Abstract
Intravesical drug delivery is the main strategy for the treatment of bladder disorders. To reduce the relief arising from frequent intravesical instillation, mucoadhesive hydrogel was used for the controlled release of the drug. However, the viscosity of mucoadhesive gel might cause severe urinary obstruction and bladder irritation. To solve all these problems, a floating hydrogel delivery system was developed using perfluoropentane (PFP) as the floating agent. After intravesical instillation of the floating hydrogel, the increased temperature in bladder vaporized PFP, resulting in the generation of microbubbles in the hydrogel. Then, it can float in urine to avoid the urinary obstruction and bladder irritation. In this study, systematic experiments were conducted to investigate the influences of PFP vaporization on the morphology and floating ability of hydrogels. The floating process is much milder and safer than other floating methods published before. In addition, PFP had been used as contrast agent, which affiliated the monitoring of gels during the operation. Therefore, this new drug delivery system addresses the problems of conventional intravesical instillation and is promising for clinic use.
Introduction
Bladder cancer is one of the most common cancers worldwide. In 2014, 74 690 bladder cancer cases and 15 580 deaths were estimated in the United States, occupying 4.5% and 2.6% of all cancer cases, respectively (GuhaSarkar & Banerjee, Citation2010). Approximate 70% of bladder cancers are superficial, which could be managed by transuretheral resection (TUR) (Siegel et al., Citation2014). However, 50–70% of superficial bladder cancer recurs within 5 years after TUR and 20–30% of recurrences progress to higher stage even when chemical and/or immunologic agents were administrated intravesically after TUR (Cima et al., Citation2014).
Drug dilution caused by continuous urine formation and voiding of most drugs during urination few hours after instillation are main challenges of intravesical instillation (GuhaSarkar & Banerjee, Citation2010). Nanoparticles and in situ gels have been developed to solve these problems (GuhaSarkar & Banerjee, Citation2010). Nanoparticles can realize the control release of drug and hence minimize the drug dilution by continuous urine formation (Fraser et al., Citation2003; Lu et al., Citation2004; Tyagi et al., Citation2004a; Chang et al., Citation2009). For example, Lu et al. delivered hydrophobic drug paclitaxel (PTX) by gelatin nanoparticles to treat bladder cancer. Results showed that the PTX concentration in urothelium and lamina propria was about three times better than that of free PTX in Cremophor/ethanol (Lu et al., Citation2004). Chang et al. used poly(ethyl-2-cyanoacrylate) (PECA) to encapsulate epirubicin for intravesical installation. Histological data showed that the EPI-NP formulation caused no structural damage on the urothelium, and tissue analysis also proved that the EPI-NP formulation achieved better penetration and accumulation in bladder tissues than free epirubicin (Chang et al., Citation2009). Even the nanoparticle formulation successfully conquers the drug dilution by urine, most nanoparticles are eliminated from the bladder due to voiding quickly after intravesical installation, resulting the limited efficacy. To solve this problem, mucoadhesive formulations including chitosan, carbomers and cellulose derivatives have been developed to extend residence of drug in bladder (Tyagi et al., Citation2004b; Men et al., Citation2012; Zhang et al., Citation2013). For example, Men et al. encapsulated deguelin into 1, 2-dioleoyl-3-trimethylammonium-propane (DOTAP) and methoxypoly(ethyleneglycol)-poly(ɛ-caprolactone) (MPEG-PCL) hybrid nanoparticles to enhance the anticancer efficacy of deguelin in vitro. Then, the nanoparticles were incorporated into thermo-sensitive F127 hydrogel for intravesical installation. Results indicated that this F127 hydrogel system could extensively extend the drug residence time and increase the drug concentration in the bladder (Men et al., Citation2012). However, the viscosity of gels might cause urinary obstruction, limiting the further clinical application and since the formulations attach on the bladder wall, another problem may be bladder irritation symptoms, just like blood clots in bladder (Collado et al., Citation2000).
