Abstract
The work was to prepare and characterize a responsive drug delivery system built of chitosan-g-poly (N-isopropylacrylamide) (CTS-g-PNIPAAm) nanogels and to evaluate the effects of CTS molecular weight (Mw) on the loading and in vitro release of insoluble drug 10-hydroxycamptothecine (HCPT). The CTS-g-PNIPAAm copolymers were synthesized by radical polymerization. The Mw and physical chemistry properties such as diameter, second virial coefficient of grafted PNIPAAm were investigated by dynamic and static laser light scattering method. A series of cross-linked CTS-g-PNIPAAm nanogels were prepared with N, N′-methylenebisacrylamide initially added as a cross-linker. The thermal and pH-sensitive features of cross-linked CTS-g-PNIPAAm nanogels were studied by determining the variance of transmittance, changeable size and reversed zeta potential. The critical aggregation concentrations (CAC) of resultant nanogels decreased from 0.045 to 0.036 mg/mL with CTS Mw increased from 50 kDa to 700 kDa. The loading efficiency of the HCPT encapsulated into CTS-g-PNIPAAm nanogels increased in parallel with CTS Mw, while the cumulative release percentage of HCPT-loaded nanogels decreased with CTS Mw increasing at both 25 °C and 37 °C. Fitting results of HCPT release data to different mathematical models suggested a diffusion-controlled mechanism at 25 °C. However, the release behaviors were dominated by combined effects of polymer erosion and osmotic pressure driven at 37 °C. The cytotoxicity study of the CTS-g-PNIPAAm nanogels against hepatic L02 cells indicated that the resultant nanogels did not exhibit apparent cytotoxicity.
Introduction
Natural polysaccharides, due to their outstanding merits (highly stable, safe, hydrophilic, biodegradable and easily modified chemically and biochemically), have received much attention in the field of drug delivery systems especially in the preparation of nanometeric carriers. Through the careful selection and blending of structurally and/or functionally diverse block copolymers based on natural polysaccharides, a population of novel and multi-functional nanoparticles bearing desirable characteristics can be easily fabricated without the need for elaborate synthetic scheme (Liu et al., Citation2008).
Chitosan (CTS), a natural based-polymer which is obtained by alkaline deacetylation of chitin, is nontoxic, biocompatible and biodegradable (Varshosaz, Citation2007). CTS is soluble in weak acid and reported to possess pH sensitivity owing to protonation and de-protonation of amine group. CTS can be modified by graft copolymerization via its amino or hydroxyl groups, to achieve versatile molecular designs (Mourya & Inamdar, Citation2008; Park et al., Citation2010a; Dash et al., Citation2011). Mw and degree of deacetylation (D.D.) of CTS are generally recognized as key factors to influence the properties of CTS-based materials. Zhang et al. observed the effect of CTS Mw on the oleoyl-CTS nanoparticles as carriers for doxorubicin (Zhang et al., Citation2010), and the result showed that the loading efficiency and the DOX release rate increased as CTS Mw decreased. Zhou et al. (Citation2008) observed the effect of Mw and D.D. of CTS on the preparation and characteristics of CTS thermo-sensitive hydrogels as a delivery system, the results presented that the hydrogels became more compact and regular when increasing concentration and Mw of CTS; while the release rate for both Adriamycin and 6-mercaptopurine from CS-αβ-GP hydrogels decreased with the increase of CTS Mw.
Poly(N-isopropylacrylamide)(PNIPAAm) is one of the most popular thermo-responsive polymers which shows dramatic and reversible phase transition behavior in water with the low critical solution temperature (LCST) at around 32 °C (Schild, Citation1992). PNIPAAm has been widely investigated for possible applications in thermal responsive drug delivery (Wei et al., Citation2009). Rejinold et al. managed to conjugate fibrinogen with PNIPAAm nanogels to co-delivery 5-FU and Megestrol acetate. It was observed that the accumulative release of both drugs were greater when the temperature was above the LCST due to the weaker hydrogen bonding between polymer chains facilitating more open structures of drug-loading nanogels. In addition, the products showed enhanced cellular uptake and maximum apoptosis of breast cancer cells compared with little uptake and minimum apoptosis of normal cells (Rejinold et al., Citation2014). Some authors reported the possibilities of grafting PNIPAAm to CTS to prepare biocompatible, thermal and pH-sensitive drug carriers with similar or different structures, such as grafting copolymer (Chen et al., Citation2013), grafting-cross-linking copolymers (Rejinold et al., Citation2011), nanoparticles (Li et al., Citation2009), etc. Rejinold et al. prepared thermo-responsive CTS-graft-Poly(N-isopropylacrylamide) nanoparticles to encapsulate 5-Fluorouracil; it was observed that 5-Fluorouracil release was higher in the case of acidic pH at above LCST than physiological pH at below LCST (Rejinold et al., Citation2013). Obviously, Mw of CTS, D.D. of CTS and Mw of grafted PNIPAAm are important factors that influence size, stability, in vitro release and therefore in vivo behavior of resultant nanocarriers. However, most of the authors concerned more about the characterization of resultant nanoparticles or copolymers by IR, LCST, DLS, SEM or TEM, DSC, drug loading and in vitro release behavior, cytotoxicity, cellular uptake, etc. Little attention was received on the polymer itself, such as the effects of CTS Mw on the characteristics, loading efficiency and release behavior of CTS-g-PNIPAAm nanogels. The appropriate Mw of PNIPAAm grafted to CTS was still unclear as far as we know. Furthermore, the thermal-responsive releasing behavior and possible mechanism from drug-loading CTS-g-PNIPAAm nanogels are rarely reported.
