Abstract
Levodopa (l-DOPA) is the most effective pharmacologic agent in Parkinson’s disease and remains the “gold standard”. Nevertheless, in long-term treatments, dyskinesias and motor complications can emerge. In this work, the combined use of l-DOPA methylester hydrochloride prodrug (LDME) with transbuccal drug delivery was supposed as a good alternative method to optimize the bioavailability of l-DOPA, to maintain constant plasma levels and to decrease the drug unwanted effects. The effects of environmental pH on buccal delivery of LDME were evaluated ex vivo. The increase of pH value from 5.8 to 6.2 implies an improvement of drug permeation. Since the pH increase causes the raising of hydrolytic conversion of LDME to l-DOPA, the pH value 6.2 was considered as a good compromise between drug stability and permeation rate. It was found that during the passage through the biological tissue, LDME undergoes a primary conversion to l-DOPA catalyzed by membrane’s enzymes. Supplementation of delivery with Tween 80® produces substantial enhancement of LDME passage through the membrane. The drug could be loaded in the IntelliDrug mechatronic device, released close to the buccal mucosa, so achieving and maintaining constant therapeutic blood levels for extensive time.
Introduction
Given the rapidly aging population, Parkinson’s disease (PD) is becoming a major public health issue in Europe. According to World Health Organization, the overall prevalence in Europe of PD is 1.6% in people older than 65 years. More than one million people live with PD in Europe today, and this number is forecast to double by 2030 (Dorsey et al., Citation2007).
Despite the introduction of different dopamine (DA) agonists for treatment of PD, Levodopa (l-DOPA), the precursor of DA, is the most effective pharmacologic agent and, even after half a century, it remains the treatment of choice and the “gold standard”. Usually, l-DOPA is administered orally by conventional dosage forms, and the clinical response is greatly affected by irregular absorption in the g.i. tract, short half-life and severe hepatic metabolism. Unfortunately, due to both the drug physico-chemical characteristics and the route of administration, the oral bioavailability of l-DOPA is about 5–15% of the administered doses and only an amount less than 1% enters the brain as unchanged drug (Moffat et al., Citation2004). The drug is slightly water soluble, has low lipophilicity and is highly susceptible to peripheral chemical and enzymatic decarboxylation. Following oral administration of l-DOPA, peripheral transformation to DA is responsible of severe side effects, including nausea, vomiting and cardiac irregularity (Mercuri & Bernardi, Citation2005; Rao et al., Citation2006; Di Stefano et al., Citation2008; Contin & Martinelli, Citation2010; Zhou et al., Citation2010).
During chronic treatment with l-DOPA, a variety of problems can emerge, e.g. the appearance of dyskinesias and motor dysfunction (Pahwa & Lyons, Citation2011), decrease of drug effects (phenomenon “wearing-off”) and increase of susceptibility to fluctuations in l-DOPA plasma levels (phenomenon “on-off”). After five years of therapy, approximately 50% of the treated patients start to suffer from motor fluctuations and dyskinesias. After nine years, these symptoms reach 70% of treated population, and a narrowing of the therapeutic window is observed. As a consequence, patients should intensify the dosage regimen that implies an increase of unwanted side effects (Varanese et al., Citation2011; Jenner et al., Citation2011).
The main goal in the treatment of PD could be the maintenance of constant DA stimulation. Keeping constant drug levels the dose-dependent side effects should be avoided, and the patient’s quality of life improved. In addition, few medications are needed, hospitalizations due to complications are reduced and assistive costs are decreased (Herrlich et al., Citation2011).
Drug plasma fluctuations could be, in part, circumvented bypassing the oral route and using alternative methods of drug delivery. Indeed, intravenous infusions of l-DOPA alone or in combination with a decarboxylase inhibitor dramatically extend the duration of drug effects, reduce fluctuations and provide significant mobility improvement. Unfortunately, l-DOPA is not very water soluble, and this delivery approach not only makes the administration of adequate doses impractical but is also problematic for routine clinical use (Wright & Waters, Citation2013).
The use of l-DOPA prodrugs could be considered as a useful means to improve the drug bioavailability; in particular, l-DOPA esters show high water solubility and efficiently cross the biological membranes due to the improved diffusion properties. Furthermore, the ester derivatives are quickly converted in vivo to the parent drug by nonspecific esterases (Di Stefano et al., Citation2008; Goole & Amighi, Citation2009; Itoh & Oo, Citation2010; Zhou et al., Citation2010).
The successful of therapy is often related to the route of drug delivery that affects administration, absorption and distribution. To optimize the bioavailability of drugs, transepithelial route is considered as a good alternative way. It has been reported that combination of nasal delivery with the use of l-DOPA alkylesters could concur to rapid and complete absorption, thus avoiding of the typical failures of l-DOPA (Kao et al., Citation2000).
Buccal mucosa is a non-invasive delivery route that allows achieving effective drug plasma and steady state levels very quickly and maintaining these concentrations for prolonged periods. Buccal delivery implies ease of administration, avoidance of hepatic first-pass metabolism and degradation in the gastrointestinal tract, excellent accessibility, easy removal in emergencies and good patient compliance. With respect to conventional peroral administration, these behaviors could contribute to higher drug bioavailability following the delivery of smaller doses (Campisi et al., Citation2010a; Patel et al., Citation2011; Senel et al., Citation2012).
