Abstract
Context: Methotrexate (MTX) is used in the treatment of malignancies; however, its clinical application is limited by its toxic dose-related side effects. An alternative to overcome the toxicity of the MTX in healthy tissues is the design of an implantable device capable of controlling the delivery of this drug for an extended period within the tumor site.
Objective: To develop methotrexate-loaded poly(ε-caprolactone) implants (MTX PCL implants) and to demonstrate their efficacy as local drug delivery systems capable of inhibiting Ehrlich solid tumor bearing mice.
Materials and methods: MTX PCL implants were produced by the melt-molding technique and were characterized by FTIR, WAXS, DSC and SEM. The in vitro and in vivo release of MTX from the PCL implants was also evaluated. The efficacy of implants in inhibiting tumor cells in culture and the solid tumor in a murine model was revealed.
Results and discussion: The chemical and morphological integrity of the drug was preserved into the polymeric matrix. The in vitro and in vivo release processes of the MTX from the PCL implants were modulated by diffusion. MTX diffused from the implants revealed an antiproliferative effect on tumor cells. Finally, MTX controlled and sustained released from the polymeric implants efficiently reduced 42.7% of the solid tumor in mice paw.
Conclusion: These implantable devices represented a contribution to improve the efficacy and safety of chemotherapy treatments, promoting long-term local drug accumulation in the targeted site.
Introduction
Implants are controlled drug delivery systems based on biodegradable or non-biodegradable polymers. These implantable devices are designed to achieve prolonged therapeutic drug concentrations in the target tissues that are not readily accessible by conventional means while limiting the side effects from systemic drug exposure, as well as improving patient compliance (Lee et al., Citation2010). Non-biodegradable polymers act as a framework of the implants and provide well-controlled sustained drug release following a zero-order kinetic. Additionally, the non-biodegradable polymer implants have no initial adverse burst of the drug, which is superior to other types of devices (Yasukawa et al., Citation2005). However, the non-biodegradable polymers are not metabolized or degraded in the body, and therefore, the empty device must be removed by a second surgery, which exposes the patient to potential complications (Mansoor et al., Citation2009). On the other hand, biodegradable implantable devices are composed of synthetic or natural biomaterials, which degrade into nontoxic byproducts by enzymatic or nonenzymatic hydrolysis or solubilize in vivo and can be eliminated safely by the human body (Lee et al., Citation2010). Devices made from these polymers do not elicit permanent chronic foreign body reactions (Chu, Citation2008) and do not require removal after the drug supply has been exhausted, representing an advantage over non-biodegradable implants (Lee et al., Citation2010).
A wide variety of natural and synthetic biodegradable polymers have been investigated for the development of implants. Natural polymers, such as bovine serum albumin, human serum albumin, collagen and gelatin, have been studied for drug delivery. However, the use of these polymers is limited due to their higher cost and questionable purity. Synthetic polymers, such as poly(amides), poly(amino acids), poly(alkyl-α-cyano acrylates), poly(esters), poly(orthoesters), poly(urethanes) and poly(acrylamides), have been increasingly used to deliver drugs as they are free from most of the problems associated with the natural polymers (Jain, Citation2000; Moura et al., Citation2011). The polyglycolic acid (PGA), polylactic acid (PLA) and the PGA/PLA copolymer, polylactic-co-glycolic acid and poly(ε-caprolactone) (PCL) are synthetic aliphatic poly(esters) extensively investigated as carriers for drug delivery systems.
The PCL is prepared by the ring-opening polymerization of the cyclic monomer ε-caprolactone (Van-Natta et al., Citation1934; Woodruff & Hutmacher, Citation2010). Catalysts such as stannous octoate are used to catalyze the polymerization and low molecular weight alcohols can be used to control the molecular weight of the polymer (Storey & Taylor, Citation1996; Woodruff & Hutmacher, Citation2010). There are various mechanisms which affect the polymerization of PCL and these are anionic, cationic, co-ordination and radical. Each method affects the resulting molecular weight, molecular weight distribution, end group composition and chemical structure of the copolymers (Okada, Citation2002). The PCL is a hydrophobic poly(ester) due to the presence of a sequence of four hydrocarbon that ends up defining a more hydrophobic behavior to the molecule (Van-Natta et al., Citation1934). This polymer degrades through hydrolysis due to ester bonds. The release of the acid by-products and oligomers during the biodegradation process of the PCL could result in inflammatory reaction in vivo (Bergsma et al., Citation1995). However, if the surrounding tissue is able to buffer the pH changes provided by the acid degradation products, disturbances may not arise. Additionally, the degradation rate of PCL is very slow (2 to 3 years), depending of the starting molecular weight of the device or implant, which minimize the probability of development of a severe inflammatory response due to the lack of accumulation of the by-products of the PCL (Woodruff & Hutmacher, Citation2010). Finally, the slow rate of hydrolytic cleavage of the PCL makes it suitable for long-term delivery extending over a period of more than 1 year (Sinha et al., Citation2004; Bourges et al., Citation2006; Ayres et al., Citation2007; Nair & Laurencin, Citation2007).
The PCL, a semi-crystalline poly(ester), has the glass transition temperature of −60 °C and melting point ranging between 59 °C and 64 °C, depending upon its crystalline nature (Barbato et al., Citation2001; Sinha et al., Citation2004). These thermal properties were capitalized to fabricate drug delivery systems by means of processes such as melt molding (Ayres et al., Citation2007), extrusion (Bourges et al., Citation2006) without risking neither the polymer nor the drug molecular integrity due to an aggressive heating treatment (Sprockel et al., Citation1997; Lemmouchi et al., Citation1998; Carcaboso et al., Citation2010). Recently, the pharmaceutical companies have adopted the injection molding as a technique for designing polymeric drug delivery systems, due to the possibility to manufacture in large scale (Altpeter et al., Citation2004).