To cover these two challenges of intravesical instillation, floating hydrogel based on thermo-sensitive polymer Poloxamer 407 (P407) as matrix has been developed to realize both the control release of drug and extend the resident time in bladder (Lin et al., Citation2014a,Citationb). The incorporation of NaHCO3 and NH4HCO3 into thermo-sensitive hydrogel could generate microbubbles and make the hydrogel float in urine, avoiding the voiding of the most drugs during urination. However, the decomposition of NaHCO3 requires acid environment that is different from the environment of urine. Furthermore, NH4HCO3 is unstable, so hydrogel cannot be restored for a long time. Moreover, NaHCO3 and NH4HCO3 are inorganic compounds that could react with drugs in gel and affect the drug efficiency.
In the present study, a floating hydrogel delivery system was developed based on P407 and perfluoropentane (PFP). Since the boiling point of PFP is 29.2 °C, much lower than body temperature (37 °C), PFP converts to gas state in the bladder and swells the gels, resulting in the floating of the gel in the urine. Furthermore, PFP is biologically inert and widely used as a contrast agent for diagnosis (Krafft, Citation2001). In our study, the hydrogel could be detected by using B-scan ultrasonography, suggesting the tracking and imaging role of PFP for intravesical installation. In vivo data demonstrated that the drug delivery efficiency of the floating hydrogel was much higher than free drugs and the non-floating hydrogels. PFP therefore acts as both floating and imaging agent in our floating hydrogel system, facilitating clinic monitoring during the operation.
Materials and methods
Materials
P407 was purchased from Meilun Biology Technology Company, Ltd. (Dalian, Liaoning, PR China), Doxorubicin (DOX) was also purchased from Meilun Biology Technology Company, Ltd. (Dalian, Liaoning, PR China) and PFP was purchased from Strem Chemicial Company (Newburyport, MA). Thirty New Zealand white male rabbits, weighting 2000 g in average (ranging from 1800 to 2500 g), were purchased from the Experimental Animal Center, University of Yangzhou, China. All animal experiment procedures were ratified by Institutional Animal Care and Use Committee (IACUC) of Nanjing University.
Preparation of hydrogel
The non-floating hydrogel is the mixture of P407 and distilled water, and the P407 concentration was 45% (w/v). To prepare the floating hydrogel, PFP was added into the P407 solution and kept in the ice bath. The mixed solution was emulsified by ultrasound at different time. To verify the density of microbubbles, hydrogel was dripped on a glass slide and observed using a Zeiss M2Bio microscope (New York, NY). The zoom of microscope was 10×, and images were captured.
Influences of PFP concentration on the hydrogel
To obtain the information on the pore structure of hydrogels, the floating hydrogel was observed using a Hitachi (Tokyo, Japan) S-570 Scanning Electron Microscope (Ilic-Stojanovic et al., Citation2013). The samples were plunged in liquid nitrogen and freeze-dried to maintain the porous structure without any collapse. The samples were mounted on the base plate and coated with gold. To evaluate the floating ability, 5 mL P407 solution with 0%, 5% and 10% PFP was injected into 37 °C water, and the time to float and erosion time of floating hydrogels were recorded. The time to float was the time it took for the gel to float in the solution. The erosion time was the time it took for the gel to dissolve completely in water. To evaluate the gel volume, 2 mL gel solution P407 solution with PFP was dripped on the dish. Then, the gel volume was recorded at 0, 2 and 8 h. The final concentration of DOX was 0.05% (w/v).
Release study in vitro
To investigate the drug release of hydrogel, DOX was incorporated into the hydrogel. A calculated amount of P407 (45%) was successively dissolved in DOX solution at 4 °C to form the DOX-loaded hydrogel solution. The release study in vitro was performed in the dissolution tester (ZRS-8G, TDTF, China). The PBS (pH 7, 100 mL) in the beaker of dissolution tester was heated to 37 °C. A 5 mL P407 and DOX solution with 0%, 5% and 10% PFP was injected into PBS, respectively. At predetermined time points, 3 mL solution was collected and replaced with the same volume of fresh PBS. The amount of DOX was measured by UV spectrophotometry at 480 nm.