In addition, the delivery of drugs through buccal mucosa could be compared to a slow i.v. infusion. The combined use of l-DOPA methylester hydrochloride prodrug (LDME, melevodopa hydrochloride) with transbuccal drug delivery was supposed as a good alternative method to optimize the bioavailability of l-DOPA. In this article, we report a study on the behavior of LDME in the oral cavity environment in order to evaluate its potential as candidate for buccal delivery. It is plausible that continuous passage of LDME through the tissue will be able to maintain constant plasma l-DOPA levels while obviating first-pass metabolism and dose-dependent side-effects.
Materials and methods
Materials
l-DOPA methyl ester hydrochloride (LDME), l-DOPA and polyoxyethylene sorbitan monooleate (Tween 80®) were obtained from Sigma-Aldrich (Milan, Italy). Buffer (pH 6.8) solution simulating saliva was prepared using KCl (1.5 g), NaHCO3 (1.75 g), KSCN (0.5 g), NaH2PO4 H2O (0.5 g) and lactic acid (1.0 g) in 1 L of distilled water (Gal et al., Citation2001). Phosphate buffer (pH 7.4) saline solution, Ca2+ and Mg2+ free, was prepared by dissolving KH2PO4 (2.8 g), anhydrous Na2HPO4 (20.9 g) in 1 L of distilled water and used as simulated plasma. Citrate buffer solutions (pH 5.8, 6.0, 6.2 and 6.5) were prepared using the appropriate amounts of citric acid and sodium citrate in 1 L of distilled water. All media were adjusted to isotonicity (290 mOsm/L) with sodium chloride. Saline isotonic solution (pH 7.0) was prepared by dissolving NaCl (9 g) in 1 L of distilled water. pH values were verified with an ORION 520 A+ pH meter equipped with an ORION 8102BN ROSS electrode (Thermo Scientific, Rodano, Italy).
HPLC solvents (HiPerSolv Chromanorm) were purchased from VWR International (Milan, Italy). All chemicals and solvents were of analytical grade and were used without further purification. In chemical and enzymatic stability tests, HPLC analyses were performed with a HPLC Agilent instrument equipped with a Quaternary Pump VL 1260 Infinity and UV detector 1260 VL + Infinity, 20 µL injector and a computer-integrating apparatus (OpenLAB CDS ChemStation Workstation, Agilent Technologies Inc., Wilmington, DE).
Evaluation of LDME hydrolysis
The ester bond chemical hydrolysis of LDME and the related formation of l-DOPA were evaluated at 37 ± 0.2 °C using buffer solutions at different pH values (pH 5.8, 6.0, 6.2, 6.5, 6.8 and 7.4) and buffer solution at pH 6.2 in the presence of Tween 80®. Experiments were initiated by adding 1 or 50 mg of LDME in 1 mL of the appropriate buffer solution; every 60 min, samples (25 µL) were withdrawn and immediately analyzed by HPLC.
Conversion of LDME to l-DOPA was also evaluated putting in contact the drug solution with porcine buccal mucosa specimens. Mucosal tissue was removed from the inner cheek (buccal area) of freshly slaughtered domestic pigs. After sampling, the specimens were transferred to the laboratory within 1 h in a refrigerated transport box. Experiments were initiated by distributing the LDME solution (50 mg in 1 mL of buffer pH 5.8 or pH 6.2 solution) on the surface of fresh tissue specimens (area 0.384 cm2). Every 60 min, samples (25 µL) of tested solutions were withdrawn and immediately analyzed by HPLC. All hydrolysis experiments were carried out for 6 h and repeated six times.
Preparation of porcine buccal mucosa for permeability studies
For ex vivo studies, the mucosal specimens were surgically treated to remove excesses of connective and adipose portion and subjected to thermal shock to separate the epithelial stratum from the whole tissue. The mucosal tissue was dipped for approximately two minutes in saline solution pre-warmed at 60 °C. The connective tissue was carefully detached from the mucosa to obtain mucosal samples 250 ± 25 μm thick. The thickness of the tissues was measured using a digital micrometer. Slicing of the tissue with a dermatome was not performed to avoid preliminary freezing, which may damage the buccal mucosa. Heat treatment did not adversely affect on permeability and integrity characteristics of the buccal mucosa (Kulkarni et al., Citation2010; De Caro et al., Citation2012).
Permeation studies
Appropriate disks of mucosa were mounted in vertical Franz type diffusion cells (Permegear, flat flange joint, 7 mm orifice diameter, 15 mL acceptor volume, SES GmbH – Analysensysteme, Bechenheim, Germany) in such a way that the epithelium faced the donor chamber and the serosal side faced the acceptor chamber. The exposed surface area of mucosa was 0.38465 cm2. A constant temperature of 37 ± 0.2 °C was maintained during the experiments to mimic the in vivo environment. Tissue disks were equilibrated for 10 min at 37 ± 0.2 °C [Polimix EH 2 bath equipped with a constant rate adjustable stirrer RECO S5 (Kinematica, Lucerne, Switzerland)] adding saline isotonic solution both in the donor and acceptor compartment. After equilibration, the saline solutions were removed and replaced with the suitable buffer solutions. Notably, 1 mL of the following drug-buffered solutions were placed in the donor compartment:
pH 5.8 containing 50 mg/mL LDME,
pH 5.8 containing 1.5 mg/mL l-DOPA,
pH 6.2 containing 50 mg/mL LDME,
pH 6.2 containing 1.5 mg/mL l-DOPA,
pH 6.2 containing 50 mg/mL LDME in the presence of 0.1% Tween 80® (w/v),
pH 6.2 containing 50 mg/mL LDME in the presence of 1% Tween 80® (w/v),
pH 6.2 containing 50 mg/mL LDME in the presence of 2% Tween 80® (w/v),
pH 6.2 containing 1.5 mg/mL l-DOPA in the presence of 0.1% Tween 80® (w/v),
pH 6.2 containing 1.5 mg/mL l-DOPA in the presence of 1% Tween 80® (w/v),
pH 6.2 containing 1.5 mg/mL l-DOPA in the presence of 2% Tween 80® (w/v),
respectively.