Studies using PCL implants prepared by different techniques and containing various anticancer active principles have been reported. For example, the melt-molding compression technique has been applied to manufacture topotecan-loaded PCL implants. These episcleral implants contributed to understand the active role of the ocular tissues, sclera and choroid in the clearance of topotecan during its transient path toward the intraocular compartments (Carcaboso et al., Citation2010). Hydrophilic 5-fluorouracil (Hou et al., Citation2011) and bis(maltolato)oxovanadium (Jackson et al., Citation1997) loaded PCL implantable devices were fabricated by melt molding compression technique and injection molding techniques, respectively. These drug delivery systems provided the controlled release of the antitumor drugs and locally inhibited the tumor growth.
Taking advantage of the biocompatibility, high permeability to many drugs, including the anticancer agents and the capability of designing drug delivery systems, in this study, PCL was used to produce implants incorporated into methotrexate (MTX) by the melt-molding technique. The methotrexate is a folate antimetabolite and interferes with the formation of DNA, RNA and proteins (Ackland & Schilsky, Citation1987; Ayyappan et al., Citation2010). Its main target is the dihydrofolate reductase, which inhibits the synthesis of new DNA by restricting the supply of deoxythymidine triphosphate and of purine nucleotides, leads to the misincorporation of the uracil into DNA (Goulian et al., Citation1980; Ackland & Schilsky, Citation1987). The methotrexate is widely used in the treatment of malignancies including childhood acute lymphocytic leukemia, osteosarcoma, non-Hodgkin’s lymphoma, Hodgkin’s disease, head and neck cancer, lung cancer, breast cancer, psoriasis, choriocarcinoma and related trophoblastic tumors (Calabresi, Citation1975; Seo et al., Citation2009). The methotrexate-loaded PCL implants were characterized in terms of content uniformity and analyzed by attenuated total reflectance-fourier transform infrared, wide angle X-ray scattering and differential scanning calorimetry to determine how the drug was distributed and interacted with the polymer. Morphological analysis was conducted by Scanning Electron Microscopy. The in vitro and in vivo release of the drug from the PCL implants was also evaluated to verify the controlled release of the methotrexate from the implantable devices. Furthermore, the antiproliferative activity of the methotrexate released from the polymeric implants was determined using tumor cells in culture. Finally, the therapeutic efficacy of this antimetabolite drug, locally leached from the PCL implantable devices, was demonstrated by means of the reduction of the Ehrlich solid tumor growth in a murine model for a prolonged period of time. It was hypothesized that the methotrexate-loaded PCL implants may be potential polymeric drug delivery systems for the treatment of human cancer.
Methods
Preparation of the implants containing PCL and methotrexate
The implants were prepared by fully blending MTX (Sigma Chemical Co., St. Louis, MO) particles with melting PCL (MW ∼14,000; density = 1.145 g/mL at 25 °C, Sigma Chemical Co.) and then molding the blends into spherical implants using a metallic mold. Briefly, PCL was heated until it was completely melted. Afterward MTX was added slowly into the melting PCL and mixed at approximately 70 °C for 20 min at a screw speed of 50 rpm (Cheng et al., Citation2009). The resultant blend was collected and further molded into spherical implants (6 mm in diameter) using a metallic mold at approximately 70 °C. The MTX-loaded PCL implants (MTX PCL implants) contained approximately 6.25% (w/w) of the drug corresponding to 1 mg of MTX. Implants without drug were also prepared (PCL implants).
Content uniformity test for the MTX PCL implants
For the determination of content uniformity of MTX in the PCL implants, the procedure stated in the general chapter <905> uniformity of dosage units of the United States Pharmacopeia was followed (United States Pharmacopeia, Citation2006). Ten implants were selected and weighted. The sample solutions were prepared as followed: each implant was dissolved in 50 mL of 0.1 mol/L hydrochloric acid. An aliquot (1 mL) of the resultant solution was transferred to volumetric flask (10 mL) and the volume was completed with the same solvent. The MTX standard solution was prepared by dissolving 1 mg of the drug in a 50 mL volumetric flask and using the same procedure described for preparing the sample solutions. The amount of MTX in the implant was determined by measuring the absorbance at 307 nm. The obtained values of the amount of MTX in each implant (mg) were estimated and the results were expressed as the percent of the pre-indicated value (approximately 1 mg). The relative standard deviation was also calculated.
Characterization
Fourier transform infrared
Infrared spectra were collected in a Fourier transform infrared spectrophotometer (FTIR; Perkin Elmer, Waltham, MA, model Spectrum 1000). Measurements were carried out using the attenuated total reflectance technique. Each spectrum was a result of 32 scans with a resolution of 4 cm−1.
Wide angle X-ray scattering
Wide angle X-ray scattering (WAXS) was performed in a Philips PW 3710 diffractometer with a copper target (λ = 1.54 Å) and Ni filters. Scans were performed from 2θ = 3.50 at rates of 0.01° min−1.
Differential scanning calorimetry
Differential scanning calorimetric (DSC) thermograms were obtained on a Mettler Toledo DSC (Schwerzenbach, Switzerland). Samples were put into aluminum pans. The calorimeter was calibrated for temperature and heat flow accuracy using pure indium melting (m.p. 156.6 °C and ΔH = 25.45 J g−1). The temperature ranged from 30–290 °C with a heating rate of 10 °C min−1 under nitrogen atmosphere.
Thermal gravimetric analysis
Thermal gravimetric analysis (TGA) was performed using a Mettler Toledo 851 e TGA/SDTA. Nitrogen was used as purge gas and the heating rate was kept constant at 10 °C min−1.
Scanning electron microscopy
Scanning electron microscopy (SEM) was performed using a JEOL microscope (model JSM – 6360LV) operating at 15 kV. MTX PCL implants were fractured and mounted on aluminum stubs using double-sided adhesive tape. Prior to microscopical examination, all the samples were sputter-coated with a gold layer under argon atmosphere using a sputter apparatus (Balzers Union SCD 040 unit, Balzers, Germany). The implants surfaces were viewed at 1000–10 000× magnification, and the images were transferred to the computer by means of a digital image transference interface. The photomicrographs were adjusted using the software Adobe Photoshop 6.0 and Adobe Illustrator 9.01 (Adobe Systems Incorporated, 2000, San Jose, CA).