The present study aimed to explore the appropriate Mw range of CTS to prepare thermal and pH-sensitive CTS-g-PNIPAAm nanogels. The effects of CTS Mw on the dual-sensitive properties were observed and analyzed. The Mw and physical chemistry properties such as diameter (D), second virial coefficient (A2) of PNIPAAm grafting to CTS were systematically investigated by Dynamic and Static Laser Light Scattering (SLLS) methods. HCPT was encapsulated into a series of CTS-g-PNIPAAm nanogels prepared from different Mw CTS, and in vitro cumulative release profiles were studied at 25 °C and 37 °C. Moreover, the release profiles were fitted by several classical mathematical models to discuss possible release mechanisms. Besides, the cytotoxicity study of the resultant nanogels against hepatic L02 cells was conducted by MTT method.
Materials and methods
Materials and reagents
CTS (D.D. > 90%, Mw approximately 50 kDa, 75 kDa, 200 kDa and 700 kDa) were obtained from Zhejiang Jinke Biochemical (China). N, N′-methylenebisacrylamide (MBA) was purchased from Sigma-Aldrich. NIPAAm was purchased from International Laboratory (USA, 99%) and recrystallized in n-hexane. Ammonium persulfate (APS) was obtained from Product of ES. All the other chemicals were of analytical grade or above and used as received without further purification.
Preparation of CTS-g-PNIPAAm copolymers
Specified amount of CTS (0.2 g) with Mw 200 kDa was dissolved in 20 mL 4 %(v/v) acetic acid solution containing NIPAAm (0.4 g, 0.8 g and 1.2 g, respectively). The solutions were purged with nitrogen and heated to 80 °C for 30 min. Then, APS was dissolved in 5 mL of distilled water and purged into the solutions. After 3.5–4 h of reaction, solutions containing CTS-g-PNIPAAm copolymers and PNIPAAm homopolymers were obtained. The completion of the reaction was monitored by detecting the disappearance of NIPAAm (developer with petroleum ether:ethyl acetate = 3:1(v/v) and colored in Iodine. The dispersions were further purified by placing them into a dialysis bag with a 14 000 Da Mw cut-off and dialyzed against 5 L of deionized water for 48 h at room temperature (R.T.) to remove small molecules and unreacted monomers. The solutions were then lyophilized and stored at desiccator before further study.
The grafting ratio (GR) and efficiency (GE) were calculated by the gravimetric analysis using the following Equation: GR = (W1−Wc)/Wc and GE = (W2−Wc)/Wc where, Wc was the initial weight of CTS, W1 was the primary weight of the products and W2 was the final weight of purified products. The shrinking rate (SR, %), intended to reflect the thermo-sensitivity of products, was calculated from following equation: SR = (size at 25 °C−size at 37 °C)/size at 25 °C × 100%.
The determination of Mw of PNIPAAm
A series of CTS-g-PNIPAAm copolymers were hydrolyzed by 12 M HCl(aq) for 4 h at 40 °C to degrade the CTS chain and obtain PNIPAAm (Chuang et al., Citation2010). Before the SLLS, the increscent of the refractive index dn/dCp of PNIPAAm was determined by the double bean differential refraction meter firstly as about 0.171 mL/g. Briefly, each PNIPAAm was dissolved in methanol at four concentrations (0.5 mg/mL, 1 mg/mL, 2 mg/mL and 4 mg/mL) and filtered by 0.22 μm microporous membrane for the SLLS experiment. Based on theory of light scattering, KCp/R (θ) = 1/Mw+2A2Cp. Where is the optical contrast in which n is the refractive index of the solvent, λ0 is the incident wavelength. Nav is the Avogadro’s constant, Cp is polymer concentration (g/mL), and R (θ) is the Rayleigh ratio at the measurement angle. R(θ) was determined at angel 90°(BI-APD, Brookhaven, USA). KCp/R (θ) against polymer concentrations (Cp) was plotted. The Mw was converted from the intercept of the Debye plots. A2 was obtained from the slope of Debye plots (Bajaj et al., Citation2007). Mean square rotating diameter (Rg) was obtained from the equipment directly.
