Abstract
Context and objectives: Since most of developed therapeutic proteins are intended to treat chronic diseases, patients are prescribed multiple injections for long time periods, and therefore, sustained release formulations are much needed. However, challenges facing these formulations are quite significant. In this context, a model protein, lysozyme (Lys), was loaded on hydrogel microparticles (beads) and the ability of layer-by-layer (LbL) coating to control Lys release and maintain its activity over a one-month period was investigated.
Methods: LbL coating was composed of chondroitin sulfate as a negatively charged polyelectrolyte and a biocompatible, hydrolytically degradable poly β-aminoester as a positively charged polyelectrolyte. Loading distribution was monitored by fluorescence imaging, and followed by depositing a series of LbL coatings of different thicknesses. Release of Lys from these formulations was studied and activity of released fraction was determined.
Results: Lys was loaded effectively on hydrogel beads achieving about 9 mg protein/100 mg wet spheres. LbL coating was proven successful by monitoring the zeta potential of the beads, which was reversed after the addition of each layer. In vitro release studies showed sustained release profiles that depend on the thickness of the deposited coat, with t50 extended from 4.9 to 143.9 h. More importantly, released Lys possessed a high degree of biological activity during the course of release maintaining at least 72% of initial activity.
Conclusions: Successful loading of Lys and extension of its release while maintaining a considerable degree of activity might make this formulation suitable for use with other active therapeutic proteins.
Introduction
The increasing development of therapeutic proteins by pharmaceutical industry has highlighted several challenges that should be addressed carefully when deciding on a suitable formulation or delivery carrier. Among these challenges, primary obstacles are short protein biological half-life, adverse immunogenic side effects, as well as poor loading efficiency of delivery carrier reaching sub-therapeutic doses, lack of long-term stability and tissue-selectivity and uncontrolled drug release profile. Furthermore, since most of these therapeutic proteins are intended to treat chronic diseases, patients are prescribed multiple injections for long time periods, which often compromises the patient compliance and increase healthcare costs, and therefore, sustained release formulations are much needed (Frokjaer & Otzen, Citation2005; Haidar et al., Citation2008; Hammer et al., Citation2014). One promising approach to solve some of these problems would be the local release of proteins from injectable carriers that are able to control the release kinetics of their payload (Hammer et al., Citation2014). Many delivery systems have been investigated during the past few decades, and various microstructures such as liposomes, microgels, polymer micelles and nano/micropolymeric particles have been suggested as sustained release protein formulations (Ron & Bromberg, Citation1998; Pisal et al., Citation2010; Gao et al., Citation2012).
Within this context, we decided to explore the potential of layer-by-layer (LbL) technology as a coating technique to achieve sustained release profile of protein loaded on microparticulate carriers. LbL deposition is an established method for the fabrication of multicomposite ultrathin films on solid surfaces. Typically, this technique is based on the use of polyelectrolytes of opposite charges assembled layer-wise on the surface of interest, thereby building up a layered system of tunable characteristics, in terms of composition, nanometer range thickness, surface charge, permeability and elasticity. More particularly relevant to protein formulation, LbL deposition has the advantage of utilizing mild conditions (i.e. aqueous solutions), which are more favorable to preserve correct protein folding and activity in contrast to organic solvents typically employed in the fabrication of many other before mentioned protein formulations (Antipov & Sukhorukov, Citation2004; Ariga et al., Citation2007; Sakr & Borchard, Citation2013).
The majority of research work done on LbL employed synthetic polymers, mainly the anionic poly(styrene sulfonate) (PSS) and the cationic poly(allylamine)-hydrochloride (PAH). However, for pharmaceutical and biological applications, the use of biocompatible and biodegradable materials is inevitable (De Temmerman et al., Citation2011). Accordingly, many research efforts were directed to screen a vast library of natural/semisynthetic polyelectrolytes such as polysaccharides (e.g. hyaluronic acid, chondroitin, heparin and chitosan) and polypeptides (e.g. poly l-lysine, poly l-arginine, poly l-glutamic acid and poly l-aspartic acid) (Hsu et al., Citation2014). However, to achieve a more sustained and linear release profile, hydrolytic surface degradation of biocompatible and degradable polymers such as poly β-aminoesters (PBAEs) would be more promising (Macdonald et al., Citation2008). This family of polymers is attractive for this purpose due to their ability to slowly degrade via ester hydrolysis at physiologically relevant pH (Akinc et al., Citation2003), their positive charge, which allows for film construction, and because such PBAEs have been shown to be biocompatible in a number of different applications (Lynn & Langer, Citation2000; Little et al., Citation2004). Another interesting advantage of LbL films based on PBAEs is that the rate of hydrolytic destabilization can be fine-tuned depending on the hydrophobic composition of the polymer (Smith et al., Citation2009a). By modifying this factor, the drug release profile could be varied from several hours to several weeks (Macdonald et al., Citation2008; Smith et al., Citation2009b).