In vitro release of MTX from the PCL implants
The United States Pharmacopeia states in the general chapter <1092> the dissolution procedure: development and validation that “sink conditions are defined as the volume of medium at least three times that required in order to form a saturated solution of drug substance. When sink conditions are present, it is more likely that dissolution results will reflect the properties of the dosage form” (United States Pharmacopeia, Citation2006).
The in vitro release study was carried out under sink conditions during 105 days. Assuming that the solubility of MTX is about 43,64 mg/mL at pH 7.4 (Yousefi et al., Citation2010), sink conditions are achieved with 0.22 mL at least for each implant. Five implants were immersed inside three different tubes containing 1 mL of PBS. The tubes were placed inside a shaker incubator set at 37 °C and 30 rpm. At predetermined intervals (0, 7, 15, 30, 45, 60, 75, 90 and 105 d), 1 mL of the medium was sampled and 1 mL of fresh medium was immediately added to each tube. The release profile was evaluated as the cumulative percentage of MTX released in the medium. The amount of MTX released was measured by measuring the absorbance at 307 nm.
Measurement of the in vitro weight loss of PCL implants
In vitro degradation study was evaluated by recording the weight loss of PCL implants (without MTX) over 105 d in PBS. The implants were placed in different tubes containing 3 mL of PBS (n = 5). Those tubes were placed inside a shaker incubator set at 37 °C and 30 rpm. At each time point (0, 7, 15, 30, 45, 60, 75, 90 and 105 d), the implants were retrieved from the PBS, rinsed with deionized water and vacuum-dried for 48 h before weight loss was analyzed. The percentage of weight loss was obtained by the ratio between the weight of the implants before and after incubation.
In vitro antimoral activity
Cell culture
Human cervix carcinoma (HeLa) cells were cultured in RPMI medium (Sigma) supplemented with 10% fetal bovine serum (Hyclone), 60 mg/mL of streptomycin and 100 mg/mL of penicillin in a humidified atmosphere of 5% CO2. Cells were seeded at 2 × 104 cells/cm2. Culture medium was changed every 48 h to avoid nutrient depletion.
Cytotoxicity of MTX PCL implants against tumor cell line
The effect of MTX released from PCL implants, pure MTX and PCL implants (without drug) on tumor cell proliferation was determined using the MTT cell proliferation assay. Briefly, the cells were plated in 96-wells plates (1 × 105 cells/well) in RPMI 1640 medium supplemented with 10% (v/v) fetal bovine serum and incubated for 24 h at 37 °C in humid atmosphere with 5% CO2 to adhesion. After 24 h of settling down, cells were washed with the culture medium and incubated in solutions containing pure MTX, MTX released from the PCL implants after 7 d of incubation in the culture medium and polymeric implants (without drug) (n = 10 for each sample). The solution of the drug was prepared by dissolving the MTX in 100% DMSO and diluting 100 times using culture medium. The MTX concentration in the solution of the pure drug was identical to the concentration of the MTX released from the PCL implants after 7 d of incubation in the culture medium. After 48 h of incubation, the culture medium was removed and 100 µL of MTT (1 mg/mL in PBS) was added to each well. After 2 h of incubation at 37 °C, cells were lysed with 100 µL of isopropanol, and absorbance values were measured at 550 nm using a microplate reader Spectramax M5e (Molecular Devices, Sunnyvale, CA). All the experiments were performed in triplicate. The cytotoxicity was calculated by the percentage of reduction of the tumor cells treated with the MTX when compared with the tumor cells untreated with the drug (control cells).
In vivo antimoral activity
Animals
Six- to eight-weeks-old female Swiss mice from the Federal University of Minas Gerais (UFMG) were maintained in individual cages, with food and water ad libitum, and controlled temperature and humidity in the animal house of the Federal University of São João Del Rei (UFSJ). Experiments were approved by the Ethics Committee in Animal Experimentation at UFSJ.
Ehrlich ascitic and solid tumor
The Ehrlich ascitic tumor, derived from a spontaneous murine mammary adenocarcinoma, was maintained in the ascitic form by sequential passages in Swiss mice, by means of weekly i.p. transplantations of 2.5 × 106 tumor cells. To the experiments with solid tumor, 2.5 × 106 tumor cells were injected in a volume of 0.05 mL in the footpad of rats (Kleeb et al., Citation1997).
Implantation of MTX PCL implants
At 5 d post-inoculation of the tumor cells in the left footpad, the animals were anesthetized with a mixture of (10 mg kg−1) xylazine and (100 mg kg−1) ketamine hydrochloride (i.p.). Their hair nearby the solid tumor was shaved and the skin wiped with 70% ethanol. MTX PCL implants were aseptically inserted into a subcutaneous pouch that was made with curved artery forceps (treated groups). The same implantation procedure was carried out to insert the PLC implants without MTX (untreated group). After the implantation procedure, the animals were maintained in individual cages and provided with chow pellets and water ad libitum. The light/dark cycle was 12:12 h with lights on at 7:00 AM and lights off at 7:00 PM. Post-operatively, the animals were monitored for any signs of infection at the operative site, or upon discomfort or distress; any mice presenting such signs were immediately sacrificed.
Evaluation of the tumoral growth
After the insertion of the MTX PCL implants, every day until the 13th day post-inoculation of the tumor cells in the left footpad, tumoral growth was evaluated by measuring the paw thickness, using a digital pachymeter. The antineoplasic activity of the MTX released from the PCL implants was evaluated as the percentage of reduction of the paw thickness of the animals in the treated groups that received the implants when compared with the control group (n = 6 for each time, for each group).
Histological analysis
The paw of the sacrificed animals of the treated and untreated groups were collected and representative slices were fixed in formalin (10% in isotonic saline), and embedded in paraffin, at which time 5 µm thick sections were obtained. The sections were stained with hematoxylin and eosin and examined under a light microscope. The images were digitized through a JVC TK-1270/JGB microcamera.
In vivo release of MTX from the PCL implants
At 2, 4, 6, 8, 12, 16 and 18 d post-implantation of the MTX-loaded PCL devices, animals (n = 5) containing the solid tumor were euthanized by cervical dislocation and the MTX PCL implants were carefully removed. The MTX PCL implant was dissolved in 50 mL of 0.1 mol/L hydrochloric acid. An aliquot (1 mL) of the resultant solution was transferred to volumetric flask (10 mL) and the volume was completed with the same solvent. The content of MTX remaining in the PCL implants was determined by measuring the absorbance at 307 nm.
