Abstract
Liver cancer has become one of the most common fatal cancers worldwide, with morbidity rates increasing each year. Wogonin (WG) is an attractive candidate for the development of new anti-cancer drugs. In this study, a novel glycyrrhetinic acid (GA)-modified WG liposome was developed for use in targeted anti-cancer therapy. Three types of WG preparations were investigated: free wogonin in solution (WG), passively targeted wogonin liposomes (WG-Lip) and GA-modified wogonin liposomes (GA-WG-Lip). The entrapment efficiency, size and zeta potential were measured. Cellular uptake, cytotoxicity, in vivo bio-distribution and anti-tumor efficacy were also investigated. Addition of GA to the liposomes did not diminish the high entrapment efficiency observed in the liposomes without GA. GA-WG-Lip showed the greatest uptake and had an IC50 value 1.46 times higher than that of WG-Lip. The GA-modified liposomes rapidly accumulated in the liver with a long retention time, and also displayed a better tumor inhibitory ratio than that of the unmodified liposomes. Overall, the data indicated that use of the GA-modified WG liposomes conferred improvements in bio-distribution, accumulation at the tumor and therapeutic efficacy, perhaps due to increased receptor-mediated uptake of liposomes by liver-targeted cells. Together, these data show that GA-WG-Lip is a promising means of targeted therapy for liver cancer.
Introduction
Over half a century following the initial launch of chemotherapy as a treatment against tumors, phytochemicals have emerged as an important class of anti-neoplastic drugs. In fact, 69% of anti-cancer drugs approved between 1940 and 2002 are either natural products or were developed based on the knowledge gained from natural products. Both the development and clinical use of phytopharmaceuticals have advanced rapidly in recent years. Traditional Chinese Medicines (TCMs) have been recognized as new sources of anti-cancer drugs and new chemotherapy adjuvants to enhance therapeutic efficacy and ameliorate the side effects of traditional chemotherapeutic agents.
Wogonin (5,7-dihydroxy-8-methoxyflavone, WG, ), a naturally occurring flavonoid isolated from the root of Scutellaria baicalensis Georgi, is a traditional Chinese herbal medicine widely used in the treatment of inflammatory conditions. WG has attracted considerable attention due to multiple pharmacological benefits (Shieh et al., Citation2000; Chi et al., Citation2001; Guo et al., Citation2007; Park et al., Citation2007; Li-Weber, Citation2009; Lim et al., Citation2010) including anti-inflammatory, anti-oxidant, anti-hepatitis B virus, anticonvulsant and neuro-protective effects, as well as anti-cancer effects. With new data constantly emerging, the promising therapeutic potential of WG as an anti-tumor agent both in vitro and in vivo has been made clear. Mechanistically, WG has been shown to produce anti-cancer effects by increasing cytotoxicity, inhibiting proliferation, inducing apoptosis, inhibiting angiogenesis, reversing drug resistance, inhibiting cell migration and inducing differentiation (Himeji et al., Citation2007; Lu et al., Citation2008; Yang et al., Citation2009; Dong et al., Citation2011; Huang et al., Citation2012; Lee et al., Citation2012).
WG has also had a long and safe record of usage in China, with no or only minor toxicity observed in normal tissues including the epithelium, peripheral blood and myeloid cells. Further, WG treatment has not been associated with any organ toxicity with long-term intravenous administration (Peng et al., Citation2009). Although WG did cause some developmental toxicity in pregnant mice and their pups, no significant malformations were observed and the in vitro potency of the chromosome aberrations was weak (Zhao et al., Citation2011a). Considering all the data, we believed that WG could be a relatively safe drug for use in the clinic.
However, in spite of the wide spectrum of pharmacologically beneficial properties of WG, use of WG as a pharmaceutical is limited due to its poor solubility and a lack of specificity. With the rate of cancer incidence increasing worldwide, there is an urgent need for improved therapeutic activity and selectivity of anti-cancer agents. To our delight, the emergence and development of novel drug delivery systems (Lu et al., Citation2011; Zhao et al., Citation2011b; Yang et al., Citation2012), including liposomes, have provided the opportunity to circumvent the problems with WG related to solubility and targeting, resulting in numerous possible preparations of WG for cancer treatment.