Several studies were published on this family of polymers by P. Hammond’s group but primarily in the form of LbL films coating solid surfaces (Wood et al., Citation2005; Macdonald et al., Citation2008; Moskowitz et al., Citation2010, Shukla et al., Citation2010). Hereby, we aim to take this one step further and investigate the potential of these biodegradable polymers in coating hydrogel microspheres. Hydrogels have been widely used in in biomedical applications as carriers for small drug molecules (Lewis et al., Citation2007) as well as biological active compounds (Hammer et al., Citation2014) due to their excellent hydrophilic properties, high swelling ratio and biocompatibility.
In this proof of concept study, market-available, sulfonated poly(vinyl alcohol)-based hydrogel beads (DC-Bead®) were used and the loading of a model protein (lysozyme, Lys) on their surface by adsorption was studied. This was followed by LbL coating with alternating layers of biocompatible and biodegradable polyelectrolytes, designed not only to slowly release the protein content, but also to prevent burst release usually encountered with protein formulations. Successful deposition of layers was followed and proven by zeta potential measurements, and the ability to fine tune the protein release from several days to several weeks was shown. Finally, the activity of the protein released from the beads was measured throughout the whole release period.
One potential application of LbL-delayed protein release from embolization beads, primarily used to treat unresectable hepatocellular carcinoma (Llovet et al., Citation2003), would be the delivery of anti-vascular endothelial growth factor (anti-VEGF) monoclonal antibodies. As a result, the angiogenic response of embolized tumor cells would be inhibited, preventing the VEGF-induced revascularization of the tumor and eventually improving the therapeutic efficiency of the embolization procedure (Liang et al., Citation2010). Reported studies in this work aim to develop a better understanding of protein interactions with embolic beads as well as to evaluate the feasibility of using LbL technology to deliver proteins in a controlled manner.
Materials and methods
Materials
DC Bead™ microspheres diameter range of 70–150 μm were obtained from Biocompatibles Ltd. (Farnham, UK) and referred to as beads. Lys from chicken egg white (14 600 Da), Micrococcus lysodeikticus bacteria and chondroitin sulfate sodium salt (CS) were obtained from Sigma Aldrich (Hannover, Germany). Lysozyme coupled to rhodamine (Lys-Rhd) was a kind gift from Capsulution Pharma (Berlin, Germany). Micro-BCA (bicinchoninic acid) Protein Assay kit was obtained from Thermo Scientific (Rhein, Germany) and used according to manufacturer’s instructions. Other chemicals were of analytical grade and were used as received without further purification. A PBAE with the structure illustrated in was chosen due to its reported degradability as well as sufficient charge density (Macdonald et al., Citation2008). The initial synthesis protocol was described by Lynn & Langer (Citation2000), synthesis conditions were even improved by Akinc et al. (Citation2003) and these latter were followed in this work. One change was the use of 1,6-heptane dioldiacrylate instead of the 1,4-butane dioldiacrylate described by the authors in order to increase the hydrophobicity of the region next to the ester bond and decrease water attack on the bond, leading to a decrease in the degradation rate of the polymer as suggested by Macdonald et al. (Citation2008).