Statistical analysis
Results were expressed as mean ± standard deviation. Data were tested for normality and investigated for statistical significance using Student’s t-test. A p value less than 0.05 was considered significant. Statistical analysis was performed using a Graph-Pad Prim 4.01.
Results
Preparation of the implants containing PCL and MTX
MTX-loaded PCL implants were prepared by blending MTX particles with melting PCL at approximately 70 °C. The PCL, a white hydrophobic polyester material, functioned as binder during the fabrication process due to its appropriated viscosity when heated up to 60 °C (Cheng et al., Citation2009). As the melting of MTX ranged from 185 °C to 204 °C (Pace, Citation2004), its stability against heating makes it suitable for incorporation into polymeric matrix during melting of the PCL. The resultant blend was collected and further molded into spherical implants (6 mm in diameter), having the structure of a solid dispersion in which MTX was homogeneously distributed in the polymeric matrix.
Content uniformity test for the MTX PCL implants
The determined MTX content values in all PCL implants were between 85.0–115.0% of the predefined value of the drug in the implant formulation [6.25% (w/w), corresponding to 1.0 mg of MTX per PCL implant]. Additionally, the relative standard deviation calculated was 3.4%. The results of content uniformity demonstrated that MTX was homogeneously dispersed in the PCL implants, as confirmed by SEM, and the quality of the implantable devices.
Characterization
depicts infrared spectra of the pure MTX (), PCL implants (without drug) () and MTX PCL implants (). Typical infrared absorptions bands observed in PCL can be detected in spectra of and c, such as (Elzein et al., Citation2004) 2944 cm−1 and 2866 cm−1, which were due to the asymmetric and symmetric CH2 stretching, respectively. The intense infrared absorption band at 1721 cm−1 was due to the carbonyl stretching vibration. The infrared band at 1293 cm−1 was attributed to the backbone C–C and C–O stretching modes in the crystalline PCL. Finally, the bands at 1238 cm−1 and 1168 cm−1 were related to the asymmetric and symmetric COC stretching vibrations, respectively. The obtained FTIR results corroborated with those previously described (Coleman & Zarian, Citation1979).
Figure 1. FTIR spectroscopy of the pure MTX (a), PCL implants (without drug) (b) and MTX PCL implants (c).
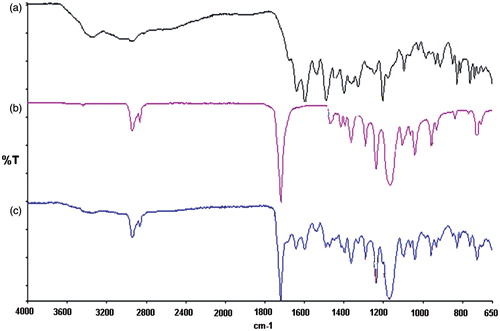
The FTIR spectral analysis revealed characteristic absorption bands observed in pure MTX ( and c) (Ayyappan et al., Citation2010): a broad band at 3344 cm−1 corresponding to the overlapping of primary amine and hydroxyl groups. A weak shoulder absorbing at ∼2948 cm−1 was attributed to symmetric and asymmetric CH2 stretching vibrations. The intense infrared absorption band at 1640 cm−1 was due to the carbonyl stretching vibration partially overlapped with the δ(N–H) band. The infrared bands at 1364 cm−1 and 829 cm−1 were also observed corresponding to hydroxyl bond in plane and out-of-plane bending, respectively.
The FTIR spectrum of MTX PCL implants () demonstrated that typical absorption bands visualized in pure MTX and polymer can be clearly identified. The existence of the infrared absorption bands due to MTX and PCL in the spectrum of the polymeric matrix containing the drug as well as the absence of new bands for MTX PCL implants suggested that the molecular structures of the compounds were conserved during the process required to produce these polymeric drug delivery systems.
shows WAXS of pure MTX (), PCL implants (without drug) () and MTX PCL implants (). The WAXS patterns corresponding to the pure MTX () exhibited several strong scattering peaks of lower and higher intensities, demonstrating the crystalline nature of the MTX. The WAXS pattern of MTX was similar to that previously reported (Jingou et al., Citation2011). The WAXS recorded for PCL () demonstrated two characteristic peaks between 20° and 25°, confirming its semi-crystalline structure (Wang & Gou, Citation2008), and a series of peaks above 20°. For the MTX PCL implants (), the scattering peaks of the polymer were preserved, indicating the existence of the crystalline structure of the PCL in the implantable devices. Furthermore, the original crystalline structure of the MTX into the polymeric implants was also conserved, but the scattering peaks of the drug presented lower intensity. This suggested that a certain fraction of the MTX crystals was dissolved or dispersed in the PCL matrix.
depicts DSC thermograms of the pure MTX (), PCL implants (without drug) (), pure PLC (not melted) () and MTX PCL implants (). The DSC thermogram corresponding to the pure PCL () exhibited a melting sharp peak at approximately 60 °C. In contrast, the PCL implants () had a broad peak, indicating a more heterogeneous crystalline structure of the polymer probably due to the preparation process of implants. The DSC recorded to pure MTX () demonstrated an endotherm in the temperature range between 110 °C and 150 °C. The TGA curves of MTX (data not shown) presented a weight loss of approximately 11% in the similar temperature range. These events, observed in the DSC and TGA curves, were probably associated to the desolvation of the drug. The literature has shown the existence of trihydrates as well as tetrahydrates of MTX (Coleman & Zarian, Citation1979); however, the percentage of weight loss in this step of thermal degradation indicated that the MTX studied is trihydrate. Further, a broad peak at 240–270 °C was also verified due to the decomposition of the MTX, which overlapped the melting endotherm of the drug. The obtained results corroborated with those previously described (Chan & Gonda, Citation1991; Chadha et al., Citation2009). For the MTX PCL implants (), the DSC thermogram showed all endothermic events corresponding to the PCL and the drug. However, the melting endothermic peak of PCL showed a shift to lower onset temperature (Tonset) and lower intensity compared to the same peak of the PCL implant (without drug – ). This event was attributed to the disturbance of the semi-crystalline structure of the PCL by the dispersed MTX into its polymeric matrix. Further, the endothermic event attributed to the desolvation of the MTX was shifted to higher temperatures, because the melting of the polymeric matrix probably functioned as a barrier to the release of the water (Chan & Gonda, Citation1991). Finally, a broad peak at 240–270 °C was also observed, which probably represented the overlapping of the decomposition and melting of the MTX. The DSC results suggested the existence of a physical dispersion of the drug in the polymeric matrix as indicated by WAXS results.