Because targeted drug delivery systems could offer the potential for site-specific cell targeting and the improvement of therapeutic indices, these systems have already become a hotspot for research. Excluding physical means, there are two types of targeting systems: passive targeting and active targeting. Passive targeting, in general, takes advantage of natural anatomical structures or physiological processes. For example, tumor targeting was achieved by the so-called “enhanced permeability and retention effect (EPR)”. Conversely, active targeting utilizes site-directed ligands that bind and interact with specific cellular or tissue epitopes, including circulating targets (Cen et al., Citation2012). Active targeting has been shown to have many benefits, including increasing the selectivity of the interaction between vectors and diseased cells. Active targeting systems also present some sizeable drawbacks (Cajot et al., Citation2012), including variations in plasma residence time due to the density of the ligands used and high toxicity due to non-specific binding. No improvements in survival were observed in vivo in systems utilizing active targeting compared to passive targeting. Moreover, the uptake of ligand-targeted particles was influenced by additional factors including vascular permeability, tissue penetrability, ligand affinity and binding site barriers (Jain & Stylianopoulos, Citation2010; Sawant & Torchilin, Citation2012). Because many of the ligand-targeted particles studied thus far resulted in little or no therapeutic improvement compared to the passive targeting systems, few targeted preparations have progressed into the clinic. Novel ligands should be intensely explored.
The use of glycyrrhetinic acid (GA) as a moiety to target liver cancer is particularly attractive because of the fundamental specificity and selectivity of GA. In 1991, specific GA binding sites were shown to be present on the cell membrane of rat hepatocytes in vitro (Negishi et al., Citation1991) and in 1998, the GA receptor was shown to be present on tumor cells in increased amounts, 1.5–5 times more than in normal tissue (Il’icheva et al., Citation1998). Shortly thereafter, growing interest developed on creating GA-mediated, liver-targeted drug delivery systems. Recently, some GA-modified vectors have been developed (Mao et al., Citation2007; Tian et al., Citation2009, Citation2010a,Citationb; He et al., Citation2010; Huang et al., Citation2010, Citation2011; Tian et al., Citation2012) and show promising results that GA may be used as a novel ligand for targeting hepatocytes. However, in vivo data were rarely obtained, especially for the use of liposomes. These data are necessary to confirm that the increased uptake of the target of the liposomes is due substantially to the increased GA-mediated uptake of the liposomes.
In this study, a GA-modified WG liposomes was developed. This novel liposome was tested alongside with other GA-targeted liposomes based on our previous research (Li et al., Citation2012). Here, we focus mainly on the improvements of the GA-modified WG liposome over the passively targeted liposomes.
Methods
Materials
WG (purity: 99.5%) was supplied from China Pharmaceutical University. (Nanjing, China). Soybean phospholipids (S100) was purchased from Lipoid GmbH (Newark, NJ). Cholesterol was purchased from Huixing Biochemical Reagents Co., Ltd. (Shanghai, China). All the other reagents were analytically pure.
Human hepatocyte (L-02) cells, non-parenchymal (LX-2) cells and human hepatocellular carcinoma (HepG2) cells were purchased from Cell Bank of the Shanghai Institute of Biochemistry and Cell Biology, Chinese Academy of Science (Shanghai, China). Kunming mice weighing 18–22 g and ICR mice were purchased from the Animal Center of the Academy of Military Medical Sciences at China (Certificate No. SCXK 2007-004). This experiment was conducted in accordance with the guidelines issued by the State Food and Drug Administration (SFDA of China). The animals were housed and cared for in accordance with the guidelines established by the National Science Council of the People’s Republic of China.
Preparation of WG liposomes
Liposomes were prepared using the reverse evaporation method. In brief, 4 mg WG was dissolved in 4 ml distilled water by adding co-solvent. Then, a mixture of 200 mg soybean phospholipids and 20 mg cholesterol was dissolved in dichloromethane in a round bottom flask. Next, the WG solution was dropped slowly into the lipid solution and sonicated for 20 min, to obtain a W/O emulsion. 3-Succinyl-30-stearyl glycyrrhetinic acid (18-GA-Suc), synthesized previously (Li & Ke, Citation2011), was dissolved in the emulsion obtained earlier, followed by removal of the organic solvent at 25–30 °C under vacuum evaporation (RE52cs rotary evaporator, Shanghai Yali-Wing Biochemical Instrument Factory, Shanghai, China) until a faint yellow suspension was obtained. The resulting suspension was hydrated with the prescribed dose of buffer, followed by another round of vacuum rotary evaporation. Then, the mixture was sonicated for 5 min at 500 W with an ultrasound probe (JY92 ultrasonic cell crusher, Ningbo Xinzhi Institute of Scientific Instruments, Ningbo, China). Finally, the obtained colloid suspension was extruded through 0.22 μm microporous membranes, giving GA-modified WG liposomes, named GA-WG-Lip. WG liposome with no surface decoration (WG-Lip) was prepared in parallel.