The acceptor chamber was filled with 15 mL of citrate buffer pH 5.8 or pH 6.2 solutions. Every 60 min, 0.5 mL samples were withdrawn from the receiver chamber, replacing the removed volume with same amount of fresh buffer solution (pH 5.8 or pH 6.2). In all experiments, the drug transferred from the donor to the acceptor compartment was monitored by HPLC analysis. Permeation studies were carried out for up to 6 h. Results are reported as mean value ± SD of six different experiments in which fractions of the same portion of tissue were used (p < 0.05). At the end of each experiment, in the donor compartment, the percent amount of administered drug was recognized. The integrity of the mucosal tissue was monitored after each permeability experiment, according to the method previously reported (De Caro et al., Citation2008).
HPLC analysis
During permeation experiments, the amount of drug (LDME or l-DOPA) transferred in the acceptor compartment was measured by HPLC analysis using the appropriate calibration curve. HPLC method was found simple, accurate and reproducible. Chromatographic separation was achieved on a reversed-phase column Phenomenex Gemini-NX (Phenomenex Inc, Torrance, CA), (5 μm, 15 cm × 4.6 mm), a mobile phase consisted of trifluoroacetic acid (TFA) 0.01% (v/v) aqueous solution and acetonitrile (95:5 v/v) in isocratic condition. The flow rate was set at 1 mL/min and the UV wavelength at 280 nm. In these conditions, the retention time for l-DOPA and LDME were 1.99 and 2.90 min, respectively. Standard curves were used for quantification of integrated areas under peaks. The calibration curves were performed in the concentration range of 0.001–1 mg/mL. Validation parameters of LDME in buffer pH 5.8 solution: regression equation Y = 1.136·104 X + 4.21, correlation coefficient 0.9998; in buffer pH 6.2 regression equation Y = 1.139·104 X + 5.23, correlation coefficient 0.9997. Validation parameters of l-DOPA in buffer pH 5.8 solution: regression equation Y = 9.95·103 X + 7.72, correlation coefficient 0.9995; in buffer pH 6.2 solution: regression equation Y = 2.82·104 X + 7.71, correlation coefficient 0.9998. At the testing concentrations, no interferences among LDME or l-DOPA and buffer solution components were observed.
Data analysis
The flux values (Js) across the membranes were calculated at the steady state per unit area by linear regression analysis of permeation data following the relationship Js = Q/At (mg/cm2h), where Q is the quantity of l-DOPA recovered in the acceptor compartment, A is the active cross-sectional area available for diffusion (cm2) and t is the time of exposure (h).
All data are presented as mean ± SD of at least six replicated experiments, unless otherwise stated. Data were elaborated using Curve Expert version 1.4 (by DG Hyams, Starkville, MS) and Kaleidagraph 3.5 (Sinergy Software Inc, Reading, PA) as software. Linear or non-linear least squares fitting methods were used to determine the optimum values for the parameters present in each equation. Analysis of variance was followed by t-test. The level of significance was selected as (p < 0.05).
Results and discussion
To verify the chance of use buccal mucosa as an alternative route for delivery LDME in treatment of PD, beginning, we considered central to assess the behavior of the active in the oral cavity environment. The study was aimed to verify the stability of the active in the physiological conditions of the oral cavity, and the ability of the active to permeate the buccal mucosa.
Stability studies
Preliminary, chemical stability of LDME was established using both artificial non-enzymatic saliva and simulated plasma (buffer solutions at pH 6.8 and pH 7.4, respectively). Hydrolysis was monitored by HPLC, evaluating both the disappearance of LDME and the appearance of its main metabolite l-DOPA. A progressive and constant formation of l-DOPA occurs in both conditions, suggesting a hydrolysis process of the ester bond that reaches 8.3% and 11% of the administered dose after 6 h in buffer solutions at pH 6.8 and pH 7.4, respectively. A concomitant and progressive darkening of solution indicated the formation of a decomposition product, which imparts to the sample a brown color. This phenomenon was attributed to the formation of oxidative degradation products, seeing that both LDME and l-DOPA following the same reaction pathway, in alkaline media and in the presence of molecular oxygen, are easily auto-oxidized to dihydroquinone derivatives. During this chain process, the drugs are likely transformed into semiquinone species by hydroxyl ions. In turn, the semiquinone derivatives are oxidized to open-chain quinones that undergo intramolecular-pH dependent cyclization to dopaminochrome and others brown byproducts (Giannola et al., Citation2008).