Preparations of cross-linked CTS-g-PNIPAAm and PNIPAAm nanogels
The preparation procedure of cross-linked CTS-g-PNIPAAm nanogels was similar to that of copolymers with initially adding 2.5% (g/g, versus NIPAAm) MBA into the solutions (Li et al., Citation2009). CTS with Mw 50 kDa, 75 kDa, 200 kDa and 700 kDa were employed.
PNIPAAm nanogels were synthesized by free-radical emulsion polymerization of NIPAAm in aqueous solutions by employing APS (0.05 M) and TEMED (0.32 M) as the redox initiator system. The solution was stirred in a thermostatic water bath at 30 °C, and the polymerization was conducted for 24 h. The post-processing of the products was the same as CTS-g-PNIPAAm copolymers as mentioned in the section “Preparation of CTS-g-PNIPAAm copolymers”.
Temperature-sensitive properties of CTS-g-PNIPAAm nanogels
The freeze-dried products were reconstituted into aqueous solution (1.5 mg/mL) and divided into two parts. Part 1 was to measure the size and zeta potential at 25 °C and 37 °C by Dynamic Laser Light Scattering (DLS, Brookhaven instrument corporation, model: BI-APD) with a laser light wavelength of 532 nm at a 90° scattering angle. The Z-average hydrodynamic diameters of the particles were intensity-based mean diameters and given by the instrument directly calculated from correlation equation. Part 2 of the solutions were subjected to observe transmittance changes at 500 nm at designed temperature (25, 32, 32.5, 33, 33.5, 34, 34.5, 35, 37 and 40 °C) by UV–vis spectrometer (Thermo scientific varioskan flash spectral scanning multimode reader). The samples were allowed to equilibrate for 10 min at each temperature before the measurements were taken. In this study, the volume phase transition temperature (VPTT) of the resultant solution was defined as transmittance at 500 nm exhibiting inflexion due to PNIPAAm phase transition with the temperature (Howe et al., Citation2011).
pH-sensitive properties of CTS-g-PNIPAAm nanogels
The pH-sensitivity of resultant products was evaluated by detecting the size variances at phosphate buffer solutions (PBS) with different pH (i.e. 5.0, 6.0, 6.8, 7.4 and 8.0), and the size of the nanogels were detected by DLS method.
Critical aggregation concentration (CAC) of CTS-g-PNIPAAm nanogels
The CAC was determined by fluorescence probe method (Liu et al., Citation2009; Ghosh et al., Citation2011; Vasilescu et al., Citation2011). Briefly, the nanogels with concentrations ranged from 1.5 × 10−3 to 1.5 mg/mL, were added into the volumetric flasks containing pyrene with final concentration of 6 × 10−7 M. Then, the solutions were sonicated for 30 min followed by incubation at 40 °C for 1 h and kept from light overnight at R.T. Fluorescence spectra were recorded with an RF-5301 PC fluorescence spectrophotometer (Shimadzu, Tokyo, Japan) with the emission wavelength at 390 nm. The slit widths for emission and excitation were set at 3.0 and 1.5 nm, respectively. The peak height intensity ratio (I3/I1) of the third peak (I3 at 338 nm) to the first peak (I1 at 333 nm) against concentrations was plotted.
Drug loading and releasing profiles of CTS-g-PNIPAAm nanogels
Preparation of HCPT-loading nanogels
HCPT-loading nanogels were prepared by emulsion-evaporation method. Briefly, the freeze-drying products were dissolved in deionized water with a final concentration of 1.5 mg/mL. The drug was dissolved in chloroform/methanol (1/1, v/v) mixture and gently dipped into polymer solution under stirring at 30 °C for 2 h to remove organic solvents. The resultant solution was centrifuged at 2000 rpm for 20 min to remove free drug. The drug-loading content of the HCPT nanogels was determined by HPLC method. SHIMAZU HPLC system was equipped with a 5 μm, ODS 250*4.6 mm column. The mobile phase consisting of methanol/water (55:45, v/v) and the column temperature of 30 °C were adopted. The flow rate was 0.8 mL/min, and the detection wavelength was 237 nm. Before the detection, the samples were acidized by 1%(v/v) acetic acid and stayed overnight in darkness. The HCPT-loading contents were calculated with the following Equation: Loading content = HCPT encapsulating in the nanogels/weight of blank nanogels × 100%.
The hydrodynamic diameters of blank and HCPT-loading nanogels were observed by DLS, and meantime the representative morphologies of blank and HCPT-loading nanogels prepared from CTS Mw 200 kDa were also shown in pictures observed by Transmission Electron Microscope (TEM, Hitachi TEM, HC-1, 80 KV).
In vitro HCPT release at 25 °C and 37 °C
HCPT release behaviors were studied in vitro by a dialysis method in PBS (pH 7.4, 0.1 M). Briefly, HCPT nanogels with specific amount were placed in a dialysis bag (MWCO: 14 000) and dialyzed against the release media at 25 °C and 37 °C in a water-bath shaker at 100 rpm. At predetermined time intervals, the 2 mL release media was removed and replaced with the same amount of fresh release media. The amount of HCPT released from the nanogels was determined by HPLC method as above described. The experiments were performed under sinking conditions.