Methods
Loading of Lys on DC Bead™
Lys solution (3 mg/ml, pH 5) was prepared in deionized water (milliQ academic, Millipore, Zug, Switzerland). To weigh the beads, the concept of wet weight was adopted, where a vial of beads suspension was mixed well, then aliquots of 1 ml suspension were transferred into preweighed tubes and beads were left to settle completely. Afterwards, supernatant was carefully removed as much as possible using a syringe, and the weight of swollen beads (wet weight) was determined. Loading studies were carried out by adding 3 ml of Lys solution to the beads followed by gentle shaking over 90 min at room temperature using a rotor shaker at 20 rpm. At preselected time points, namely 5, 15, 30, 60 and 90 min, 150 µl samples were withdrawn from the clear, beads-free supernatant and analyzed for Lys content using the micro-BCA kit, and replaced by equal amounts of deionized water. Amount of loaded Lys was calculated by subtracting residual Lys amount in the supernatant from the known added amount. All measurements were done in triplicates.
The loading efficiency was calculated as below:
while loading capacity was calculated as:
Optical and fluorescence imaging
Optical microscopy images were taken using a Nikon optiphot-2 microscope (Egg, Switzerland). For visualization purposes, rhodamine-labeled lysozyme (Lys-Rhd) was loaded on beads and fluorescence imaging was performed with a Zeiss LSM710 2P microscope (Feldbach, Switzerland) at excitation and emission wavelengths of 540/570 nm, to localize adsorbed protein and determine adsorption homogeneity.
Coating loaded beads with polyelectrolyte multilayer films
Polyelectrolytes chosen as building blocks were CS as a biocompatible negative polyelectrolyte and the synthetic hydrolytically degradable PBAE as a positive polyelectrolyte. CS was dissolved in deionized water to a concentration of 1 mg/ml, while PBAE was prepared in sodium acetate buffer (pH 5, 100 mM) at a concentration of 2 mg/ml to avoid rapid degradation (Wood et al., Citation2006). LbL build-up was carried out using a typical protocol (Ye et al., Citation2005) where 1 ml CS was added to a plastic vial containing 100 mg of beads (wet weight), followed by gentle mixing using a rotary shaker for 15 min. Then, beads were centrifuged (9.5 g, 5 min) and supernatant was removed. Excess polyelectrolyte was washed off by resuspending the beads in deionized water (pH 5), which was followed by a second centrifugation step. This washing step was repeated twice before the addition of the next polyelectrolyte layer PBAE, which was deposited using the same technique, and so on with other layers till achieving the following formulations, denoted by the letter F and number of deposited layers:
F0: [loaded beads]
F1: [loaded beads], CS
F3: [loaded beads], (CS, PBAE), CS
F7: [loaded beads], (CS, PBAE)3, CS
F13: [loaded beads], (CS, PBAE)6, CS
Similarly, a series of thicker coatings (ranging from 0 to 13 polyelectrolyte layers) on preloaded beads were prepared to study the effect of increasing LbL coating on the loss of preloaded Lys and resulting release profiles (). All washes were carefully collected in separate vials and analyzed for loss of preloaded Lys during the LbL process. Coated beads were kept in 1 ml of deionized water until further use.
Zeta potential measurements
Successful deposition of polyelectrolyte multilayers was followed by measuring the microelectrophoretic mobility of coated particles after addition of each polylectrolyte layer using a Malvern Zetasizer 3000 HAS (Malvern, Lausanne, Switzerland). All measurements were performed in deionized water (pH 5) without any added electrolyte.
Scanning electron microscopy (SEM)
Changes in particle surface morphology were observed by SEM using a Jeol electron microscope (JSM-7500F, Eching, Germany) at an accelerating voltage of 5 kV. Prior to observations, samples were mounted on metal stubs, using carbon-conductive double-sided adhesive tape, and coated with a 10-nm gold layer under vacuum prior to imaging (Leica EM SCD500, Heerbrugg, Switzerland).
In vitro drug release studies
In vitro drug release studies were carried out to investigate the ability of deposited layers to control the release profile of Lys loaded on beads. Briefly, coated beads of each formulation stored in 1 ml water were carefully transferred into a 15-ml Falcon tube, supernatant removed and 5 ml of PBS pH 7.4 were added. The tubes were horizontally fixed to an orbital shaker rotating at 80 rpm and incubated at 37 °C. This setup maintained the beads suspended and kept them from getting stuck together at the pointed bottom of the tube. At predetermined time points, tubes were centrifuged (9.5 g, 5 min) and the whole medium was aspirated as much as possible without touching the beads and directly replaced with fresh release medium kept at the same temperature. Samples were directly analyzed for Lys content using the micro-BCA kit and percentage of cumulative release was plotted against time. All experiments were carried out in triplicates.