Floating state in vivo
Rabbits were anesthetized with pentobarbital (30 mg/kg, intravenous injection). P407 solution was intravesically instilled into bladder using a catheter. The hydrogels in the bladder was detected via B-scan ultrasonography. The rabbits were randomly chosen. They were maintained in a controlled atmosphere of 12 h dark/light cycle, 22 ± 2 °C temperature and 50–70% humidity, with free access to pellet feed and fresh tap water.
Release study in vivo
The rabbits were supplied with dry food pellets. All rabbits were fastened on a desk for intravesical instillation. Rabbits were intravesically instilled with DOX-loaded P407 solution (0% or 5% PFP). Intravesical administration was performed by inserting a catheter (9F) into the bladder through the urethra. Afterwards, the urine samples of rabbits were collected. The concentration of DOX was determined by a fluorescence microplate reader. The frozen section was prepared immediately after isolating rabbit bladder tissues. The bladder section was observed via a Zeiss M2Bio fluorescence microscope. The frozen biopsy was 10 μm thick, and the exposure time was 1 s.
Results and discussion
Preparation of floating hydrogel consisting of P407 and PFP
Our floating hydrogel system is schematically illustrated in . The constituents of the system are introduced as a mixture of P407 and nanodroplets that are formed by PFP. The optimal concentration of P407 is 45% (w/v) according to our previous work (Lin et al., Citation2014a). P407 is a polyoxyethylene–polyoxypropylene–polyoxyethylene (PEOn–PPOn–PEOn) tri-block copolymer. As a non-ionic surfactant, P407 is biocompatible, low toxicity and weak immunogenic, with no safety concern for clinic use (Dumortier et al., Citation2006). Most importantly, P407 is thermo-sensitive: it is solution at low temperature, and forms gel at body temperature. So it is possible to inject P407 solution into the bladder before it forms gel, and then P407 solution forms gel in urine. Once injected into the bladder, PFP nanodroplets encapsulated in the gel vaporize inside the gel at physiological temperature, resulting in conversion of the nanodroplets into nano- and/or microbubbles (Rapoport et al., Citation2007). The generation of microbubbles swells the gel and hence floats it in the urine. In our paper, influence of PFP microbubbles generation on the morphology and floating properties of hydrogels was systematically investigated.
Influences of PFP concentration on the hydrogel morphology and volume
The morphology of hydrogels of 0%, 5% and 10% PFP was investigated using microscope, ultrasound imaging and scanning electron microscope (SEM), as shown in . The microbubble density of 10% PFP gels doubled than that of 5% PFP gels (). PFP microbubbles could produce strong echo in ultrasound imaging. The ultrasound images manifested the contrast ability of PFP: the 5% and 10% PFP hydrogels were quite bright and clear (). This image indicated that PFP had been encapsulated into the gels. consists of images obtained with SEM, which depicted the porous structure of hydrogels. The micro-pore density correlated well with the microbubbles density in .
Figure 2. Characterizations of floating hydrogel. (a) Bubbles in hydrogels under microscope; (b) bubbles in hydrogel solution detected by ultrasound and (c) bubbles in hydrogel solution detected by scanning electronic microscope.