Physicochemical characterization of WG liposomes
HPLC
The content of WG in liposomes was determined using an HPLC system (Shimadzu LC-2010C HT high performance liquid chromatography system, Japan). A Lichrospher C18 column (150 mm × 6 mm i.d., 5 μm, Hanbon, China) was maintained at 35 °C. The mobile phase was methanol and 0.5% acetic acid solution (70:30, V/V) at a flow rate of 1 ml/min with detection at 275 nm. The injection volume was 20 μl.
Determination of entrapment efficiency of WG liposomes
The entrapment efficiency (EE) of WG liposomes was measured by the mini-column centrifugation method. The gel was prepared by leaving Sephadex G50 (10 g) to swell in pH 6.8 citrate buffer (120 ml) at room temperature with occasional shaking for at least 5 h and was then stored at 4 °C. To prepare the mini-columns, absorbent cotton was inserted in the bottom of the barrels of 5.0 cm3 syringes, which were then filled with gel. Excess buffer was removed by centrifugation at 1000 rpm for 3 min.
About 0.2 ml liposome suspension was added drop-wise to the top of the column followed by centrifugation as before and collection of the elutes. Another 0.2 ml citrate buffer was then added into the mini-column and centrifuged. Elutes were again collected. The two elutes were then combined and, following digestion in methanol, the amount of drug entrapped in the liposomes was determined by HPLC. EE was calculated according to the formula: EE = Wliposome/Wtotal × 100%, where Wtotal is the total amount of drug in the liposome suspension and Wliposome is the amount of drug encapsulated in the liposome.
To validate the method, 0.2 ml WG solution and 0.2 ml citrate buffer were separately applied to the mini-column. After centrifuging twice at 1000 rpm for 3 min each, no drug was detected in the elutes, indicating that no free drug would be present when recovering the liposomes.
Size, zeta potential and morphology
The size and zeta potential of the liposomes were measured using a Zetasizer 3000HS (Malvern Instruments Ltd., UK) according to the manufacturer’s protocol. The Dispersion Technology Software was used to analyze the effective diameter. The measurement was performed in triplicate. The vesicle shape of different liposome formulations was evaluated by transmission electron microscopy (H-7000, Hitachi, Japan).
In vitro cellular uptake
Cellular uptake of the WG preparations (WG, WG-Lip, GA-WG-Lip) was studied in three cell types, including hepatocyte (L-02), hepatic non-parenchymal (LX-2) and HepG2 cells.
Cells were grown in RPMI-1640 medium supplemented with 10% heat-inactivated bovine serum (Sijiqing, Hangzhou, China), 100 U/ml benzyl penicillin G and 100 mg/l streptomycin under a humidified atmosphere of 5% CO2 at 37 °C (3111 water-nested-CO2 incubator, USA Thermo Electron Corporation). When in the logarithmic growth phase, the cells were seeded in a 6-well flat-bottomed tissue-culture plate (1 × 105 cells per well) in 2 ml of growth medium and cultured in 5% CO2 at 37 °C. After 24 h, the cells were incubated with WG preparations for 2 h at 37 °C at a final WG concentration of 400 μg/ml. Control experiments were performed by adding medium alone. The cells were then washed three times with 4 °C to eliminate any drug that was not internalized and resuspended in lysis buffer containing 1 mM PMSF for 5 min on ice. The suspension was transferred to a centrifuge tube, then 100 μl acetonitrile was added and gently mixed to completely lyse the cells. The lysates were clarified by centrifugation at 4 °C, 12 000 g for 10 min, then the supernatant was collected and analyzed by HPLC to determine the WG content. The protein concentration was measured by a BCA protein assay kit (Novagen Inc., Darmstadt, Germany) with bovine serum albumin as a standard. The concentrations were expressed as ng WG bound per mg cellular protein.