Biological activity
Activity of Lys released from the slowest release formulation (F13) was tested using the decrease in optical density at 450 nm (A450) of a M. lysodeikticus suspension, adopting a modified literature method (Lee & Yang, Citation2002) that was applied using a microplate reader (Power Wave XS, Bio-Tek Instruments, Colmar, France). Briefly, 9 mg bacterial cells were suspended in 30 ml of 100 mM potassium phosphate, pH 6.2, shortly before the assay so that the suspension had an A450 between 0.2 and 0.6. To one of the 48-microplate wells, 50 µl Lys sample and 200 µl of the bacterial cell suspension was added. The plate was gently shaken for 5 s, and measurement of A450 nm was commenced after 5 s and continued for 2 min at 20 s intervals. The obtained A450 nm values were plotted as a function of time. The activity of Lys was calculated from the slope of the time course by linear regression of data points. A unit of enzyme was defined in this study as the quantity of enzyme causing a decrease in absorbance of 0.001 min−1, under mentioned set of specified conditions. Thus, the following equation was adopted:
For control purposes and to study the ability of LbL coating to preserve Lys activity, a portion of 3 mg Lys, resembling the amount of Lys loaded per formulation, was added to 5 ml PBS, resembling the total volume of release medium, and the vial was incubated as other release vials and was subjected to the same temperature and shaking conditions.
It is worthy to mention that Lys activity unit measured in a 48-well plate is different from the standard activity unit measured in a normal cuvette with a path length of 1 cm, and therefore numerical values cannot be compared. Results are plotted as percentage decrease in activity over the release period of time.
Statistical analysis
All experiments were performed in at least three independent assays. Data are summarized as mean ± standard deviation (SD). Statistical analysis of the results was performed by Student’s t-test for unpaired samples. A p value of <0.05 was considered as statistically significant.
Results and discussion
Loading of Lys on DC Bead™
Lys was loaded successfully and efficiently on DC bead™ microspheres, with about 90% adsorbed in the first 15 min, reaching almost 100% loading efficiency and 9 mg/100 mg wet beads loading capacity in 60 min. These results can be directly attributed to ionic interactions between oppositely charged species under working conditions (pH 5), as the beads were negatively charged due to the presence of sulfonate groups on their surface (Lewis et al., Citation2007), while Lys (pI = 11) was positively charged. Exposure to lower protein concentrations (data not shown) leads to a similar rapid uptake with final/equilibrium bead load proportional to the initial protein concentration.
Fluorescence imaging
Fluorescence microscopy was used to image 70–150 µm beads loaded with labeled Lys-Rhd. shows an even distribution of the protein on the surface of the microsphere with no visible inhomogeneity. Fluorescence is concentrated in the outer 10–20 µm of the beads structure. This localization was attributed to the presence of a much denser cross-linked layer with higher charge density on the beads surface, which has about the same 20 µm thickness and is more likely to entrap cationic drugs (Lewis et al., Citation2007).
Coating of loaded beads with polyelectrolyte multilayer films
Successful layer deposition was proven by the reversal of external surface charge with the addition of each polyelectrolyte layer. illustrates this phenomenon where the charge of the underlying layer is over-compensated by the next layer applied. Starting with negatively charged beads, the adsorption of Lys led to a slight increase in zeta potential that was not enough to reverse the charge. With more layers being deposited, the cycles of charge overcompensation became bigger up to layer number 6, after which charge converged toward stable values. These results suggest that the underlying negative charge of the substrate was not masked completely with the addition of the first couple of bilayers, and that surface coverage by deposited polyelectrolytes was not complete. However, only after 6 layers the bead surface was covered enough and surface charge was attributed only to the coating polymer. This assumption agrees with the suggestion by Buron et al. (Citation2007) that layer deposition in the early phase (first 2–3 bilayers) is governed by the underlying substrate, while afterwards, the effect of substrate diminishes and surface properties are ruled only by the deposited polyelectrolytes.