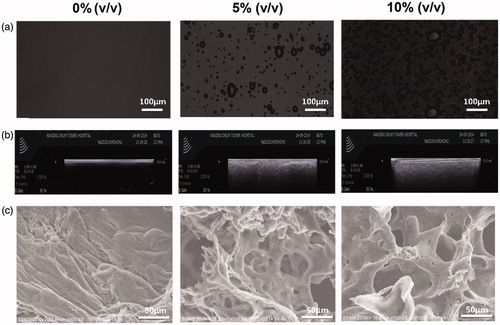
Then we evaluated the gel volume changes with the vaporization of PFP (). We can see that the gels swelled during the eight hours of vaporization of PFP. The 5% PFP gel and 10% PFP gel swelled to about 7 and 10 mL in 8 h, respectively. So the volume of gels was influenced greatly by the concentration of PFP, because more PFP indicated more microbubbles, which enlarged the volume of hydrogels.
Figure 3. Change of gel volume with the perfluoropentane of different concentration. (a) Swelling process of gels encapsulating 0%, 5% and 10% PFP recorded by camera at 37 °C; (b) histogram of the volume changes; (c) swelling process of gels encapsulating 0%, 5% and 10% PFP recorded by camera at 20, 30, 37 °C; (d) histogram of the volume changes.
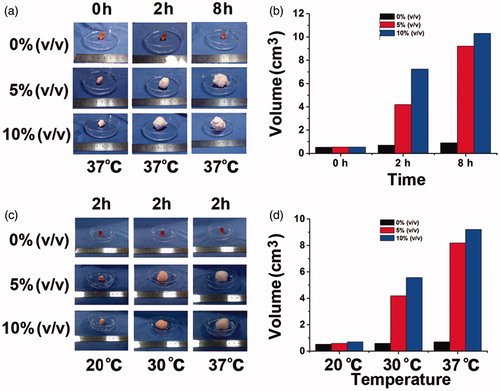
In contrast to the chemical decomposition of NaHCO3 and NH4HCO3, the floating ability of our present floating hydrogel stemmed from the vaporization of PFP, so this process was influenced by temperature. From the initial preparation of gels to the injection of gels into the bladder, the environment temperature varied from 20 to 37 °C. The boiling point of PFP was 29.2 °C, right in this temperature range. Therefore, we also investigated the changes of gel properties including volume at 20, 30 and 37 °C. shows that both the 5% PFP gel and 10% PFP gel rarely swelled at 20 °C. The 5% PFP gels had swelled in 2 h to 4 mL at 30 °C and 6 mL at 37 °C, respectively, while the 10% PFP gel had swelled to 8 mL at 30 °C and 9 mL at 37 °C, respectively. So the temperature did influence the volume of gels, but only to a small extent.
Influences of PFP concentration and temperature on the floating process of hydrogels
Next, we evaluated the impact of PFP vaporization on the floating process of floating hydrogels. To characterize this process, we measured the time to float and erosion time of hydrogel, as defined in the “Methods” section. Because hydrogel should float instantly after injected into the bladder to reduce the potential of urinary obstruction. In addition, since the release of the drug was terminated once hydrogels completely dissolved in water, the erosion time should be longer to enhance the drug efficacy.
Theoretically, the high density of microbubbles indicated better vaporization of PFP and hence shorter time to float for hydrogels. To verify this, we evaluated the time to float of hydrogels with 0%, 5% and 10% PFP (). Gels with 0% PFP failed to float, and the time to float of 5% and 10% PFP gels were about 1.7 and 0.3 min, respectively. So the 10% PFP gels achieved the shortest time to float. The time to float of gels was also influenced by the environment temperature, since the floating ability was correlated positively with gel volume (). The gels failed to float at 20 °C, but gels containing either 5% or 10% PFP at 30 °C could float within 6 and 3 min, respectively, and the time to float was shortened to 2 and 0.5 min at 37 °C, respectively. The results indicated that the time to float was shortened by increasing the PFP concentration, because PFP concentration was positively correlated with gel volume, as demonstrates. So the buoyancy force on gels was increased to make the hydrogel float more quickly.
Figure 4. (a) Floating process of gels encapsulating 0%, 5% and 10% PFP recorded by camera at 37 °C; (b) time to float of gels at 37 °C; (c) erosion time of gels at 37 °C; (d) time to float of gels at 20, 30 and 37 °C.