In vitro cytotoxicity test
Sensitivity of HepG2 to WG preparations (WG, WG-Lip, GA-WG-Lip) was evaluated employing 3-(4,5-dimethylthiazol-2-yl)-2,5-diphenyltetrazolium bromide (MTT)-based cytotoxicity assay.
HepG2 cells were cultured as described earlier, then were seeded in a 96-well plate (1.0 × 105 cells per well) followed by treatment with increasing concentration of WG preparations, with untreated medium as a control. The cells were cultured for 24 h and then washed twice with PBS. After that, 20 μl MTT solution (5 mg/ml in PBS) was added into each well and cells were incubated for an additional 4 h at 37 °C. The supernatant was aspirated off, and 100 μl DMSO was added into each well to dissolve any precipitate present. The optical ratio at 570 nm was measured using the Enzyme Immunoassay Instrument (DJ-3200, Huadong Electron Tube Company, China), as an indicator of cell viability. The cell inhibitory ratio was calculated by the following formula: Inhibitory ratio (%) = (1 − average absorbance of treated group/average absorbance of control group) × 100%.
In vivo bio-distribution
The in vivo bio-distribution of WG preparations was investigated in tumor-bearing mice. Tumors were injected with HepG2 cells in their right armpit. Briefly, the animals were divided into three groups of nine mice each. WG solution, WG-Lip or GA-WG-Lip containing equivalent does of WG (20 mg kg−1) were injected to the different groups of mice via tail-vein injection. The mice were subsequently sacrificed at 15-, 90- and 180-min post-injection by cervical dislocation, following collection of blood samples from the eyeballs. Following sacrifice, the major organs including heart, liver, spleen, lung and kidney were collected, frozen in liquid nitrogen and stored at −20 °C for further analysis. To determine the amount of drug accumulation, organs were weighed and homogenized in 0.5 ml saline solution per 100 mg tissue. About 0.01 M H3PO4 solution and 150 µl acetonitrile were added to the homogenate, incubated for 10 min and vortexed for 5 min. After centrifugation at 12 000 rpm for 10 min, the supernatant was determined by HPLC. About 0.1 ml of plasma was added in with the 0.01 M H3PO4 and 150 μl acetonitrile solution, then vortexed for 5 min, followed by centrifugation at 12 000 rpm for 10 min. The supernatant was analyzed by HPLC, demonstrated to be a reliable technique in each tissue including blood.
In vivo tumor growth inhibition study
The in vivo anti-tumor efficacy of WG preparations was evaluated with the animal tumor models set up by inoculation with HepG2 cells. Based on the data obtained in our previous study in BALB/c nude mice (Li et al., Citation2012), the ICR mice used in this study (18–22 g, 50% male and 50% female) were selected and injected subcutaneously in the armpit of the right anterior limb with 0.2 ml of cell suspension containing HepG2. After 24 h, mice were weighed and randomly divided into three groups (n = 10, each group): (1) WG-Lip, (2) GA-WG-Lip and (3) negative control group (saline). The control saline or liposomes with the drug dose of 10 mg kg−1 were administered once every 3 days via tail-vein injection continuously 3 times, and weighed every other day. On the third day following treatment, all of the mice were weighed then sacrificed by cervical dislocation, followed by separation and measurement of the tumor block. The anti-tumor efficacies of each group were evaluated by calculating the tumor inhibition rate (TIR) via the following formula:
Statistics
Results were expressed as the mean ± SD. Analysis of variance (ANOVA) was used to test the statistical significance of differences among groups. Statistical significance was evaluated by using Student’s t-test for single or multiple comparisons between experimental groups, respectively.
Results
Characterization of WG liposomes
The average sizes of WG-Lip and GA-WG-Lip are displayed in . Both liposomes had an average size of ∼90 nm. The liposomes were negatively charged with zeta potentials of −16.2 and −16.8 mV, respectively, and the EE values were both >92%. shows the regular spherical morphology of the liposomes. No significant changes were observed in any characteristic following the addition of 18-GA-Suc to the liposomes.