It has been reported that l-DOPA remains almost completely in non-oxidized form in acidic solutions (Kankkunen et al., Citation2002), so that chemical stability of LDME was evaluated also in buffer solutions ranging from pH 5.8 to pH 6.5. The decrease of pH value reduces the drug hydrolysis rate; even for relatively small variations of pH the reduction of reaction rate was observed, as shown in .
Figure 1. Amount of l-DOPA formed by hydrolysis of LDME versus time in buffered solutions at pH 7.4 (▴), pH 6.8
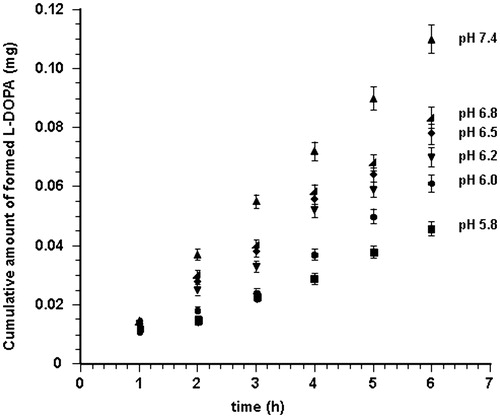
In summary, when the pH value was >6.5, more than the appearance of l-DOPA in the reaction solution, a brownish color that increased with the pH value took place. Indeed, exceeded pH 6.5, the hydrolysis trend () is not reflective of the real process since the formed l-DOPA undergoes rapid oxidation and its HPLC quantification at 280 nm does not reveal the actual amount of drug. Probably, also at pH values <6.5 oxidation occurs; however, it is negligible and the color change is not sufficient to disturb quantification.
The hydrolytic behavior of LDME is resumed in and expressed as percent average amount calculated on the basis of l-DOPA detected in buffered media at different pH values after six hours of experiments (n = 6).
Table 1. Hydrolysis of LDME in absence and in presence of mucosal tissue after six hours of experiments (n = 6).
Permeation studies
Since drug permeation through the mucosa is the rate-determining step for buccal absorption, in this study, the LDME ability to cross the buccal tissue and the main membrane transport mechanism were assessed. Drug passage was evaluated using porcine buccal mucosal specimens obtained from tissue removed from the inner cheek (buccal area) of freshly slaughtered domestic pigs.
Sections of mucosa were mounted in Franz type diffusion cells. To avoid misleading of quantification data due to the potential LDME chemical transformation, the donor compartment was placed a drug buffered (pH 5.8) solution and the acceptor compartment was filled with buffered (pH 5.8) solution. Each experiment was carried out for 6 h. At each time interval, the drug amount transferred in the acceptor compartment was monitored by HPLC analysis. The results showed that, in these conditions, the only specie present in the acceptor compartment was l-DOPA. Drug permeation profile was obtained by plotting the cumulative amount of l-DOPA appearing in the receptor phase versus time ().
Figure 2. Cumulative amount of l-DOPA that reaches the receptor phase versus time following delivery of LDME solution (50 mg/mL, 37 ± 0.2 °C) at pH 5.8 (•) and pH 6.2 (▪). Values are presented as mean ± SD (n = 6).
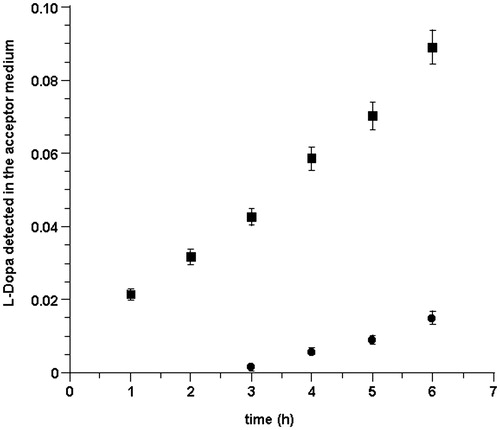
Permeation profile shows that, following administration of LDME, the appearance of l-DOPA in the acceptor medium, after a lag phase of about 2.5 h, is linear with time. The drug flux value through porcine buccal mucosa was 11.855 ± 0.237 µg/cm2 h.
These data did not agree with our expectations: in particular, the only species present in the acceptor phase was l-DOPA, and the drug flux was surprisingly low. We attributed this behavior to a limited aptitude of LDME to cross the membrane in the pH 5.8 conditions. It has been reported that rapid and enhanced drug absorption occurs in pH conditions at which maximum aqueous solubility of both unionized and ionized species are present, and the maximum absorption is usually a compromise between pH solubility and pH permeability (Wang et al., Citation2010; Meng-Lund et al., Citation2014). Indeed, as estimated by calculations, at pH 5.8, only 6.29% of LDME is in its undissociated form and hence the amount of drug available for permeation is low. Accordingly, we performed the same experiments using a pH 6.2 citrate buffer solution where 14.42% of LDME is in its undissociated form (http://www.chemicalize.org/structure/#!mol=melevodopa&source=fp).
Once more, results proved that the only species present in the acceptor compartment is l-DOPA. In these conditions, the flux value was 32.84 ± 0.66 µg/cm2 h. shows the drug permeation profile at pH 6.2 in comparison to that observed at pH 5.8.