Cross-linked CTS-g-PNIPAAm nanogels cytotoxicity evaluation
Cell culture
Human hepatic cell line L02 was cultured in a RPMI 1640 medium with 2 mM L-glutamine, 5% penicillin–streptomycin, and 10% fetal bovine serum in a humidified incubator at 37 °C and with a 5% CO2 atmosphere to evaluate the in vitro cytotoxicity of blank nanogels.
Briefly, the cells were plated at a density of 1 × 104 cells/well in a 96-well plate and cultured for 24 h and then exposed to blank nanogels for another 72 h. After nanogels exposure at various concentrations, the cells were washed three times with PBS. Then 180 μL of RPMI 1640 medium without serum and 20 μL of MTT solution (5 mg/mL) were then added to each well. The plates were incubated for an additional 4 h and then the medium was discarded. 200 μL of DMSO was added to each well, and the solution was gently mixed to dissolve the reacted dye. The absorbance of each well was read on a microplate reader (Thermo scientific varioskan flash spectral scanning multimode reader) at a test wavelength of 490 nm.
Results and discussion
Preparation of CTS-g-PNIPAAm grafted copolymers
The structures of products have been identified by IR and NMR in our former studies (Wang et al., Citation2013). As shown in , GR, GE and SR increased accordingly with the feeding amount of NIPAAm. However, we noticed that with continuing increase of NIPAAm, GE and SR kept a much slower increasing way probably attributed to the byproducts produced by homopolymerization. Our results were in good agreement with former studies. To further investigate the effect of NIPAAm feeding on the Mw of grafted PNIPAAm and resultant thermal-sensitive features of products, the Mw of grafted PNIPAAm was determined soon afterwards.
Table 1. Summary of GR, SR and Mw of PNIPAAm prepared from different monomer feeding.
The Mw of PNIPAAm chains
As reported, CTS could be degraded by strong acid such as hydrochloric acid into small molecules (Chuang et al., Citation2010). So in this study, CTS-g-PNIPAAm grafted copolymers were first processed with hydrochloric acid to obtain a series of linear PNIPAAm polymers. Typical Debye plots are shown in . Mw, Rg and A2 of these linear copolymers were obtained from the Debye plots determined by the concentration dependence of scattering intensity with Cp of 0.5, 1, 2 and 4 mg/mL, as are listed in . The Mw of PNIPAAm increased accordingly with the feeding amount of NIPAAm (Li et al., Citation2011). Meantime, the A2 of copolymers presented an increasing trend. As far as we know A2 is a property describing the interaction strength between the polymers and the solvent or appropriate dispersion medium (Gibson, Citation1987). Samples where A2 > 0, the particles “like” the solvent more than itself, will tend to stay as a stable solution. When A2 < 0, the particle “likes” itself more than the solvent, and therefore may aggregate. When A2 = 0, the particle–solvent interaction strength is equivalent to the molecule–molecule interaction strength, the solvent can then be described as being a θ solvent. In this study, PNIPAAm with a lower Mw and shorter chain length in methanol showed A2 as a negative value (−0.00076), which indicated the very weak interaction between PNIPAAm and solvent, and the polymer like the solvent more than itself; while PNIPAAm with higher Mw and longer chain showed A2 as increasing positive values (from 0.00042 to 0.000692), which indicated the polymers became more solvophilic. Therefore, conclusion could be made that NIPAAm feeding weight at the medium level (0.8 g) could make a good balance with CTS main backbone and the products could show stable nanogel-like structures from collective SR and corresponding Mw data. The corresponding Mw of grafting PNIPAAm was about 484842.3. The polymerization degree of PNIPAAm was calculated as 4290.
Preparations of cross-linked CTS-g-PNIPAAm and PNIPAAm nanogels
As listed in , GR and GE increased accordingly with increase of CTS Mw. To illustrate the possible reasons, we calibrated the Mw and D.D. of CTS by acid–base titration method based on Mark–Houwink equation and the results are also listed in . We surprisingly found that D.D. increased with the CTS Mw. Hence, the higher GR and GE could be ascribed to the higher D.D. providing more active reaction sites.
Table 2. Summary of GR, GE and drug-loading contents of CTS-g-PNIPAAm nanogels prepared from different Mw CTS.
Temperature-sensitive properties of CTS-g-PNIPAAm nanogels
Variance of transmittance of CTS-g-PNIPAAm nanogels
shows the results of CTS-g-PNIPAAm nanogels obtained from transmittance measurements as a function of the temperature. The VPTT appeared at 32.5 °C which was independent to CTS Mw. Our result was in accordance with the former researchers (Fujishige, Citation1973) who reported that LCST of PNIPAAm was independent of Mw and concentration, but could hinge on a critical hydrophilic/hydrophobic balance between the chemical groups on the polymer. However, it was noticed that the variance of transmittance of resulting nanogels possessed a downward trend with the CTS Mw increase. This may be due to (1) the increased viscosity of CTS with the higher Mw prevented the molecular chain extension freely and the approaching of activated monomer; (2) the grafted PNIPAAm with similar Mw contributed less to the thermal features of products prepared from CTS with higher Mw.