Figure 4. Reversal of zeta potential of beads with addition of successive polyelectrolyte layers (n = 6, error bars represent SDs).
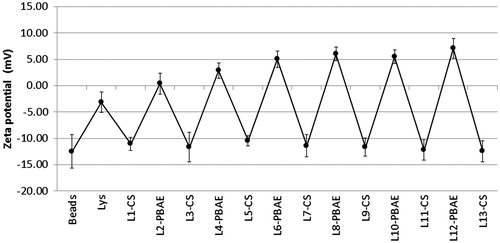
The last layer was always kept negative in all formulations. The reason behind this is that beads were found to shrink (appeared denser and darker in color) and tend to aggregate when the outer layer was positive (). Interestingly, beads were able to restore their diameter when the next negatively charged layer was deposited. This behavior might be attributed to hydrogel ionic equilibrium. Upon negative zeta potential and thus superficial charge, the inner sulfonated hydrogel matrix is expected to swell. In contrast, net positive charge is expected to interact with hydrogel matrix peripheral layers, decreasing intra-hydrogel ionic repulsive forces resulting in water expel and bead shrinking.
Figure 5. Optical microscopy images of beads (a) with negative outer surface, (b) with positive outer surface (each major mark indicates a distance of 50 µm).
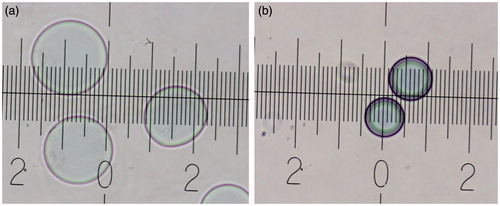
The change in bead surface morphology after layer deposition was examined using SEM imaging. Beads before coating () showed a smooth continuous surface. After 13 layers (), surface became covered with random depositions of polyelectrolytes. However, even after 13 layers, the surface was not fully covered, but since loading was enough, and the achieved release profile was satisfactory (see “In vitro release studies” section), there was no need to deposit more layers and achieve full coating. The term LbL coating might give the false impression to be a smooth regular coat as one would think of a film coat, which is not the case. LbL coating typically produces random depositions on the surface that grow with time (), resulting in an uneven surface covered with distinguishable features. This has been reported in other studies visualizing layer deposition using AFM (Buron et al., Citation2007; Lin et al., Citation2009).
Figure 6. SEM images showing (a) beads before coating and (b) after coating with 13 layers (F13). Scale bar = 10 µm.
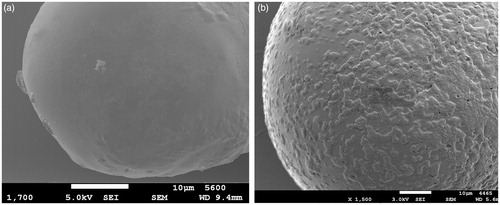
Furthermore, it was important to determine how much Lys was lost during the LbL coating procedure, which involved quite an exposure to aqueous solutions. During the coating process, care was taken to use only salt-free solutions to minimize any possible premature release of Lys. illustrates the loss of Lys during the LbL coating process. In brief, loss of Lys was limited to about 16 ± 1.7% after addition of 13 layers. No significant loss was encountered after addition of one layer of CS, while significant changes started to occur with the addition of PBAE layers. Interestingly, loss in Lys almost leveled off after seven layers, as no significant difference was found between Lys loss in F7 (7 layers) and F13 (13 layers), which indicates that preloaded Lys was sufficiently covered by deposited layers such that Lys leakage did not occur anymore with further exposure to water. However, due to the high loading capacity of the beads, this limited loss is not considered to be critical to the whole formulation strategy.
In vitro release studies
illustrates release profiles of Lys loaded on beads and coated with different thicknesses of polyelectrolyte multilayers, followed for 30 days. In the following discussion, special attention is paid to the effect of change in formulation on burst release over the first 2 h and after 1 day, as well as the effect on rate and extent of drug release. F1, representing the control formulation (no LbL coat), showed a burst release of loaded Lys into the release medium (PBS), where about 48% were released during the first day and up to 55% in the next day, after which release leveled off. Since Lys is adsorbed on the bead’s surface mainly due to ionic interactions, this burst release can be attributed to ionized salt molecules in PBS competing with adsorbed Lys molecules and eventually releasing them from the surface. However, since about half of the loaded protein amount remained adsorbed to the beads, this may indicate that the ionic strength of the PBS was not sufficient to overcome all ionic interactions and release all Lys molecules.