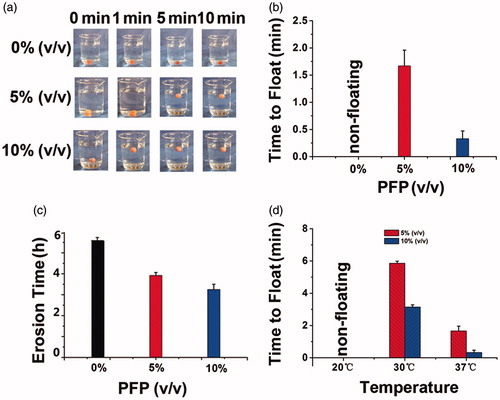
As to the erosion time, the results showed that the 0% PFP gel dissolved completely in the water about 5.7 h, and the time of 5% and 10% PFP gels were 3.8 and 3.3 h, respectively (). To explain this phenomenon, we should refer to the SEM images of , where microbubbles made the hydrogel porous. The porous and loose structure would enlarge the contact area of the gel and solvent and therefore shorten the erosion process (Ilic-Stojanovic et al., Citation2012).
Release study in vitro
Drug release influences the drug efficacy of a drug delivery system, so we measured the release profile of DOX in the PBS. depicts the cumulative amount of DOX released as a function of time in vitro to demonstrate its release behavior. Once injecting hydrogels into the PBS, hydrogels of 5% or 10% PFP floated in the water immediately, whereas the gel without PFP sunk in the beaker throughout the releasing process. As the graph shows, the time for DOX to be completely released from gel of 5% and 10% PFP was 4 and 3 h, respectively. The time was equivalent to the erosion time of hydrogels, because the drug release was synchronous with erosion of hydrogels. So lower concentration of PFP could extend the drug release time in vitro by prolonging the erosion time of gels.
Figure 5. Cumulative release of DOX-loaded non-floating hydrogel and DOX-loaded floating hydrogel in vitro (mean ± SD, n = 3).
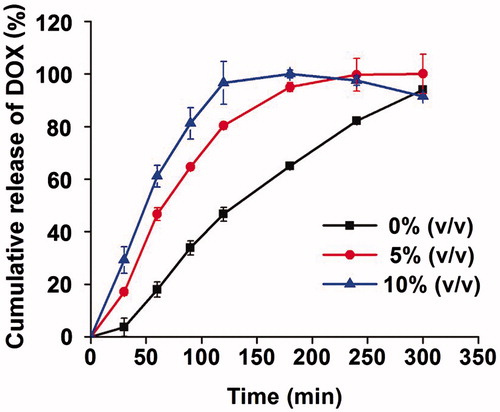
Since the P407 consists of a large population of micelles in aqueous phase, the incorporated drug was released by diffusion through gel matrix. Drug release correlates well with P407 dissolution and the drug release is controlled by erosion of the gel (Zhang et al., Citation2002). The Higuchi model (Qt = KH√t) could predict the drug release from floating hydrogel perfectly in which the correlation coefficient (R) is 0.91. It indicated that the drug release from floating hydrogels was in a controlled manner and square root time dependent.
Verification of hydrogel floating in vivo
We then depict the floating of hydrogels in vivo. The intravesical injection of 5% PFP hydrogel into rabbit bladder was detected by ultrasound (). The white dashed curves in the pictures outlined the rabbit bladder wall and gels in the rabbit bladder. According to the images, the hydrogel without PFP failed to float in urine and adhered to the bladder wall. Whereas, the hydrogel of 5% PFP was able to float in the urine 2 min after the injection into the bladder, corresponding to the time to float of hydrogels ().