Variance of size and SR of CTS-g-PNIPAAm nanogels
As shown in the main map in , the size of resultant nanogels reduced along with the increase of the CTS Mw when the temperature was lower than VPTT and the size decreased to a uniform level when the temperature rose to above VPTT. Meantime, insert map in also shows SR decreased with the increase of CTS Mw. The results were in good accordance with . Therefore, the thermal-responsive characterization of resultant nanogels by transmittance and size corresponded well with each other. Besides, the PNIPAAm, selected as control, showed most obvious size change caused by temperature.
Variance of zeta potential of CTS-g-PNIPAAm nanogels
shows variance of zeta potential of CTS-g-PNIPAAm nanogels at below (25 °C) and above (37 °C) VPTT. The nanogels prepared with the CTS Mw lower than 200 kDa carried weak positive charge below the VPTT. However, both of nanogels prepared with CTS Mw higher than 700 kDa and PNIPAAm nanogels carried negative charge below the VPTT. Interestingly, as the temperature rose above VPTT, CTS-g-PNIPAAm nanogels carried more positive charge, while PNIPAAm nanogels carried more negative charge. This might be caused as all the nanogels carried very weak positive charge below the VPTT resulting from the protonation of unreacted amino. The product prepared from CTS 700 kDa with few residual amino group carried negative charges, as was the same as PNIPAAm nanogels. When the temperature increased to above VPTT, the cross-linking PNIPAAm became hydrophobic and more condensed. Thus, the hydrophilic CTS apparently could carry a more positive charge. This process is illustrated by .
pH-sensitive properties of CTS-g-PNIPAAm nanogels
is very interesting. It was found that the size of resultant nanogels prepared with the CTS Mw lower than 200 kDa increased suddenly when the solution pH increased to above 7.4 and presented pH-responsive structure changes. We thought it was related with D.D. of CTS. The pKa of CTS with Mw lower than 200 kDa was determined as 6.3 by acid–base titration method, while that of resultant products was about 6.8. Therefore, when the solution pH increased to above 6.8, an obvious structure change happened. While the nanogels prepared with CTS Mw 700 kDa were not sensitive to the changes of solution pH, which may due to that the D.D. of CTS 700 kDa was nearly up to 100% (), thus the grafting-copolymerization reaction was so completed that few residual amino remained on the resultant nanogels. Moreover, PNIPAAm, which showed dramatic and reversible phase transition behavior attributed to different hydrogen bond interaction between isopropyl group and water at below and above LCST at around 32 °C, presented pH-sensitive property for the first time. However, the reason was still not clear.
Critical aggregation concentration (CAC) of CTS-g-PNIPAAm nanogels
As detailed by many authors (Liu et al., Citation2009; Ghosh et al., Citation2011; Vasilescu et al., Citation2011), pyrene fluorescence probe method was widely employed to estimate hydrophobic microenvironments within micelles in aqueous medium from the fluorescent excitation spectra of pyrene. At low concentration of the di-block copolymer, a shift of the excitation band at 334 nm is negligible. As polymer concentration increases, a red shift of the excitation band to 338 nm can be clearly detected. CAC is defined as the intersection of the lines drawn through the points of flat regions at low concentration and the drastically increasing regions at high concentration. Plots of pyrene I338/I334 ratio versus logarithm of nanogels concentration are shown in . As expected, the CAC showed a decreasing trend, which was in accordance with the former studies (De Vasconcelos et al., Citation2006). This difference could be attributed to the differences of the GE as mentioned above. The CAC values of CTS-g-PNIPAAm nanogels were smaller than some conventional micelles (Park et al., Citation2010b; Ferreira et al., Citation2011), which might be attributed to that the nanogels were very hydrophilic and tended to be very stable under dilution. The low CAC is especially important for the structural stability of long-circulating polymeric nanocarriers in vivo, since the nanogels structures must be stable in the blood stream after dilution with large volumes of surface-active components in blood.
Drug loading and releasing profiles of CTS-g-PNIPAAm nanogels
As shown in , the drug-loading level of resultant nanogels increased with CTS Mw. The low-loading content might be due to the weak interaction between the HCPT and nanogels. The representative photos by TEM are shown in . These nanoparticles were well dispersed as individual. The size obtained from TEM (50–100 nm) was smaller than that observation from DLS (200–250 nm). This could be related to the fact that DLS measured the hydrodynamic diameter of the nanoparticles in an aqueous environment whereas the TEM micrograph showed the dehydrated solid state of the nanogels (Rodríguez-Hernández et al., Citation2005). The structure of HCPT-loaded nanogels was smaller and more compact, which may result from hydrophobic interaction within HCPT molecules.