Figure 3. DSC thermograms of the pure MTX (a), PCL implants (without drug) (b), pure PLC (not melted) (c) and MTX PCL implants (d).
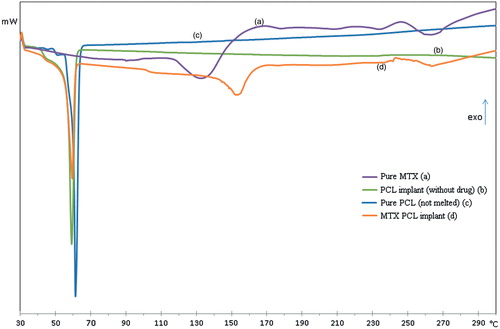
shows the SEM of the internal surface of the MTX PCL implants. The internal surface revealed the presence of small particles homogeneously distributed, corresponding to the fraction of MTX crystals which did not dissolve in the melting PCL. As no large MTX aggregates could be visualized in the surfaces of the implants, it can be suggested that the manufacture procedure of the implantable devices yielded the uniform distribution of the drug crystal into the polymeric matrix.
Measurement of weight loss of PCL implants
In the initial phase of the degradation process of the PCL, no weight loss is observed. The random cleavage of the polymeric chains leads to an initial decrease of molecular weight without any significant mass loss. In the second phase of this polymer degradation, it is observed the onset of weight loss that has been attributed to the diffusion of small polymeric fragments from the matrix (Merkli, Citation1998; Sinha et al., Citation2004). In this study, there was no indication that polymer degradation had begun during the follow-up period, because the PLC did not exhibit progressive mass loss ().
In vitro release of MTX from the PCL implants
shows also the cumulative release curve of MTX from PCL implants over a period of 105 d. The implants led to a controlled release of the drug that can be divided into two different kinetic phases. During the first 7 d, approximately 25.40% of the MTX was leached from the implantable devices. This initial burst release was controlled mainly by diffusion of the drug accumulated on the surface of the polymeric system (Bourges et al., Citation2006). In the second phase, approximately 55% of the drug was gradually released from the implants in a constant regime.
In vivo release of MTX from the PCL implants
The profile of in vivo MTX release from the PCL implants can be observed in . The MTX-loaded PCL implants maintained a sustained release of the drug for 18 d within subcutaneous tissue of the mice. The implantable devices exhibited an initial burst release. Approximately 49.9% of the MTX was leached during the first 4 d due to the diffusion of the drug on the surface of the polymeric implant. Afterwards, almost 46% of the MTX was controlled and sustained released from the devices by permeating into PCL matrix and subsequent drug molecular diffusion through PCL matrix or voids left by released drug (Cheng et al., Citation2009). Finally, the MTX release rate from PCL implants in vivo was approximately 53 μg/d.
Figure 7. In vivo cumulative MTX (%) released from PCL implants inserted into the subcutaneous tissue of mice. Results represent mean ± standard deviation (n = 5 for each time).
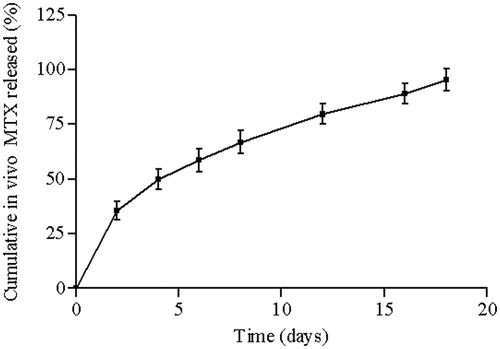
It was observed that approximately 40% of MTX was released from PCL implants in vitro and 95% of drug was delivered by PCL implants in vivo during 18 d. The differences between the physiological environment (in vivo test) and the simulated physiological conditions (in vitro test) explain the lack of correlation between the in vivo and in vitro profile release of MTX from PCL implants (Pinto et al., Citation2011).
In vitro antimoral activity
shows the cytotoxicity of the solution of PCL implants (without drug) against HeLa cells. The PCL implants revealed no antiproliferative effect on tumor cells. Moreover, it was also evaluated the cytotoxicity of the solutions of MTX released from the PCL implants after 7 d of incubation in the culture medium and of pure drug at identical concentration of the MTX leached from the implants. Pure MTX and MTX released from the polymeric system inhibited HeLa cell growth by about 59.8 ± 6.6% and 62.1 ± 4.5%, respectively. There was no significant difference (p > 0.05) on the viability of tumor cells cultured in direct contact with the MTX. Therefore, the MTX incorporated into PCL implants had similar antitumor potential as free MTX.
Evaluation of the tumoral growth
The antineoplasic activity of the MTX released from the PCL implants was evaluated as the percentage of reduction of the paw thickness of mice of the treated group that received the implants when compared with the untreated group. The MTX inhibited the tumor growth 1 d after the insertion of the implantable devices, and this effect was maintained until the end of the experiment (). By contrast, there was continuous tumor growth in paw of animals of the untreated group. The MTX reduced 42.7% of the Ehrlich solid tumor growth, as compared to untreated group. There was significant difference (p < 0.05) on the paw thickness of the treated and untreated groups. Therefore, the obtained result confirmed that MTX was eluted in the active form capable of exerting a cytotoxic effect on tumor cells.