Slightly increasing the pH of the donor solution caused an increase of drug permeation and a reduction of the lag phase. Permeation improvement appears to be consistent with the pH-partition hypothesis. The pH value 6.2 was considered as a good compromise between drug stability and undissociated amount available for permeation.
If on one the hand, the use of donor solutions at pH 6.2 produces a significant improvement of drug flux, on the other hand, it does not imply the passage of LDME. The occurrence of only l-DOPA in the acceptor phase could be attributed to the following:
(a) chemical/enzymatic hydrolysis of LDME in the donor compartment and consequent diffusion of formed l-DOPA;
(b) enzymatic hydrolysis of LDME during its permeation through the mucosal tissue; and
(c) rapid chemical hydrolysis of LDME in the acceptor after its permeation through the mucosa.
This last supposition could be taken out in the light of stability evidences obtained during chemical hydrolysis experiments.
Seeing that chemical hydrolysis in the experimental conditions produces, after six hours, only 4.5 and 7% of l-DOPA at pH 5.8 and 6.2, respectively, we verified if a concomitant enzymatic hydrolysis can take place in the donor compartment during the permanence of the drug on the mucosal tissue. So, the hydrolytic conversion of LDME to l-DOPA was evaluated in vitro by putting the drug solution in contact with specimens of buccal mucosa just removed from freshly slaughtered domestic pigs.
The results showed that, in both pH 5.8 and 6.2 conditions, the hydrolytic conversion in the presence of mucosa was a little greater than the chemical hydrolysis alone () but not sufficient to transform the whole dose of LDME to l-DOPA. In , it is reported the cumulative conversion of LDME after six hours of experiments (n = 6) as average percent amount of l-DOPA detected in the hydrolysis medium with respect of the administered l-DOPA equivalent molar amount.
Figure 3. l-DOPA formed in absence (chemical hydrolysis) and in presence (cumulative: chemical plus enzymatic hydrolysis) of mucosal tissue versus time following delivery of LDME solution (50 mg/mL, 37 ± 0.2 °C) at: pH 5.8 chemical (♦); pH 5.8 cumulative (▾); pH 6.2 chemical (▪); and pH 6.2 cumulative (•). Values are presented as mean ± SD (n = 6).
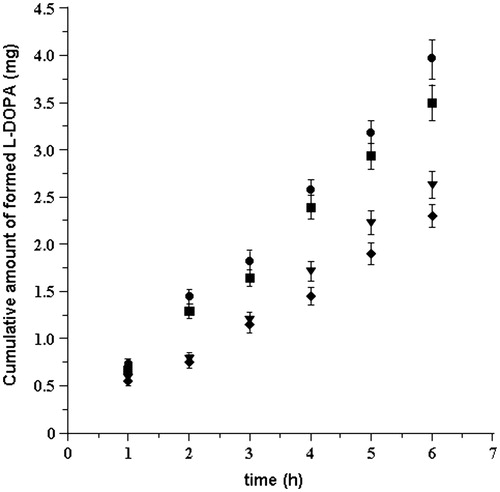
To validate our hypotheses, we evaluated also the behavior of l-DOPA in the same experimental conditions. For this purpose, diffusion studies of per se l-DOPA were carried out using 1.5 mg/mL drug solutions (maximum solubility at 37 °C). The amount of l-DOPA transferred in the acceptor compartment was reported versus time ().
Figure 4. Cumulative amount of drug that reaches the acceptor compartment versus time following delivery of l-DOPA solution (1.5 mg/mL, 37 ± 0.2 °C) at pH 5.8 (♦) and pH 6.2 (▾). Values are presented as mean ± SD (n = 6).
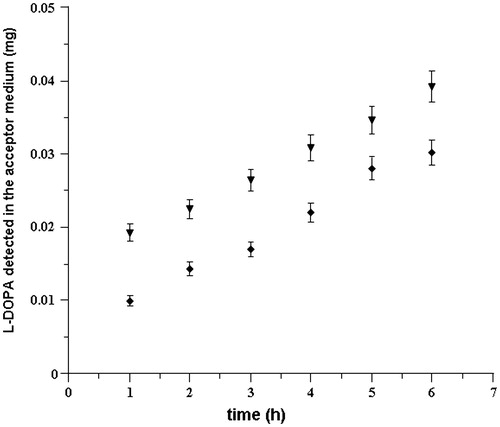
The drug fluxes were calculated as 10.72 ± 0.222 μg/cm2 h and 10.41 ± 0.21 μg/cm2 h at pH 5.8 and pH 6.2, respectively. At the two different pH values, fluxes resulted to be quite similar (p < 0.05). According to literature data, l-DOPA crosses bio-membranes by a saturable carrier-mediated transport mechanism that could justify what has been experienced (Contin & Martinelli, Citation2010).
These results are in agreement with a possible hydrolytic conversion of LDME to l-DOPA during its permeation through the mucosal tissue. Really, in the acceptor medium were not found traces of LDME, even if the detected l-DOPA is greater than that occurred in permeation experiments following delivery of only l-DOPA. Moreover, as observed in LDME permeation studies, at the end of each experiment, in the donor compartment, more than 90% of administered drug was recognized.