Figure 7. TEM of blank (left) and HCPT-loaded (right) CTS-g-PNIPAAm nanogels. Scale bars are 200 nm.
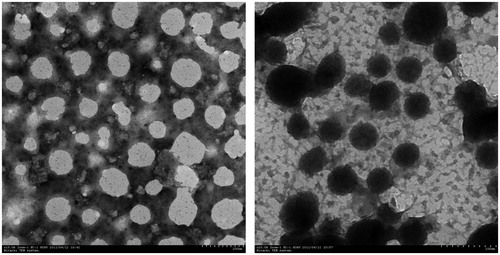
Understanding the release mechanisms, as well as the factors affecting drug release, is important to modify drug release. It is known to all that diffusion, swelling and erosion are the main release mechanisms associated with drug release. Many processes or events influence the rate of drug diffusion and the degradation kinetics, for example, polymer–drug interactions, water absorption, pore closure and so on. Drug release is often preceded by a chain of processes (water absorption, swelling and erosion). The most common way of transport through water-filled pores is diffusion, such as random movements of the molecules driven by the chemical potential gradient, which can often be approximated by the concentration gradient. The other way of transport through water-filled pores is convection, which is driven by a force such as osmotic pressure. Osmotic pressure may be created by the influx of water into a non-swelling system. Drug transport driven by this force is more common in drug delivery systems utilizing hydrophobic polymers (Arifin et al., Citation2006; Siepmann & Siepmann, Citation2008; Sackett & Narasimhan, Citation2011)
PNIPAAm have been widely employed as a non-toxic and responsive polymer whose structure showed dramatic changes with temperature increasing (Schild, Citation1992; Chung et al., Citation1999; Eeckman et al., Citation2004). Since the significant temperature-responsive structure changes cannot complete within 1 °C, to observe the temperature-dependent effects of complete structure changes on the HCPT release from HCPT-loading nanogels, we designed 25 °C and 37 °C to mimic R.T. and normal body temperature. shows the release profiles of HCPT-loading nanogels at below and above VPTT respectively. Several classical mathematical models, such as Higuchi, Ritger–Peppas and Weibull empirical model were employed to describe the possible processes that would enhance or hinder drug release. The fitting results are listed in and. The results showed that the main release mechanism was Higuchi diffusion at both temperatures, probably because our study was well performed under leaking conditions. At 25 °C, the cumulative release percentage decreased with the increasing Mw of CTS, which indicated that the higher Mw CTS held a more complete hydration layer to restrict drug diffusion. Besides, all the profiles showed a short time lag release, which lasted 1–2 h; while at 37 °C, we obtained a good fitting by Ritger–Peppas model. The products prepared with Mw 50 kDa CTS with a slope 0.49 showed a complex mechanism including diffusion and erosion, while products prepared from higher Mw CTS with slope over 0.85 showed a zero-order diffusion profile and might suggest an erosion controlled release. Our results were in good agreements with the former researchers (Sackett & Narasimhan, Citation2011). The cause might be that, at below the VPTT, the double hydrophilic polymer held a diffusion controlled release; while at above the VPTT, the PNIPAAm became hydrophobic and could not swell, and the release behavior was dominated by erosion controlled and osmotic driving.
Figure 8. In vitro release of CTS-g-PNIPAAm nanogels at different temperatures (a)T = 25 °C; (b) T = 37 °C.
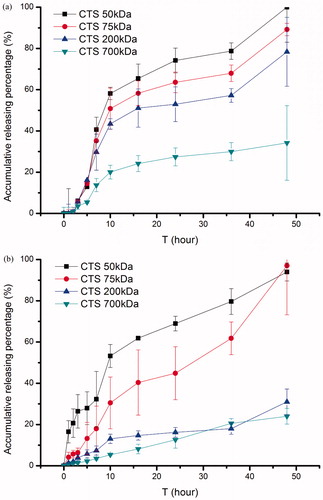
Table 3. Fitting results of HCPT release profiles to several classical kinetic mathematical models.
Cytotoxicity evaluation of cross-linked CTS-g-PNIPAAm nanogels
The in vitro cytotoxicity of CTS-g-PNIPAAm nanogels against human hepatic cells L02 was evaluated by MTT assay. The MTT assay relies on the mitochondrial activity of fibroblasts and represents a parameter for their metabolic activity. As shown in , the cytotoxicity of CTS-g-PNIPAAm nanogels, similar to CTS and PNIPAAm, increased in parallel with concentration increasing from 0.025 to 1 mg/mL after 24 h incubation with human hepatic cells L02. The CTS-g-PNIPAAm nanogels, CTS and PNIPAAm were all found to be more than 100% viable relative to control at the concentration as high as 0.5 mg/mL. Some authors also reported that the gelatin nanoparticles (Gupta et al., Citation2004) and pullulan nanoparticles (Dion Sio et al., Citation2013) were found to be more than 100% viable relative to control at the concentration as high as 0.5 mg/mL. Our results were in good agreement with them. The cause might be that, both of CTS (Ikinci et al., Citation2002; Cho et al., Citation2004) and PNIPAAm (Shimojo et al., Citation2002; Cho et al., Citation2004) were reported to be biocompatible and could be employed as tissue engineering materials. Therefore, the resultant nanogels made of CTS and PNIPAAM retained their original effects to cells in some way.