As illustrated in , the addition of one layer of CS (F1) did not cause any significant effect, neither on the rate nor release extent of drug release. However, the introduction of the first bilayer of CS-PBAE (F3) led to a significant decrease in three aspects: burst release decreased to 9% over 2 h and only 32% released during the first day. In addition, both release rate and extent were significantly decreased. The same tendency was found with the addition of three CS-PBAE bilayers (F7), with a further decrease of burst release to 3% and 17% after 2 h and 1 day, respectively. Interestingly, the addition of 6 CS-PBAE bilayers (F13) inhibited release completely over the first 2 h and as little as 3% were released during the first day, which was followed by a more or less steady linear release for 24 days that leveled off at about 30% of loaded amount.
Table 1. Different release parameters of investigated formulations.
To elaborate, PBS would flow through the network of deposited multilayers, where salt molecules would compete with the ionic interactions between bead surface and Lys leading eventually to the release of Lys molecules. Released Lys molecules will have to diffuse through the LbL network to reach the bulk of the release medium, yet spaces between entangled polyelectrolyte fibers allow only for the diffusion of low molecular weight compounds (Pavlukhina & Sukhishvili, Citation2011). With time, PBAE molecules start to undergo hydrolytic degradation and the multilayer network starts to erode, creating bigger voids and allowing for the release of Lys molecules. The obtained results show clearly a direct dependence of the release rate and extent on number of layers with a strong effect even after deposition of the three first layers. This finding can be beneficial in terms of enabling relatively accurate prediction of release profile as a function of number of deposited layers. It is important to mention that the ionic strength of PBS is not sufficient to destroy ionic interactions between alternating layers as this needs a salt concentration of 0.6–5 M (Wood et al., Citation2005). Therefore, surface erosion of degradable PBAE molecules is the limiting factor for the destruction of LbL film and release of protein molecules.
Moreover, the reason behind incomplete release was investigated by addition of an excess of 5 M NaCl to the coated beads after the full release period in order to obtain full Lys release. However, no further Lys release was detected, which may indicate that some Lys molecules got entangled physically between the polymer network chains of the bead during the loading and coating steps. Lys retention thus appeared to be independent of ionic binding.
This release mechanism is highly valuable for drug delivery applications, because it allows for constant, low levels of protein to be released during a longer span of time, in a controlled, easy to predict fashion, compared to burst release profiles in which most of the protein is released instantly and is therefore lost to a greater distribution volume and cleared before therapeutic action can take place. However, caution must be taken with extending the utility of these results too far, due to the idealized simulations of body conditions that lack enzymes, cellular reactions, and complex mass transfer profiles likely to be encountered in vivo. Still, the linear release trend is encouraging and granting further in vivo investigations.
Biological activity
Lys catalyzes the hydrolysis of glycosidic bonds of muramic acids in microbial cell wall polysaccharides. This functionality was taken as a base for many Lys biological activity assays, where one can detect the amount of functional protein in solution by a kinetic reduction in turbidity of a bacterial solution (Lee & Yang, Citation2002; Macdonald et al., Citation2008). illustrates the activity of Lys released from the formulation of slowest release rate (F13) compared to a control vial containing the same loaded amount of Lys dissolved in PBS 7.4 used as a release medium. Results are given as relative percentage of activity of Lys freshly dissolved in PBS. Obviously, Lys in the control vials encountered a continuous degradation of activity as only 35% activity was still present after 24 days, accompanied by excessive aggregation and fibrils formation. On the other hand Lys released from LbL-coated microspheres was 10% less active than fresh Lys in the first day, but activity decay rate was much slower and Lys released after 24 days maintained about 72% of its original activity.