However, the amount of drug that reaches the acceptor phase is not adequate to the requested therapeutic levels. To facilitate the drug passage through the tissue, permeation could be promoted by means of chemical and/or physical enhancement techniques. Many different techniques are commonly used to improve the bioavailability of poorly water soluble, but permeable drugs. In this study, optimization of the drug penetration rate was achieved by adding a chemical enhancer to the drug solution. Currently, permeation enhancement is attributed to different mechanisms: extraction membrane protein or lipids, membrane fluidization, disturb the intracellular lipid packing, acting on the components at tight junctions and increasing the thermodynamic activity of drugs (Notman & Anwar, Citation2013). The use of surfactants can improve drug solubility in an aqueous medium (e.g. by entrapment of the active within micelles) and drug permeation through the membranes (Chaudhary et al., Citation2012). Surfactants are believed to enhance the transbuccal permeation by extraction of membrane protein and lipids, and tissue swelling. The critical micelle concentration (CMC) and the hydrophilic–lipophilic balance (HLB) are the two main parameters of enhancers that can alter the membranes organization leading to an increase in permeation. At concentration exceeding the CMC, the barrier properties of the buccal mucosa are reduced, thus allowing the drug passage. The effect is markedly increased when the concentration is above the CMC. Polysorbates are widely used in cosmetics, food products and oral, parenteral and topical pharmaceutical formulations, and are generally regarded as nontoxic and nonirritant materials (Rowe et al., Citation2009; Thackaberry et al., Citation2010). Among polysorbates, we selected Tween 80®, a non-ionic surfactant that possesses optimal physico-chemical properties (HLB, 15.0; CMC, 0.014 mg/mL), it is widely used in the manufacture of a variety of pharmaceutical products and is known for its ability to induce alteration of physicochemical properties of the biological barriers, to change the vehicle-membrane partition coefficient, thus improving the permeability of phospholipid membranes (Wan & Lee, Citation1974; Akhtar et al., Citation2011; Amani et al., Citation2011; Rai et al., Citation2011).
In this study, surfactant concentrations were chosen in a range that agrees with reported data (Veuillez et al., Citation2001). When 0.1% Tween 80® (w/v) was added to the drug solution, no significant increase in the permeation of LDME was observed. Following addition of 1% Tween 80® (w/v) showed slight increase in the permeation and the flux value was 36.31 ± 6.24 µg/cm2 h (p < 0.05; enhancement ratio 1.12). As mentioned above, in the acceptor compartment, l-DOPA was the only present species. The addition of 2% Tween 80® (w/v) to the solution of LDME determined a doubling of l-DOPA amount in the acceptor phase, and the flux value reached 71.22 ± 1.27 µg/cm2 h (; enhancement ratio 2.17). Moreover, as confirmed by hydrolysis studies, the presence of Tween 80® produces a little improvement of LDME stability (), probably due to the drug–surfactant interaction.
Figure 5. Cumulative amounts of l-DOPA in the acceptor compartment versus time: (a) following delivery of LDME at pH 6.2 in absence of enhancer (•) and in the presence of 1% (▪) and 2% (♦) of Tween 80®. (b) Following delivery of l-DOPA in absence of enhancer (○) and in the presence of 1% (□) and 2% (⋄) of Tween 80®. Values are presented as mean ± SD (n = 6).
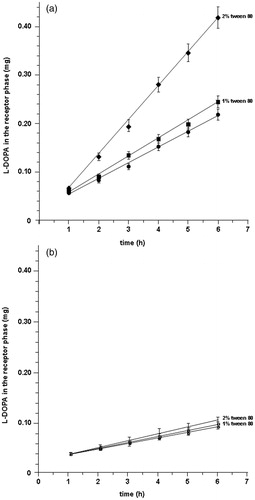
Once more, to validate our suppositions, we evaluated also the behavior of l-DOPA per se in the same experimental conditions; solutions of only l-DOPA supplemented with the same amounts of Tween 80® showed very little increase in drug flux (13.02 ± 2.70 µg/cm2 h) only when the enhancer was added at 2% (; enhancement ratio 1.25).
These data support our preliminary assumption that LDME passively crosses the buccal mucosa, undergoing a conversion reaction to l-DOPA during transmembrane passage, whereas l-DOPA crosses the membrane via a saturable carrier-mediated transport, as primary mechanisms.
In PD therapy, after administration of SINEMET® 50–200 to elderly subjects, the average levels of l-DOPA at steady state is 74 ng/mL (see the patient package insert at http://dailymed.nlm.nih.gov/dailymed/archives/fdaDrugInfo.cfm?archiveid=4188). However, the very large therapeutic range 0.3–1.0 mg/L is also reported (Dutton et al., Citation1993). Indeed, the threshold serum concentration of l-DOPA is extremely variable since it depends on various factors primarily including patient’s sex, age, conditions, diet and in particular the progression of the disease. Moreover, the pharmacodynamic response to l-DOPA changes during long-term therapy so that personalized doses should be administered.
These variability problems could be overcome by the use of the IntelliDrug device as a controlled delivery system. The device is an electronically controlled intraoral micro-system based on real-time sensing and remote control for personal and/or external supervision. It can be implanted or inserted onto a prosthetic tooth crown or a dental implant. Allocated closely to the buccal mucosa, it is able to control the administered drug amount and delivery time (Scholz et al., Citation2008). The device contains a medication reservoir and is able to release the medication according to the patient’s needs over periods of days, weeks or months. The delivery is typically done in accordance to a pre-programmed regimen and at controlled rate. The time of exposure and the mucosal area exposed to drug solution can be regulated. The system can be equipped with a refillable cartridge (BuccalDose) as reservoir (Herrlich et al., Citation2012). The drug reservoir can be loaded by combining two or more drugs in a simple and non-invasive way. The patient can replace the drug delivery cartridge that is docked on a partial removable prosthesis (Campisi et al., Citation2010b; Giannola et al., Citation2010; Herrlich et al., Citation2011; Paderni et al., Citation2013).
