P.W., Whitehead, R.H., Leach, K.G., 1977. Distribution of 5-fluorouracil to body tissues compared after intraluminal, intravenous, and intramural administration in gastrointestinal cancer. Am. J. Surg. 133, 346–350.
- Wang, G.H., Liu, S.J., Ueng, S.W., Chan, E.C., 2004. The release of cefazolin and gentamicin from biodegradable PLA/PGA beads. Int. J. Pharm. 273, 203–212.
- Wei, X.W., Gong, C.Y., Gou, M.L., Fu, S.Z., Guo, Q.F., Shi, S., Luo, F., Guo, G., Qiu, L.Y., Qian, Z.Y., 2009. Biodegradable poly(-caprolactone)–poly(ethylene glycol) copolymers as drug delivery system. Int. J. Pharm. 381, 1–18.
- Xiaosong L., Roland B., 2006. In situ forming microparticle system for controlled delivery of leuprolide acetate: Influence of the formulation and processing parameters. Eur. J. Pharm. Sci. 27,143–149.
- Xie, J., Marijnissen, J.C.M., Wang, C.H., 2006. Microparticles developed by electrohydrodynamic atomization for the local delivery of anticancer drug to treat C6 glioma in vitro. Biomaterials. 27, 3321–3332.
- Yadav, A. K., Agarwal, A., Rai, G., Mishra, P., Jain, S., Mishra, Anil K., Agrawal, H., Agrawal, G. P., 2010. Development and characterization of hyaluronic acid decorated PLGA nanoparticles for delivery of 5-fluorouracil. Drug Delivery, 17, 561–572.
- Yang, R., Chen, T., Chen, H., Wang, W., 2005. Microfabrication of biodegradable (PLGA) honeycomb-structures and potential applications in implantable drug delivery. SENSOR ACTUAT B-CHEM. 106, 506–511.
- Yang, Y., Wang, J.C., Zhang, X., Lu, W.L., Zhang, Q., 2009. A novel mixed micelle gel with thermo-sensitive property for the local delivery of docetaxel. J. Control. Release. 135, 175–182.
- Zinutti, C., Barberi-Heyob, M., Hoffman, M., Maincent P., 1998. In-vivo evaluation of sustained release microspheres of 5-FU in rabbits. Int. J. Pharm. 166, 231–234.