Histological analysis
Postoperative unexpected findings and complications were not verified in mice subjected to this experiment. Tissue samples of the paw of mice of the untreated group exhibited extensive areas of necrosis, located mainly in the deep dermis (). Necrosis was associated with a diffuse inflammatory process, consisting of mononuclear cells predominantly (). Accordingly (Pinto et al., Citation2009), the inflammatory response on the Ehrlich tumor site is mediated by the neutrophil infiltration. The neutrophils produce chemotatic factors and reactive oxygen species that can activate macrophages to release TNF-α. This cascade of events is important for eliminating tumor cells, but an unregulated pattern can induce cell lesion and necrosis. On the other hand, tissues samples of mice of the treated group showed smaller necrotic areas and lower number of tumor cells (). The decreased necrosis was consistent with the reduction of the growth of the Ehrlich solid tumor on mice. The MTX controlled released from the PCL implants during 18 d probably was capable of reducing some inflammatory biomarkers, such as TNF-α, C-reactive protein and interleukin-6 (Vendramini-Costa et al., Citation2010), and consequently, decreasing necrotic areas. Furthermore, the MTX locally delivery by the polymeric implants was uptake by tumor cells probably through endocytosis. The intracellular MTX was released by a lysosomal process and delivered to the cytosol where it binds to its target enzyme (dihydrofolate reductase) and induced a strong S-phase block. This alteration in tumor cell cycle provided the antitumor activity in vivo, represented by the regression of the volume of the solid tumor (Wosikowski et al., Citation2003).
Figure 10. (a) Histological analyses revealed an extensive necrotic area in the deep dermis of mice paw of the untreated group after 18 days of the experiment (arrow, HE, 100×). (b) Inflammatory cells accumulated on the tumor site of mice paw of the untreated group (arrow, HE, 400×). (c) Smaller necrotic area was observed in mice paw of the treated group (receiving MTX PCL implants) after 18 days of the experiment (arrow, HE, 100×). (d) Tumor cells from both groups presented similar characteristics: pleomorphism and anaplasty, with diminished cytoplasm and frequent mitosis events. (HE 100×).
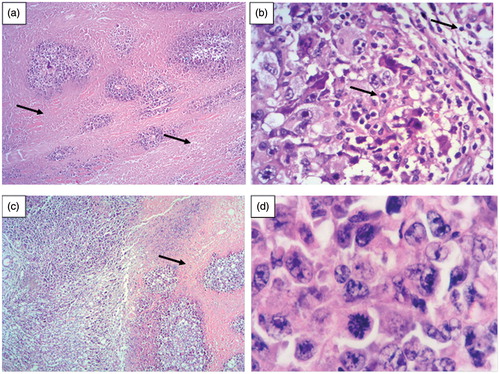
Discussion
MTX, a folate antimetabolite, is widely used in the treatment of malignancies including osteosarcoma, non-Hodgkin’s lymphoma, Hodgkin’s disease, head and neck cancer, lung cancer, breast cancer, psoriasis, choriocarcinoma and related trophoblastic tumors (Seo et al., Citation2009). However, the clinical application of this drug is limited by its toxic dose-related side effects. The lack of selectivity of the low molecular weight antitumor drug MTX is closely related to its pharmacokinetic properties, i.e. short half-life in the bloodstream and rapid diffusion throughout the body resulting in an essentially uniform tissue distribution (Wosikowski et al., Citation2003). An alternative to overcome the toxicity of the MTX in healthy tissues is the design of an implantable device capable of controlling the delivery of the anticancer drug for an extended period within the tumor site.
In this study, MTX-loaded PCL implants were developed by incorporating particles of the drug into the melted polymer and designing it into spherical devices. The PCL was selected as polymeric matrix due to its capability of designing this implantable drug delivery system, biocompatibility and versatility to entrap the MTX. The entrapment of the MTX into the PCL did not affect the chemical and morphological integrity of the drug, as confirmed by the FTIR, WAXS, DSC and SEM. Additionally, the PCL implants functioned as drug delivery systems, because the polymeric matrix was able to control the diffusion of the MTX for a prolonged period. In this study, the degradation of the PCL was not considered as the main responsible for the release of the drug, because this polyester is characterized by a very low-hydrolysis rate (as evidenced by the weight loss study), which can vary from months to years (Merkli, Citation1998).
The Ehrlich tumor is a fast-growing and aggressive adenocarcinoma that generates a local inflammatory response characterized by the increased vascular permeability which accounts for edema formation and promotes cell migration (Stewart, Citation1959; Vendramini-Costa et al., Citation2010). Cancer-associated inflammation includes the infiltration of white blood cells, prominently phagocytic cells such as macrophages, the presence of polypeptide messengers of inflammation (as tumor necrosis factor, interleukin-1 and chemokines) and the occurrence of tissue remodeling and angiogenesis (Vendramini-Costa et al., Citation2010). This cascade of events presents an unregulated pattern that can induce cell lesion and necrosis. The efficacy of MTX-loaded PCL implants in decreasing necrosis and growth of the Ehrlich solid tumor on mice paw was attributed to the following reasons: (1) The biphasic pattern of release of the MTX from the implants contributed to the inhibition of the tumor growth, because higher local concentration of the drug can be immediately achieved, as an attack dose; and the long-lasting release of MTX at a slower rate might be important in recruiting more tumor cells at the S-phase of the cycle (Carcaboso et al., Citation2010). It was previously mentioned that MTX induces a strong S-phase block, and this alteration in tumor cell cycle provides its antitumor activity. (2) The concentration of MTX released from the PCL implants (approximately 53 µg/day, according to the in vivo MTX release) was locally maintained in the therapeutic range for 18 d, without peak-and-valley drug levels, and efficiently provided tumor growth inhibitory effect in this experimental model. (3) The maintenance of the chemical integrity of the MTX embedded in the PCL matrix, prepared by melt molding technique, was an essential factor that assured its cytotoxicity towards tumor cells, inhibiting Ehrlich cell growth, clearly acting as an anticancer an anti-inflammatory drug.