Conclusion
In this study, a series of CTS-g-PNIPAAm copolymers were synthesized by radical polymerization with APS as an initiator. The average Mw of PNIPAAm was characterized by SLLS method. Then CTS-g-PNIPAAm monodisperse polymer hydrogels, named as nanogels, were obtained by combining cross-linking and polymerization technique with different Mw CTS. Thermal and pH-sensitive characteristics of CTS-g-PNIPAAm nanogels were comprehensively investigated by measuring the transmittance, changeable size and zeta potential. CTS with Mw 200 kDa was the most appropriate candidate for grafting modification to prepare dual-sensitive nanogels. The nanogels prepared from CTS Mw 700 kDa lost its pH-sensitivity but presented a more obvious sustained drug-releasing effect. The anti-tumor drug HCPT was loaded into nanogels by emulsion-evaporation method. Fitting results of HCPT release data to different mathematical models suggested a diffusion-controlled mechanism at 25 °C. However, the release behaviors were dominated by combined effects of polymer erosion and osmotic pressure driven at 37 °C. We suggest this kind of nanogels may provide an alternative way to design intelligent responsive drug carrier followed by further structure modification. Illustration of structures of CTS-g-PNIPAAm nanogels and responsive drug-releasing behaviors was shown in Scheme 1.
Declaration of interest
The authors report no declarations of interest. This study is in part financially supported by the National Natural Science Foundation of China (No. 21373062). Natural Science Foundation of Jiangsu Province (SBK201241121) and Research Special Fund for Central University (JKPZ2013007).
References
- Arifin DY, Lee LY, Wang CH. (2006). Mathematical modeling and simulation of drug release from microspheres: implications to drug delivery systems. Adv Drug Deliv Rev 58:1274–325
- Bajaj H, Sharma VK, Kalonia DS. (2007). A high-throughput method for detection of protein self-association and second virial coefficient using size-exclusion chromatography through simultaneous measurement of concentration and scattered light intensity. Pharm Res 24:2071–83
- Chen C, Liu M, Gao CLS, et al. (2013). A convenient way to synthesize comb-shaped chitosan-graft-poly (N-isopropylacrylamide) copolymer. Carbohydrate Polym 92:621–28
- Cho JH, Kim S-H, Park KD, et al. (2004). Chondrogenic differentiation of human mesenchymal stem cells using a thermosensitive poly(N-isopropylacrylamide) and water-soluble chitosan copolymer. Biomaterials 25:5743–51
- Chuang C-Y, Don T-M, Chiu W-Y. (2010). Preparation of environmental-responsive chitosan-based nanoparticles by self-assembly method. Carbohydr Polym 84:765–9
- Chung JE, Yokoyama M, Yamato M, et al. (1999). Thermo-responsive drug delivery from polymeric micelles constructed using block copolymers of poly(N-isopropylacrylamide) and poly(butylmethacrylate). J Control Rel 62:115–27
- Dash M, Chiellini F, Ottenbrite RM, Chiellini E. (2011). Chitosan–a versatile semi-synthetic polymer in biomedical applications. Progr Polym Sci 36:981–1014
- De Vasconcelos CL, Bezerril PM, Dos Santos DES, et al. (2006). Effect of molecular weight and ionic strength on the formation of polyelectrolyte complexes based on poly(methacrylic acid) and chitosan. Biomacromolecules 7:1245–52
- Dion Sio M, Cordeiro C, Remu NL, et al. (2013). Pullulan-based nanoparticles as carriers for transmucosal protein delivery. Eur J Pharmaceut Sci 50:102–13
- Eeckman F, Moes AJ, Amighi K. (2004). Poly(N-isopropylacrylamide) copolymers for constant temperature controlled drug delivery. Int J Pharm 273:109–19
- Ferreira SA, Pereira P, Sampaio P, et al. (2011). Supramolecular assembled nanogel made of mannan. J Colloid Interface Sci 361:97–108
- Fujishige S. (1973). [Unusual labor complications]. Josanpu Zasshi 27:38–9
- Ghosh A, Yusa S, Matsuoka H, Saruwatari Y. (2011). Surface activity and micellization behavior of cationic amphiphilic block copolymer synthesized by reversible addition-fragmentation chain transfer process. Langmuir 27:9237–44
- Gibson WG. (1987). Levinson's theorem and the second virial coefficient in one, two, and three dimensions. Phys Rev A 36:564–75