On the basis of the above-reported drug plasma levels needed for therapeutic effects and considering our experimental results on LDME flux through the porcine mucosa, we believe that drug permeation could be sufficient in treatment of PD. Following application of the IntelliDrug mechatronic device, the drug solution could be spread on adequate mucosal surface for pre-programmed times thus achieving the desired personalized doses.
We estimated that, loading the reservoir cartridge with a buffered pH 6.2 LDME solution containing 50 mg/mL (supplemented with 2% Tween 80®) and spraying few microliters of this solution on mucosal surface for adequate time, the therapeutic 0.3 mg/L level should be reached. After this time, the solution delivery could be reduced until the blood entrance of the drug matches the eliminated amount.
With the aim of prolonging drug effects, supplementation of LDME with decarboxylase inhibitors like carbidopa, benserazide, entacapone or their combination could be added. This addition should also reduce fluctuations of drug plasma levels and provide significant mobility improvement.
Conclusions
This work systematically evaluated the effect of pH on the buccal delivery of LDME in ex vivo permeation behavior. It was found that the increase of pH value from 5.8 to 6.2 implies an improvement of drug passage, probably due to raising of its undissociated form; at the same time the lag time becomes negligible. On the other hand, the pH increase causes the raising of hydrolytic conversion to l-DOPA. The pH value 6.2 could be considered as a good compromise between drug stability and undissociated amount available for permeation. Since in the acceptor compartment, LDME was not detected, but only l-DOPA, we suppose that, during the passage through the biological tissue, the drug undergoes a conversion catalyzed by membrane’s esterases, in addition to the pH induced hydrolysis.
The addition of 2% Tween 80® to the delivery solution of LDME produced an enhancement in concentration of l-DOPA in the acceptor medium. We assume that the development of an adequate buccal dosage system containing LDME could constitute a promising new way for l-DOPA administration.
The LDME solution could be loaded in the IntelliDrug mechatronic device, close to the buccal mucosa, so achieving and maintaining constant therapeutic blood levels for extensive time. It could provide a new opportunity in management of PD.
Declaration of interest
The authors report no conflicts of interest.
This study was supported by the European Commission that partially funded the HELP Consortium through the Ambient Assisted Living Joint Programme (no.: aal-2008-1-022) and the MIUR, Rome.
References
- Akhtar N, Rehman MU, Khan HMS, et al. (2011). Penetration enhancing effect of polysorbate 20 and 80 on the in vitro percutaneous absorption of l-ascorbic acid. Trop J Pharm Res 10:281–8
- Amani A, York P, de Waard H, Anwar J. (2011). Molecular dynamics simulation of a polysorbate 80 micelle in water. Soft Matter 7:2900–8
- Campisi G, Paderni C, Saccone R, et al. (2010a). Human buccal mucosa as an innovative site of drug delivery. Curr Pharm Des 16:641–52
- Campisi G, Giannola LI, Florena AM, et al. (2010b). Bioavailability in vivo of naltrexone following transbuccal administration by an electronically-controlled intraoral device: a trial on pigs. J Control Release 145:214–20
- Chaudhary A, Nagaich U, Gulati N, et al. (2012). Enhancement of solubilization and bioavailability of poorly soluble drugs by physical and chemical modifications: a recent review. J Adv Pharm Educ Res 2:32–67
- Contin M, Martinelli P. (2010). Pharmacokinetics of levodopa. J Neurol 257:253–61
- De Caro V, Giandalia G, Siragusa MG, et al. (2008). Evaluation of galantamine transbuccal absorption by reconstituted human oral epithelium and porcine tissue as buccal mucosa models: part I. Eur J Pharm Biopharm 70:869–73
- De Caro V, Giandalia G, Siragusa MG, et al. (2012). New perspectives in treatment of Parkinson's disease: studies on permeation of ropinirole through buccal mucosa. Int J Pharm 429:78–83
- Di Stefano A, Sozio P, Cerasa LS. (2008). Antiparkinson prodrugs. Molecules 13:46–68
- Dorsey ER, Constantinescu R, Thompson JP, et al. (2007). Projected number of people with Parkinson’s disease in the most populous nations, 2005 through 2030. Neurology 68:384–6
- Dutton J, Copeland LG, Ptayfer JR, Roberts NB. (1993). Measuring l-Dopa in plasma and urine to monitor therapy of elderly patients with Parkinson disease treated with l-Dopa and a dopa decarboxylase inhibitor. Clin Chem 39:629–34
- Gal JY, Fovet Y, Adib-Yadzi M. (2001). About a synthetic saliva for in vitro studies. Talanta 53:1103–15