Figure 9. Activity of Lys released from slow release formulation (F13) compared to activity of Lys in PBS (control) subjected to the same condition and expressed as relative activity % in comparison to freshly dissolved Lys in PBS. Corner image shows Lys fibril aggregations in control solution after 24 days (n = 3, error bars represent SDs).
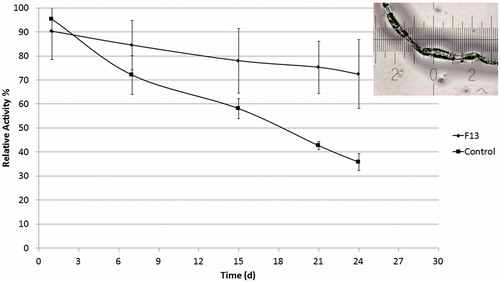
Indeed, preserving protein activity is one of the tough challenges facing protein delivery in general and sustained release formulations in particular. Beside obtaining a desirable release, it is critical to maintain activity of released protein as much as possible. In this context, LbL coatings offer interesting advantages such as the sole use of aqueous solutions and avoidance of organic solvents, mild formulation conditions as no temperature or physical pressure needed which are more favorable to preserve fragile protein folding and activity (Sakr & Borchard, Citation2013). It has been proven that entrapped water remains in LbL films, in spite of applied drying procedures, which is important for the preservation of activity of biomolecules (Moraes et al., Citation2009).
Conclusions
This report addressed the issue of sustained protein delivery from microparticles. The main objective was to investigate the ability to load and slowly release an active protein on hydrogel beads while maintaining its activity. Lysozyme was chosen as a model protein in this proof of concept study, to pave the way for other more therapeutically relevant proteins. Microspherical beads were effectively loaded with Lys, and LbL coating with alternating layers of biocompatible and biodegradable polyelectrolytes was successfully carried out, not only to slowly release the protein content, but also to prevent burst release usually encountered with most protein formulations. In vitro drug release testing showed a direct relation between the coating thickness and the suppression of release rate and extent. Examining the activity of released fractions indicated the ability of LbL coating to preserve a reasonable percentage of the protein activity and protect it from aggregation. Further investigations are required to better understand the mechanisms of the protein release process and the relative contribution of diffusion-controlled versus biodegradation-controlled sustained release. However, data presented here are justifying more research work involving a wide range of therapeutic proteins differing in size and conformational structures.
Acknowledgements
The authors are indebted to Ms. Katrin Fuchs for enriching comments and critical discussions as well as for the fluorescence imaging. Thanks are also extended to Mr. Tayeb Jbilou for SEM photos and lab support.
Declaration of interest
Authors have no declarations of interest to report.
References
- Akinc A, Anderson D G, Lynn DM, Langer R. (2003). Synthesis of poly(beta-amino ester)s optimized for highly effective gene delivery. Bioconjug Chem 14:979–88
- Antipov AA, Sukhorukov GB. (2004). Polyelectrolyte multilayer capsules as vehicles with tunable permeability. Adv Colloid Interface Sci 111:49–61
- Ariga K, Hill JP, Ji Q. (2007). Layer-by-layer assembly as a versatile bottom-up nanofabrication technique for exploratory research and realistic application. Phys Chem Chem Phys 9:2319–40
- Buron CC, Filiatre C, Membrey F, et al. (2007). Early steps in layer-by-layer construction of polyelectrolyte films: the transition from surface/polymer to polymer/polymer determining interactions. J Colloid Interface Sci 314:358–66
- De Temmerman ML, Demeester J, De Vos F, De Smedt SC. (2011). Encapsulation performance of layer-by-layer microcapsules for proteins. Biomacromolecules 12:1283–9
- Frokjaer S, Otzen DE. (2005). Protein drug stability: a formulation challenge. Nat Rev Drug Discov 4:298–306
- Gao GH, Park MJ, Li Y, et al. (2012). The use of pH-sensitive positively charged polymeric micelles for protein delivery. Biomaterials 33:9157–64