In this study, MTX released from the PCL implants reduced 42.7% of the Ehrlich solid tumor growth, with no signals of toxicity observed. Among the studies that MTX is applied as an anticancer drug to murine models of tumor, Wosikowski et al. (2003) reported a reduction of 19% and 82% in tumor size, induced by subcutaneous inoculation of human breast cancer cells (ZR-75-1) and mesotheliomas (MSTO-211), respectively, after daily administration of 20 mg/kg MTX. Lee et al. (2011) described that a daily injection of the solution containing cucurbitacin B and MTX (150 mg/kg) inhibited the human osteosarcoma xenografts in nude mice in approximately 81%, when compared to the injections of pure MTX. The MTX-loaded PCL implants were demonstrated to be as effective as the MTX injections in reducing the tumor growth. However, these implantable devices offer the advantage of being controlled drug delivery system, providing the maintenance of the therapeutic levels in the target site, and avoiding the toxic risk to targeted tissues. Additionally, they do not need to be repeatedly administrated, which is usually necessary to preserve the therapeutic concentration of the chemotherapeutic drug delivered by parenteral route. Finally, these implantable devices were prepared based on PCL, a biodegradable polymer, so that they do not need to be removed by surgical process after complete drug release.
The favorable obtained results encourage the performance of new investigations to evaluate the feasibility of MTX-loaded PCL implants for the treatment of tumors and rheumatoid arthritis, because they overcome most of the disadvantages of the conventional therapies. For example, in the field of ophthalmology, the MTX-loaded PCL implants could be potentially used to treat primary intraocular lymphoma, a non-metastatic malignant lymphoid neoplasia that arises primarily within the eye. These implantable devices could be inserted into the vitreous cavity or episclera, overtaking the blood–retina barrier and achieving effective concentrations of drug in the target site. The systemic drug exposition would be reduced, and consequently, it would minimize the toxicity to the normal tissues. Additionally, the MTX PCL implants could be directly inserted in the joint for the treatment of arthritis, osteoarthritis or musculoskeletal disorders to reduce pain, inflammation and facilitate motion and function. The implantable devices would be localized in the joint cavity, avoiding the systemic concentration of the MTX, and consequently, minimizing the systemic side effects promoted by this drug (Pinto et al., Citation2012). In conclusion, the MTX-loaded PCL implants could be clinically applied to treat various tumors and/or inflammatory pathologies, because they might achieve therapeutic concentrations and long-term local drug accumulation in the targeted site.
Conclusion
In this study, MTX-loaded PCL implants were prepared using the melt-molding technique. The monolithic implants showed uniform dispersion of the drug in the polymeric matrix. Data obtained from FTIR demonstrated no detectable chemical interactions at the molecular level between drug and polymer. DSC and WAXS revealed not only the morphological integrity of the components of the implant, but also the physical dispersion of the MTX in the polymeric chains. The in vitro and in vivo release studies showed that MTX release process was predominantly modulated by diffusion of the drug through the polymeric matrix, because the degradation of the PCL did not contribute significantly for the delivery of the drug. Additionally, the in vitro and in vivo release curves could be divided into two different phases: an initial burst, which probably occurred due to the faster dissolution of the drug, and the sustained release phase, characterized by the low dissolution of the drug crystals in aqueous solutions. These implantable devices demonstrated their efficiency of decreasing necrosis and growth of the Ehrlich solid tumor on mice paw. The therapeutic levels of MTX controlled and sustained released from the PCL implants provided the recruitment of more tumor cells at the S-phase of the cycle, altering the life cycle of Ehrlich tumor cells. Finally, the favorable obtained results demonstrated that MTX-loaded PCL implants could be potentially applied as anticancer drug delivery systems for local and prolonged treatment of malignancies including osteosarcoma, head and neck cancer, lung cancer and breast cancer, among others.
Declaration of interest
The authors report no declarations of interest.
Acknowledgements
The authors acknowledge the financial support received from the following institutions: CNPq/MCT (Brazil), FAPEMIG (Minas Gerais – Brazil) and UFSJ (Minas Gerais – Brazil).
References
- Ackland SP, Schilsky RL. (1987). High-dose methotrexate: a critical reappraisal. J Clin Oncol 5:2017–31
- Altpeter H, Bevis MJ, Grijpma DW, Feijen J. (2004). Non-conventional injection molding of poly(lactide) and poly(e-caprolactone) intended for orthopedic applications. J Mater Sci Mater Med 15:175–84
- Ayres E, Oréfice RL, Yoshida MI. (2007). Phase morphology of hydrolysable polyurethanes derived from aqueous dispersions. Eur Polym J 43:3510–11
- Ayyappan S, Sundaraganesan N, Aroulmoji V, et al. (2010). Molecular structure, vibrational spectra and DFT molecular orbital calculations (TD-DFT and NMR) of the antiproliferative drug Methotrexate. Spectrochim Acta A 77:264–75
- Barbato F, La Rotonda MI, Maglio G, et al. (2001). Biodegradable microspheres of novel segmented poly(ether-esteramide)s based on poly(e-caprolactone) for the delivery of bioactive compounds. Biomaterials 22:1371–8
- Bergsma JE, Bruijn WC, Rozema FR, et al. (1995). Late degradation tissue response to poly(-lactide) bone plates and screws. Biomaterials 16:25–31
- Bourges JL, Bloquel C, Thomas A, et al. (2006). Intra-ocular implants for extended drug delivery: therapeutic applications. Adv Drug Deliv Rev 58:1182–202
- Calabresi P. (1975). The pharmacological basis of therapeutics. New York: Macmillan
- Carcaboso AM, Chiappetta DA, Operzzo JAW, et al. (2010). Episcleral implants for topotecan delivery to the posterior segment of the eye. Invest Ophthalmol Vis Sci 51:2126–34
- Chadha R, Poonam A, Rupinder K, et al. (2009). Characterization of solvatomorphs of methotrexate using thermoanalytical and other techniques. Acta Pharm 59:245–57
- Chan HK, Gonda I. (1991). Methotrexate: existence of different types of solid. Int J Pharm 68:179–90
- Cheng L, Shengrong G, Weiping W. (2009). Characterization and in vitro release of praziquantel from poly(ε-caprolactone) implants. Int J Pharm 377:112–9
- Chu CC. (2008). Biodegradable polymers: an overview. In: Wnek GE, Bowlin GL, eds. Encyclopedia of biomaterials and biomedical engineering. New York: Informa Healthcare USA, Inc.