Figure 2. Transmission electron photomicrographs of WG liposomes. (A) WG liposome with no surface decoration (WG-Lip) and (B) GA-modified WG liposome (GA-WG-Lip).
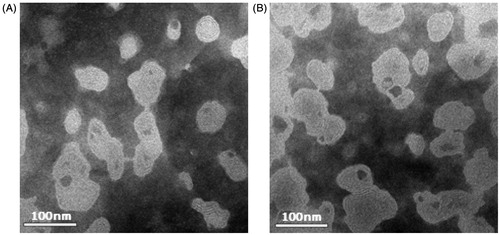
Table 1. The particle size and Zeta potential of the liposomes (n = 3, ± SD).
Cellular uptake in vitro
Three cell types, hepatocyte (L-02), hepatic non-parenchymal (LX-2) and HepG2 cells, were selected to investigate the uptake of WG preparations, which all contained 400 µg ml−1 WG. All of the groups were incubated in parallel for 2 h, then the cellular uptake indices were tested. The results are displayed in . Among the three types of WG preparations, the uptake of liposomes was higher than the uptake of WG alone in all three cell types.
Figure 3. Cellular uptake of WG preparations in L02, LX2 and HepG2 cells. Each group was contain 400 µg ml−1 of WG, which was parallely incubated for 2 h. Each data represent the mean ± SD (n = 3). *Significant difference between the WG solution and WG liposomes in the groups of L-02 and HepG2 (p < 0.05). ΔSignificant difference between groups of L-02 and HepG2 with the treatment of WG-Lip and GA-WG-Lip (p < 0.05).
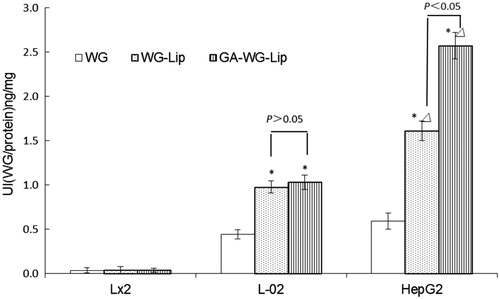
The amount of uptake of the three WG preparations in LX-2 cells was much lower than in other cell types. The L-02 cells took up a much greater amount of WG compared to the LX-2 cells (p < 0.05), implying that hepatocytes can better take up WG preparations. Further, the two WG liposomes were superior to the WG solution (p < 0.05), showing that encapsulation of WG in a liposome benefits the uptake of WG. There was no difference in the uptake of WG-Lip and GA-WG-Lip in L-02 cells (p > 0.05). The uptake of WG liposomes in HepG2 cells was even greater than the uptake in L-02 cells (p < 0.05). This phenomenon may be due to the different nature of HepG2 and L-02 cells. This cellular selectivity could be advantageous for the use of WG liposomes in cancer therapy.
Glycyrrhetinic acid-modified WG liposomes showed the highest cellular uptake, at a rate almost 1.6 times that of unmodified WG-Lip on HepG2 cells. The uptake ratio of GA-WG-Lip in HepG2 cells was 2.5 times that in L-02 cells, showing that the GA-modification can distinguish hepatic carcinoma cells from normal hepatocytes. Similar results were also reported by Tian in self-assembly sulfate chitosan nanoparticles functionalized with GA (Tian et al., Citation2010b). These results may indicate that more GA-specific binding sites are present on HepG2 cells than there are on L-02 cells, resulting in increased endocytosis and therefore increased WG uptake.
Cytotoxicity test
First, the cytotoxicity of GA-modified blank liposomes was assessed as a control. The concentration of blank liposomes was equal to the concentrations used in the cytotoxicity assay of drug-loaded liposomes. The blank liposomes were incubated with HepG2 cells for 24 h. Over the incubation period, >95% of the cells survived, indicating that blank liposomes had no significant cytotoxicity against HepG2 cells.
Results of the cytotoxicity assays with WG preparations are displayed in . As shown, tumor inhibitory efficacy of all WG preparations increased as the WG concentration increased. Meanwhile, the inhibitory efficacy of both liposomes was distinctly higher than that of WG solution (*p < 0.01) when the WG concentration was beyond 1 μg ml−1. The GA-modified liposome performed better than the unmodified liposome, especially at low doses (Δp < 0.01). However, at higher doses, there is no significant difference between the two liposomes. The IC50 value of each preparation, according to the cytotoxicity, was 16.248, 3.344 and 2.292 μg ml−1, respectively, which reveals that the HepG2 cell inhibitory efficacy of GA-WG-Lip was 1.46 times higher than that of WG-Lip.