Release study in vivo
describes the DOX release profile of hydrogels of 0% and 5% PFP in the rabbit bladders. The first urination was triggered by brimming the bladder with normal saline 2–10 min after intravesical instillation with 0% PFP or 5% PFP hydrogel solution. The non-floating hydrogel failed to float and adhered to the bladder wall, so it caused serious bladder irritation. Consequently, most gels were excreted during the first urination, with few remained. In contrast, the 5% PFP hydrogel floated in the urine and showed control release of DOX. The concentration of DOX released from hydrogel reached 24.08% before the first urination. After urination, floating hydrogel remained releasing drug, and the concentrate of DOX was 29.86%, 19.33%, 11.08% and 11.41% during each urination in 330 min when the hydrogel dissolved completely in urine. Compared to the 0% PFP hydrogel, the 5% PFP hydrogel significantly prolonged the drug residence time in bladder vesicle and therefore enhances its bioavailability.
At last, the residual amount of DOX in the rabbit bladder of DOX-loaded non-floating hydrogel and DOX-loaded floating hydrogel was investigated (). We can see that the fluorescence of DOX was mainly distributed in the mucosa of bladder tissue, where the bladder cancer and interstitial cystitis invaded. It demonstrated that the floating hydrogel greatly reinforced the accumulation of drugs into diseased site. The fluorescence intensity of the floating hydrogel group was greater than the non-floating hydrogel group. The residual amount of DOX in the bladder tissue was proportional to its fluorescent intensity, so the residual amount of DOX in the rabbit bladder of floating hydrogel group was higher than the non-floating hydrogel group. Therefore, the floating hydrogel could significantly increase drug concentration that penetrated into bladder tissue, and hence improve the drug efficacy.
Figure 8. The frozen section was prepared immediately after isolating rabbit bladder tissues. The fluorescence of DOX was mainly distributed in the mucosa of bladder tissue, where the bladder cancer and interstitial cystitis invaded. The fluorescence intensity of the floating hydrogel group was greater than the non-floating hydrogel group.
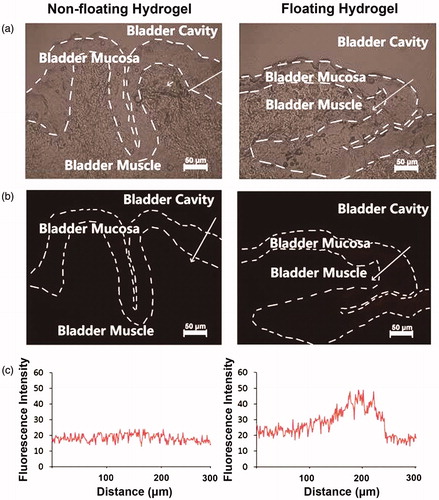
In contrast to our previous floating hydrogels, which float via microbubbles generation due to the chemical decomposition of inorganic compounds, this hydrogel encapsulating PFP is able to float since the density of gels decreases with the physical vaporization of PFP that swells the gel. The phase transition of PFP is milder and safer than chemical decomposition for clinic use. In addition, two perfluocarbon contrast agents have been approved by FDA for left ventricular opacification, Optison® and Definity®. Like these two products, PFP also acts as a safe and effective contrast agent and visualize the injection and floating process of hydrogel. This property makes our hydrogel the first intravesical delivery system that could be monitored using ultrasound.
Conclusion
This paper presents a new floating hydrogel drug delivery system for intravesical instillation with clinic prospects, by incorporation of contrast agent, PFP. The ingredients of floating hydrogel are P407 and PFP, both of which are biologically safe, addressing safety concerns for clinic use. The floating property of floating hydrogel solves the problem of urinary obstruction and bladder irritation that traditional mucoadhesive formulations might cause, and improves drug efficacy by prolonging the residence time of drug in the bladder. In addition, PFP as contrast agent makes the hydrogel clearly detectable via ultrasound, facilitating the clinic monitoring. Therefore, this new drug delivery system addresses the problems of conventional intravesical instillation and is promising for clinic use.