- Coleman MM, Zarian J. (1979). Fourier-transform infrared studies of polymer blends. II. Poly(ε-caprolactone)–poly(vinyl chloride) system. J Polym Sci 17:837–50
- Elzein T, Nasser-Eddine M, Delaite C, et al. (2004). FTIR study of polycaprolactone chain organization at interfaces. J Colloid Interface Sci 273:381–7
- Goulian M, Bleibe B, Tseng BY. (1980). Methotrexate-induced misincorporation of uracil into DNA. Proc Acad Sci USA 77:1956–60
- Hou J, Li C, Cheng L, et al. (2011). Study on hydrophilic 5-fluorouracil release from hydrophobic poly(ε-caprolactone) cylindrical implants. Drug Dev Ind Pharm 37:1068–75
- Jackson JK, Min W, Cruz TF, et al. (1997). A polymer-based drug delivery system for the antineoplastic agent bis(maltolato)oxovanadium in mice. Br J Cancer 75:1014–20
- Jain RA. (2000). The manufacturing techniques of various drug loaded biodegradable poly(lactide-co-glycolide) (PLGA) devices. Biomaterials 21:2475–90
- Jingou J, Shilei H, Weigi L, et al. (2011). Preparation, characterization of hydrophilic and hydrophobic drug in combine loaded chitosan/cyclodextrin nanoparticles and in vitro release study. Colloids Surf B Biointerfaces 83:103–7
- Kleeb SR, Xavier JG, Frussa-Filho R, Dagli MLZ. (1997). Effect of haloperidol on the development of the solid Ehrlich tumor in mice. Life Sci 60:PL69–74
- Lee DH, Thoennissen NH, Goff C, et al. (2011). Synergistic effect of low-dose cucurbitacin B and low-dose methotrexate for treatment of human osteosarcoma. Cancer Lett 306:161–70
- Lee SS, Hughes P, Ross AD, Robinson MR. (2010). Biodegradable implants for sustained drug release in the eye. Pharm Res 27:2043–53
- Lemmouchi, Y, Schacht E, Kageruka P, et al. (1998). Biodegradable polyesters for controlled release of trypanocidal drugs: in vitro and in vivo studies. Biomaterials 19:1827–37
- Mansoor S, Kuppermann BD, Kenney MC. (2009). Intraocular sustained-release delivery systems for triamcinolone acetonide. Pharm Res 26:770–84
- Merkli A. (1998). Biodegradable polymers for the controlled release of ocular drugs. Prog Polym Sci 23:563–80
- Moura SAL, Lima LDC, Andrade SP, et al. (2011). Local drug delivery system: inhibition of inflammatory angiogenesis in a murine sponge model by dexamethasone-loaded polyurethane implants. J Pharm Sci 100:2886–95
- Nair LS, Laurencin CT. (2007). Biodegradable polymers as biomaterials. Prog Polym Sci 32:762–98
- Okada M. (2002). Chemical syntheses of biodegradable polymers. Progr Polym Sci 27:87–133
- Pace GW. (2004). United States Patent No: US 6,682,761 B2. bks5.books.google.ht/patents/US6682761.pdf
- Pinto FCH, Da Silva-Cunha AJ, Oréfice RL, et al. (2012). Controlled release of triamcinolone acetonide from polyurethane implantable devices: application for inhibition of inflammatory angiogenesis. J Mater Sci Mater Med 23:1431–45
- Pinto FCH, Menezes GB, Moura SAL, et al. (2009). Induction of apoptosis in tumor cells as a mechanism of tumor growth reduction in allergic mice. Pathol Res Pract 205:559–67
- Pinto FCH, Silva-Cunha A, Pianetti GA, et al. (2011). Montmorillonite clay based polyurethane nanocomposite as local triamcinolone acetonide delivery system. J Nanomater 2011:1--11. DOI: 10.1155/2011/528628
- Seo DH, Dong-Hyuk S, Young-Il J, et al. (2009). Methotrexate-incorporated polymeric nanoparticles of methoxy poly(ethyleneglycol)-grafted chitosan. Colloids Surf B Biointerfaces 69:157–63
- Sinha VR, Bansal K, Kaushik R, et al. (2004). Poly-ecaprolactone microspheres and nanospheres: an overview. Int J Pharm 278:1–23
- Sprockel OL, Sen M, Shivanand P, Prapaitrakul W. (1997). A melt-extrusion process for manufacturing matrix drug delivery systems. Int J Pharm 155:191–9
- Stewart HL. (1959). The cancer investigator. Cancer Res 19:804–18
- Storey RF, Taylor AE. (1996). Effect of stannous octoate concentration on the ethylene glycol initiated polymerization of epsilon-caprolactone. Abstr Pap Am Chem Soc 211:114–20
- The United States Pharmacopeia (USP 29). (2006). United States Pharmacopeial Convention Inc., CD-ROM, 29th edition (Insight Publishing Productivity), Rockville
- Van-Natta FJ, Hill JW, Carruthers WH. (1934). Polymerization and ring formation, ε-caprolactone and its polymers. J Am Chem Soc 56:455–9
- Vendramini-Costa DB, Castro IBD, Ruiz ALTG, et al. (2010). Effect of goniothalamin on the development of Ehrlich solid tumor in mice. Bioorg Medicinal Chem 18:6742–7
- Wang S, Gou S. (2008). Disodium norcantharidate loaded poly(ε-caprolactone) microspheres. I. Preparation and evaluation. Int J Pharm 350:130–7
- Woodruff MA, Hutmacher DW. (2010). The return of a forgotten polymer polycaprolactone in the 21st century. Prog Polym Sci 35:1217–56
- Wosikowski K, Biedermann E, Rattel B, et al. (2003). In vitro and in vivo antitumor activity of methotrexate conjugated to human serum albumin in human cancer cells. Clin Cancer Res 9:1917–26
- Yasukawa T, Ogura Y, Sakurai E, et al. (2005). Intraocular sustained drug delivery using implantable polymeric devices. Adv Drug Deliv Rev 57:2033–46
- Yousefi G, Gholamhossein Y, Foroutan SM, et al. (2010). Synthesis and characterization of methotrexate polyethylene glycol esters as a drug delivery system. Chem Pharm Bull 58:147–53