In vivo bio-distribution
represents the tissue and plasma distribution results in samples taken 15, 90 and 180 min after injection of WG preparations into the tail-vein of mice. The WG solution was present in every tissue, including heart, liver, spleen, lung, kidney and plasma, and was present in particularly high levels in the kidney, where the WG would be rapidly excreted. WG-Lip was passively targeted to both the tumor and liver and was present at low levels in the heart, lung and kidney. In contrast, GA-WG-Lip rapidly accumulated in tumor, liver and spleen, with especially high levels in the tumor and liver just 15 min after injection. The uptake of GA-WG-Lip in the tumor was the highest among all of the excised tissues and was also much higher overall than the uptake of WG and WG-Lip. As time went on, the uptake of GA-WG-Lip in the liver remained the highest, but uptake gradually decreased in other tissues, including the tumor. We reasoned that the ectopic tumor may have been recognized as foreign by the mice. Nevertheless, WG from the GA-WG-Lip group was concentrated in the tumor at higher amounts than in other tissues except for liver.
In vivo anti-tumor efficacy
As shown in , both liposome groups resulted in significant growth inhibition of Heps-transplanted solid tumors compared with the control group (p < 0.01). The tumor inhibitory ratios (TIRs) of WG-Lip and GA-WG-Lip were 31.34% and 53.73%, respectively. The TIR of GA-WG-Lip was significantly better than that of WG-Lip (p < 0.05). All of these results indicate that the GA-modified WG liposomes had better anti-tumor effects in vivo than unmodified liposomes. It can be further inferred that liposomes modified with 18-GA-Suc mediated cellular uptake via the GA-specific binding sites on the surface of hepatic cells, which improved liver targeting and consequently enhanced therapeutic outcomes.
Table 2. The effect of WG-Lip and GA-WG-Lip on tumor model of Heps in mice (n = 10, ± SD).
Discussion
In this report, GA-modified WG liposome was developed and compared to the passively targeted WG-Lip. Their average size, zeta potential and EE were similar, indicating that the addition of 18-GA-Suc had no significant effect on the physical properties of the liposomes. The bio-distribution of the vectors could be affected by the particle size, so it was critical to show that size was not playing a role in the effects of the liposomes.
Three types of cells were selected to investigate the uptake of WG preparations, aimed to demonstrate the increased uptake of GA-WG-Lip in vitro because GA was employed on the surface of the liposome. It was indeed noted that GA-modified WG liposomes showed the greatest uptake, especially in HepG2 cells. It was speculated that more GA-specific binding sites existed on HepG2 cells than L-02 cells, resulting in increased endocytosis and therefore increased uptake.
Prior studies found that specific GA binding sites exist on the cellular membranes of rat hepatocytes in vitro. Other studies indicated that GA-modified nanocarriers had increased affinity for human hepatic or hepatic carcinoma cells, but no studies specifically looked at the difference in GA binding sites between hepatic carcinoma cells and normal liver cells. The present study displays the variation of GA binding sites on different liver cells, indicating that GA modification is a good candidate for the targeting of hepatic carcinoma cells and that this mechanism deserves further study.
Our hypothesis was further supported by the sequenced results of the in vitro cytotoxicity test. The IC50 value of GA-WG-Lip was 1.46 times higher than that of WG-Lip. Hence, GA played an important role in the efficacy of the designed liposomes. These results allowed us to establish that GA-modified liposomes could act as vectors able to carry WG into targeted cells in vitro. However, these experiments did not address whether GA-WG-Lip could act as a preferential method of delivery to distal tumor sites in vivo, so the bio-distribution and anti-cancer effects were studied in vivo in the present study.