- Haidar ZS, Hamdy RC, Tabrizian M. (2008). Protein release kinetics for core-shell hybrid nanoparticles based on the layer-by-layer assembly of alginate and chitosan on liposomes. Biomaterials 29:1207–15
- Hammer N, Brandl FP, Kirchhof S, Goepferich AM. (2014). Cleavable carbamate linkers for controlled protein delivery from hydrogels. J Control Release 183:67–76
- Hsu BB, Hagerman SR, Jamieson K, et al. (2014). Multilayer films assembled from naturally-derived materials for controlled protein release. Biomacromolecules 15:2049–57
- Lee YC, Yang D. (2002). Determination of lysozyme activities in a microplate format. Anal Biochem 310:223–4
- Lewis AL, Gonzalez MV, Leppard SW, et al. (2007). Doxorubicin eluting beads – 1: effects of drug loading on bead characteristics and drug distribution. J Mater Sci Mater Med 18:1691–9
- Liang B, Zheng CS, Feng GS, et al. (2010). Correlation of hypoxia-inducible factor 1alpha with angiogenesis in liver tumors after transcatheter arterial embolization in an animal model. Cardiovasc Intervent Radiol 33:806–12
- Lin QK, Ren KF, Ji J. (2009). Hyaluronic acid and chitosan–DNA complex multilayered thin film as surface-mediated nonviral gene delivery system. Colloids Surf B Biointerfaces 74:298–303
- Little SR, Lynn DM, Ge Q, et al. (2004). Poly-beta amino ester-containing microparticles enhance the activity of nonviral genetic vaccines. Proc Natl Acad Sci USA 101:9534–9
- Llovet JM, Burroughs A, Bruix J. (2003). Hepatocellular carcinoma. Lancet 362:1907–17
- Lynn DM, Langer R. (2000). Degradable poly(b-amino esters): synthesis, characterization, and self-assembly with plasmid DNA. J Am Chem Soc 122:10761–8
- Macdonald M, Rodriguez NM, Smith R, Hammond PT. (2008). Release of a model protein from biodegradable self assembled films for surface delivery applications. J Control Release 131:228–34
- Moraes ML, De Souza NC, Hayasaka CO, et al. (2009). Immobilization of cholesterol oxidase in LbL films and detection of cholesterol using ac measurements. Mater Sci Eng C 29:442–7
- Moskowitz JS, Blaisse MR, Samuel RE, et al. (2010). The effectiveness of the controlled release of gentamicin from polyelectrolyte multilayers in the treatment of Staphylococcus aureus infection in a rabbit bone model. Biomaterials 31:6019–30
- Pavlukhina S, Sukhishvili S. (2011). Polymer assemblies for controlled delivery of bioactive molecules from surfaces. Adv Drug Deliv Rev 63:822–36
- Pisal DS, Kosloski MP, Balu-Iyer SV. (2010). Delivery of therapeutic proteins. J Pharm Sci 99:2557–75
- Ron ES, Bromberg LE. (1998). Temperature-responsive gels and thermogelling polymer matrices for protein and peptide delivery. Adv Drug Deliv Rev 31:197–221
- Sakr OS, Borchard G. (2013). Encapsulation of enzymes in layer-by-layer (LbL) structures: latest advances and applications. Biomacromolecules 14:2117–35
- Shukla A, Fleming KE, Chuang HF, et al. (2010). Controlling the release of peptide antimicrobial agents from surfaces. Biomaterials 31:2348–57
- Smith RC, Leung A, Kim BS, Hammond PT. (2009a). Hydrophobic effects in the critical destabilization and release dynamics of degradable multilayer films. Chem Mater 21:1108–15
- Smith RC, Riollano M, Leung A, Hammond PT. (2009b). Layer-by-layer platform technology for small-molecule delivery. Angew Chem Int Ed Engl 48:8974–7
- Wood KC, Boedicker JQ, Lynn DM, Hammond PT. (2005). Tunable drug release from hydrolytically degradable layer-by-layer thin films. Langmuir 21:1603–9
- Wood KC, Chuang HF, Batten RD, et al. (2006). Controlling interlayer diffusion to achieve sustained, multiagent delivery from layer-by-layer thin films. Proc Natl Acad Sci USA 103:10207–12
- Ye S, Wang C, Liu X, Tong Z. (2005). Deposition temperature effect on release rate of indomethacin microcrystals from microcapsules of layer-by-layer assembled chitosan and alginate multilayer films. J Control Release 106:319–28