- Giannola LI, De Caro V, Giandalia G, et al. (2008). Synthesis and in vitro studies on a potential dopamine prodrug. Pharmazie 63:704–10
- Giannola LI, Paderni C, De Caro V, et al. (2010). New prospectives in the delivery of galantamine for elderly patients using the IntelliDrug intraoral device: in vivo animal studies. Curr Pharm Des 15:653–9
- Goole J, Amighi K. (2009). Levodopa delivery systems for the treatment of Parkinson’s disease: an overview. Int J Pharm 380:1–15
- Herrlich S, Spieth S, Nouna R, et al. (2011). Ambulatory treatment and telemonitoring of patients with Parkinson’s disease. In: Wichert R, Eberhardt B, eds. Ambient assisted living. Advanced technologies and societal change series. Berlin: Springer, 295–305
- Herrlich S, Spieth S, Messner R, Zengerle R. (2012). Osmotic micropumps for drug delivery. Adv Drug Deliv Rev 64:1617–27
- Itoh S, Oo C. (2010). A feasibility study of differential delivery of levodopa ester and benserazide using site-specific intestinal loops in rats. J Pharm Sci 99:227–33
- Jenner P, McCreary AC, Scheller DKA. (2011). Continuous drug delivery in early- and late-stage Parkinson’s disease as a strategy for avoiding dyskinesia induction and expression. J Neural Transm 118:1691–702
- Kankkunen T, Huupponen I, Lahtinen K, et al. (2002). Improved stability and release control of levodopa and metaraminol using ion-exchange fibers and transdermal iontophoresis. Eur J Pharm Sci 16:273–80
- Kao HD, Traboulsi A, Itoh S, et al. (2000). Enhancement of the systemic and CNS specific delivery of l-Dopa by nasal administration of its water soluble prodrugs. Pharm Res 17:978–84
- Kulkarni U, Mahalingam R, Pather I, et al. (2010). Porcine buccal mucosa as in vitro model: effect of biological and experimental variables. J Pharm Sci 99:1265–77
- Meng-Lund E, Marxen E, Pedersen AML, et al. (2014). Ex vivo correlation of the permeability of metoprolol across human and porcine buccal mucosa. J Pharm Sci 103:2053–61
- Mercuri NB, Bernardi G. (2005). The “magic” of l-dopa: why is it the gold standard Parkinson’s disease therapy? Trends Pharmacol Sci 26:341–4
- Moffat AC, Osselton MD, Widdop B, eds. (2004). Clarke’s analysis of drugs and poisons and identification of drugs. 3rd ed. London: The Pharmaceutical Press, 1172
- Notman R, Anwar J. (2013). Breaching the skin barrier – insights from molecular simulation of model membranes. Adv Drug Deliv Rev 65:237–50
- Paderni C, Campisi G, Schumacher A, et al. (2013). Controlled delivery of naltrexone by an intraoral device: in vivo study on human subjects. Int J Pharm 452:128–34
- Pahwa R, Lyons KE. (2011). Current issues in the diagnosis and treatment of Parkinson’s disease. Introduction. Int J Neurosci 121:1–2
- Patel VF, Liu F, Brown MB. (2011). Advances in oral transmucosal drug delivery. J Control Release 153:106–16
- Rai V, Tan HS, Michniak-Kohn B. (2011). Effect of surfactants and pH on naltrexone (NTX) permeation across buccal mucosa. Int J Pharm 411:92–7
- Rao SS, Hofmann LA, Shakil A. (2006). Parkinson’s disease: diagnosis and treatment. Am Fam Physician 74:2047–54
- Rowe RC, Sheskey PJ, Quinn ME (eds). (2009). Handbook of pharmaceutical excipients. 6th ed. London: Pharmaceutical Press, 551–3
- Scholz OA, Wolff A, Schumacher A, et al. (2008). Drug delivery from the oral cavity: focus on a novel mechatronic delivery device. Drug Discov Today 13:247–53
- Senel S, Rathbone MJ, Cansız M, Pather I. (2012). Recent developments in buccal and sublingual delivery systems. Exp Opin Drug Del 9:615–28
- Thackaberry EA, Kopytek S, Sherratt P,et al. (2010). Comprehensive investigation of hydroxypropyl methylcellulose, propylene glycol, polysorbate 80, and hydroxypropyl-beta-cyclodextrin for use in general toxicology studies. Toxicol Sci 117:485–92
- Varanese S, Birnbaum Z, Rossi R, Di Rocco A. (2011). Treatment of advanced Parkinson’s disease. Parkinsons Dis 2010:480260. Doi:10.4061/2010/480260, 2010:480260, 9 pages
- Veuillez F, Kalia YN, Jacques Y, et al. (2001). Factors and strategies for improving buccal absorption of peptides. Eur J Pharm Biopharm 51:93–109
- Wan LSC, Lee PFS. (1974). CMC of polysorbates. J Pham Sci 63:136–7
- Wang YF, Zuo Z, Chen X, et al. (2010). Improving sublingual delivery of weak base compounds using pH(max) concept: application to propranolol. Eur J Pharm Sci 39:272–8
- Wright BA, Waters CH. (2013). Continuous dopaminergic delivery to minimize motor complications in Parkinson’s disease. Expert Rev Neurother 13:719–29
- Zhou T, Hider RC, Jenner P, et al. (2010). Design, synthesis and biological evaluation of l-dopa amide derivatives as potential prodrugs for the treatment of Parkinson’s disease. Eur J Med Chem 45:4035–42