GA-WG-Lip was especially accumulated in tumor and liver just 15 min following tail-vein injection. The uptake of GA-WG-Lip in the tumor was originally the highest, but decreased to levels lower than that in the liver over time, possibly due to self-clearance by the immune system. Nevertheless, WG was concentrated in the tumor and maintained for longer than in other tissues in GA-WG-Lip group, with the exception of normal liver tissue. WG encapsulated in unmodified liposomes was also relatively high, likely due to passive targeting to the tumor site or liver tissues. Together, these data led us to believe that WG encapsulated in GA-modified liposomes would be accumulated in high concentrations in a long-lasting manner in orthotopic-implantation tumors.
Evidence suggested that GA-modified liposomes were not only targeted to the liver, but further, were taken up by hepatic parenchymal cells with the most abundant GA receptors. Increased uptake of GA-WG-Lip by hepatic cells due to more abundant GA receptors allows specific targeting of WG to liver tumors, consequently enhancing therapeutic outcomes.
Conclusions
In this study, GA-modified WG liposomes were developed. The GA-WG-Lip can actively and specifically target the liver, resulting in decreased tumor weight. An improvement in bio-distribution, tumor accumulation and therapeutic efficacy due to the increased receptor-mediated uptake of liposomes was observed in liver cells. WG actively modified liposomes, and these produced better anti-cancer effects in vivo than the passively targeted liposomes. The contribution of GA in the targeted delivery of liposomes containing WG to the liver provides a promising liver-targeted moiety for achieving enhanced liver cancer therapy.
Declaration of interest
The authors report no conflict of interest in this research. This study was financially supported by the National Natural Science Foundation of China (Grant No. 81073055) and the Project Program of State Key Laboratory of Natural Medicines, China Pharmaceutical University (No. JKGP201102).
Acknowledgements
Thanks to the Jiangsu Key Laboratory of Carcinogenesis and Intervention (China Pharmaceutical University) for assistance with cell and animal tests.
References
- Cajot S, Butsele KV, Paillard A, et al. (2012). Smart nanocarriers for pH-triggered targeting and release of hydrophobic drugs. Acta Biomater 8:4215–23
- Cen P, Ni XL, Yang JX, et al. (2012). Circulating tumor cells in the diagnosis and management of pancreatic cancer. Biochimica et Biophysica Acta 1826:350–6
- Chi YS, Cheon BS, Kim HP. (2001). Effect of wogonin, a plant flavone from Scutellaria radix, on the suppression of cyclooxygenase-2 and the induction of inducible nitric oxide synthase in lipopolysaccharide-treated RAW 264.7 cells. Biochem Pharmacol 61:1195–203
- Dong P, Zhang Y, Gu J, et al. (2011). Wogonin, an active ingredient of Chinese herb medicine Scutellaria baicalensis, inhibits the mobility and invasion of human gallbladder carcinoma GBC-SD cells by inducing the expression of maspin. J Ethnopharmaco 137:1373–80
- Guo Q, Zhao L, You Q, et al. (2007). Anti-hepatitis B virus activity of wogonin in vitro and in vivo. Antivir Res 74:16–24
- He ZY, Zheng X, Wu XH, et al. (2010). Development of glycyrrhetinic acid-modified stealth cationic liposomes for gene delivery. Int J Pharm 397:147–54
- Himeji M, Ohtsuki T, Fukazawa H, et al. (2007). Difference of growth-inhibitory effect of Scutellaria baicalensis-producing flavonoid wogonin among human cancer cells and normal diploid cell. Cancer Lett 245:269–74
- Huang KF, Zhang GD, Huang YQ, et al. (2012). Wogonin induces apoptosis and down-regulates survivin in human breast cancer MCF-7 cells by modulating PI3K-AKT pathway. Int Immunopharmacol 12:334–41
- Huang W, Wang W, Wang P, et al. (2010). Glycyrrhetinic acid-modified poly (ethyleneglycol) – b – poly (γ – benzyl L-glutamate) micelles for liver targeting therapy. Acta Biomater 6:3927–35