- Gupta AK, Gupta M, Yarwood SJ, Curtis ASG. (2004). Effect of cellular uptake of gelatin nanoparticles on adhesion, morphology and cytoskeleton organisation of human fibroblasts. J Control Rel 95:197–207
- Howe AJ, Howe AM, Routh AF. (2011). The viscosity of dilute poly(N-isopropylacrylamide) dispersions. J Colloid Interface Sci 357:300–7
- Ikinci G, Senel S, Akincibay H, et al. (2002). Effect of chitosan on a periodontal pathogen Porphyromonas gingivalis. Int J Pharm 235:121–7
- Li F, Wu H, Zhang H, et al. (2009). Antitumor drug Paclitaxel-loaded pH-sensitive nanoparticles targeting tumor extracellular pH. Carbohydr Polym 77:773–8
- Li W, Li J, Gao J, et al. (2011). The fine-tuning of thermosensitive and degradable polymer micelles for enhancing intracellular uptake and drug release in tumors. Biomaterials 32:3832–44
- Liu Y, Cao X, Luo M, et al. (2009). Self-assembled micellar nanoparticles of a novel star copolymer for thermo and pH dual-responsive drug release. J Colloid Interface Sci 329:244–52
- Liu Z, Jiao Y, Wang Y, et al. (2008). Polysaccharides-based nanoparticles as drug delivery systems. Adv Drug Deliv Rev 60:1650–62
- Mourya VK, Inamdar NN. (2008). Chitosan-modifications and applications: opportunities galore. React Funct Polym 68:1013–51
- Park JH, Saravanakumar G, Kim K, Kwon IC. (2010a). Targeted delivery of low molecular drugs using chitosan and its derivatives. Adv Drug Deliv Rev 62:28–41
- Park W, Park SJ, Na K. (2010b). Potential of self-organizing nanogel with acetylated chondroitin sulfate as an anti-cancer drug carrier. Colloids Surf B Biointerface 79:501–8
- Rejinold NS, Baby T, Chennazhi KP, Jayakumar R. (2014). Dual drug encapsulated thermo-sensitive fibrinogen-graft-poly (N-isopropyl acrylamide) nanogels for breast cancer therapy. Colloids Surf B: Biointer 114:209–17
- Rejinold NS, Chennazhi KP, Nair SV, Jayakumar R. (2013). Thermo-responsive chitosan-graft-poly(N-isopropyl acrylamide) co-polymeric nanoparticles for 5-fluorouracil delivery to breast cancer cells in vitro. J Nanopharmaceut Drug Deliv 1:18–29
- Rejinold NS, Sreerekha PR, Chennazhi KP, et al. (2011). Biocompatible, biodegradable and thermo-sensitive chitosan-g-poly (N-isopropylacrylamide) nanocarrier for curcumin drug delivery. Int J Biol Macromol 49:161–72
- Rodríguez-Hernández J, Ch Cot F, Gnanou Y, Lecommandoux S. (2005). Toward “smart” nano-objects by self-assembly of block copolymers in solution. Progr Polym Sci 30:691–724
- Sackett CK, Narasimhan B. (2011). Mathematical modeling of polymer erosion: consequences for drug delivery. Int J Pharm 418:104–14
- Schild H. (1992). Poly(N-isopropylacrylamide): experiment, theory and application. Progr Polym Sci 17:163–249
- Shimojo S, Akaike T, Hara M, et al. (2002). Difference in the attachment of hepatocytes between a poly(gamma-benzyl L-glutamate) (PBLG)/poly(N-isopropylacrylamide) (PNIPAAm) diblock copolymer cast surface and a PBlg/PNIPAAm Langmuir-Blodgett one. J Biomater Sci Polym Ed 13:829–41
- Siepmann J, Siepmann F. (2008). Mathematical modeling of drug delivery. Int J Pharmaceut 364:328–43
- Varshosaz J. (2007). The promise of chitosan microspheres in drug delivery systems. Expert Opin Drug Deliv 4:263–73
- Vasilescu M, Angelescu DG, Bandula R, Staikos G. (2011). Microstructure of polyelectrolyte nanoaggregates studied by fluorescence probe method. J Fluoresc 21:2085–91
- Wang Y, Wang J, Ge L, et al. (2013). Synthesis, properties and self-assembly of intelligent core-shell nanoparticles based on chitosan with different molecular weight and N-isopropylacrylamide. J Appl Polym Sci 127:3749–59
- Wei H, Cheng S-X, Zhang X-Z, Zhuo R-X. (2009). Thermo-sensitive polymeric micelles based on poly(N-isopropylacrylamide) as drug carriers. Progr Polym Sci 34:893–910
- Zhang J, Chen XG, Sun GZ, et al. (2010). Effect of molecular weight on the oleoyl-chitosan nanoparticles as carriers for doxorubicin. Coll Surf B: Biointerf 77:125–30
- Zhou HY, Chen XG, Kong M, et al. (2008). Effect of molecular weight and degree of chitosan deacetylation on the preparation and characteristics of chitosan thermosensitive hydrogel as a delivery system. Carbohydr Polym 73:265–73