Declaration of interest
This study was supported by National Natural Science Foundation (No. 81202474, 81273464 and 81473146), Changzhou Special Project of Biotechnology and Biopharmacy (No. CE20105006). Science Bridges China-Changzhou Biotechnology and Pharmaceutical Technology Special Project (Grant Number: CE20105006). J. Wu is sponsored by Qing Lan Project.
References
- Chang LC, Wu SC, Tsai JW, et al. (2009). Optimization of epirubicin nanoparticles using experimental design for enhanced intravesical drug delivery. Int J Pharm 376:195–203
- Cima MJ, Lee H, Daniel K, et al. (2014). Single compartment drug delivery. J Control Release 190:157–71
- Collado A, Chechile GE, Salvador J, Vicente J. (2000). Early complications of endoscopic treatment for superficial bladder tumors. J Urol 164:1529–32
- Dumortier G, Grossiord JL, Agnely F, Chaumeil JC. (2006). A review of poloxamer 407 pharmaceutical and pharmacological characteristics. Pharm Res 23:2709–28
- Fraser MO, Chuang YC, Tyagi P, et al. (2003). Intravesical liposome administration – a novel treatment for hyperactive bladder in the rat. Urology 61:656–63
- Guhasarkar S, Banerjee R. (2010). Intravesical drug delivery: challenges, current status, opportunities and novel strategies. J Control Release 148:147–59
- Ilic-Stojanovic S, Nikolic L, Nikolic V, et al. (2012). Potential application of thermo-sensitive hydrogels for controlled release of phenacetin. Hem Ind 66:831–9
- Ilic-Stojanovic S, Nikolic L, Nikolic V, et al. (2013). Synthesis and characterization of thermosensitive hydrogels and the investigation of modified release of ibuprofen. Hem Ind 67: 901–12
- Krafft MP. (2001). Fluorocarbons and fluorinated amphiphiles in drug delivery and biomedical research. Adv Drug Deliv Rev 47:209–28
- Lin T, Zhang Y, Wu J, et al. (2014a). A floating hydrogel system capable of generating CO2 bubbles to diminish urinary obstruction after intravesical instillation. Pharm Res 31:2655–63
- Lin TS, Wu JH, Zhao XZ, et al. (2014b). In situ floating hydrogel for intravesical delivery of adriamycin without blocking urinary tract. J Pharm Sci 103:927–36
- Lu Z, Yeh TK, Tsai M, et al. (2004). Paclitaxel-loaded gelatin nanoparticles for intravesical bladder cancer therapy. Clin Cancer Res 10:7677–84
- Men K, Liu W, Li L, et al. (2012). Delivering instilled hydrophobic drug to the bladder by a cationic nanoparticle and thermo-sensitive hydrogel composite system. Nanoscale 4:6425–33
- Rapoport N, Gao Z, Kennedy A. (2007). Multifunctional nanoparticles for combining ultrasonic tumor imaging and targeted chemotherapy. J Natl Cancer Inst 99:1095–106
- Siegel R, Ma J, Zou Z, Jemal A. (2014). Cancer statistics, 2014. CA Cancer J Clin 64:9–29
- Tyagi P, Chancellor MB, Li Z, et al. (2004a). Urodynamic and immunohistochemical evaluation of intravesical capsaicin delivery using thermosensitive hydrogel and liposomes. J Urol 171:483–9
- Tyagi P, Li ZH, Chancellor M, et al. (2004b). Sustained intravesical drug delivery using thermosensitive hydrogel. Pharm Res 21:832–7
- Zhang D, Sun P, Li P, et al. (2013). A magnetic chitosan hydrogel for sustained and prolonged delivery of Bacillus Calmette-Guerin in the treatment of bladder cancer. Biomaterials 34:10258–66
- Zhang L, Parsons DL, Navarre C, Kompella UB. (2002). Development and in-vitro evaluation of sustained release Poloxamer 407 (P407) gel formulations of ceftiofur. J Control Release 85:73–81