- Huang W, Wang W, Wang P, et al. (2011). Glycyrrhetinic acid-functionalized degradable micelles as liver-targeted drug carrier. J Mater Sci Mater Med 22:853–63
- Il’icheva TN, Proniaeva TR, Smetannikov AA, et al. (1998). Content of progesterone, glucocorticoid and glycyrrhizic acid receptors in normal and tumoral human breast tissue. Vopr Onkol 44:390–4
- Jain RK, Stylianopoulos T. (2010). Delivering nanomedicine to solid tumors. Nat Rev Clin Oncol 7:653–64
- Lee DH, Lee TH, Jung CH, et al. (2012). Wogonin induces apoptosis by activating the AMPK and p53 signaling pathways in human glioblastoma cells. Cell Signal 24:2216–25
- Li J, Ke X. (2011). Preparation of docetaxel liposomes surface-modified with glycyrrhetinic acid and its in vitro anti-tumor effect. Pharm Clin Res 19:207–10
- Li J, Xu H, Ke X, et al. (2012). The anti-tumor performance of docetaxel liposomes surface-modified with glycyrrhetinic acid. J Drug Target 20:467–73
- Lim JS, Yoo M, Kwon HJ, et al. (2010). Wogonin induces differentiation and neurite outgrowth of neural precursor cells. Biochem Bioph Res Co 402:42–7
- Li-Weber M. (2009). New therapeutic aspects of flavones: the anticancer properties of Scutellaria and its main active constituents Wogonin, Baicalein and Baicalin. Cancer Treat Rev 35:57–68
- Lu CT, Zhao YZ, Wu Y, et al. (2011). Experiment on enhancing antitumor effect of intravenous epirubicin hydrochloride by acoustic cavitation in situ combined with phospholipid-based microbubbles. Cancer Chemother Pharmacol 68:343–8
- Lu N, Gao Y, Ling Y, et al. (2008). Wogonin suppresses tumor growth in vivo and VEGF-induced angiogenesis through inhibiting tyrosine phosphorylation of VEGFR2. Life Sci 82:956–63
- Mao SJ, Bi YQ, Jin H, et al. (2007). Preparation, characterization and uptake by primary cultured rat hepatocytes of liposomes surface-modified with glycyrrhetinic acid. Pharmazie 62:614–9
- Negishi M, Irie A, Nagata N, et al. (1991). Specific binding of glycyrrhetinic acid to the rat liver membrane. Biochim Biophys Acta 1066:77–82
- Park HG, Yoon SY, Choi JY, et al. (2007). Anticonvulsant effect of wogonin isolated from Scutellaria baicalensis. Eur J Pharmacol 574:112–9
- Peng J, Qi Q, You Q, et al. (2009). Subchronic toxicity and plasma pharmacokinetic studies on wogonin, a natural flavonoid, in Beagle dogs. J Ethnopharmacol 124:257–62
- Sawant RR, Torchilin VP. (2012). Challenges in development of targeted liposomal therapeutics. AAPS J 14:303–11
- Shieh DE, Liu LT, Lin CC. (2000). Antioxidant and free radical scavenging effects of baicalein, baicalin and wogonin. Anticancer Res 20:2861–5
- Tian Q, Wang W, He XT, et al. (2009). Glycyrrhetinic acid-modified nanoparticles for drug delivery: preparation and characterization. Chin Sci Bull 54:3121–6
- Tian Q, Wang X, Wang W, et al. (2010a). Insight into glycyrrhetinic acid: the role of the hydroxyl group on liver targeting. Int J Pharm 400:153–7
- Tian Q, Zhang CN, Wang XH, et al. (2010b). Glycyrrhetinic acidmodified chitosan/poly(ethylene glycol) nanoparticles for livertargeted delivery. Biomaterials 31:4748–56
- Tian Q, Wang XH, Wang W, et al. (2012). Self-assembly and liver targeting of sulfate chitosan nanoparticles functionalized with glycyrrhetinic acid. Nanomed Nanotechnol 8:870–9
- Yang L, You Q, Yang Y, Guo Q. (2009). Research advances in wogonin’s anti-tumor effects. J Chin Pharm Univ 40:576–9
- Yang XY, Li YX, Li M, et al. (2012). Hyaluronic acid-coated nanostructured lipid carriers for targeting paclitaxel to cancer. Cancer Lett 334:338–45
- Zhao L, Chen Z, Zhao Q, et al. (2011a). Developmental toxicity and genotoxicity studies of wogonin. Regul Toxicol Pharm 60:212–7
- Zhao YZ, Sun CZ, Lu CT, et al. (2011b). Characterization and anti-tumor activity of chemical conjugation of doxorubicin in polymeric micelles (DOX-P) in vitro. Cancer Lett 311:187–94