Abstract
Topotecan (TPT) is indicated against a variety of solid tumors, but has restricted clinical use owing to associated pharmaceutical caveats. This study is focused at formulating a successful TPT PLGA nanosystem which ameliorates the rapid conversion of active lactone form of drug to its inactive carboxylate form and consequently improvises its efficacy. TPT PLGA nanoparticles were formulated by a double emulsion-solvent evaporation technique with sequential optimization to obtain desired particle size, PDI, zeta potential, and entrapment efficiency. Stability of TPT was ensured by maintaining an acidic pH in the drug-containing phase and the system was evaluated for in vitro–in vivo performance including cytotoxic potency. The optimized nanosystem had a particle size of 187.33 ± 7.50 nm, a PDI of 0.179 ± 0.05, and an entrapment efficiency of 56 ± 1.2%. Low pH in the interior of nanoparticles stabilized the drug to remain in its active lactone form and revealed a biphasic release pattern till 15 d. Additionally, an in vitro cytotoxicity testing as well as in vivo antitumor efficacy demonstrated a significant potential of higher proliferation inhibition as compared with neat drug (TPT). Thus, the investigation summarized an innovative simple tool for developing stable TPT NPs for effective delivery for treating solid tumors.
Introduction
The pathological complexity of cancer as a disease, its limited understanding, and varied mechanisms of treatment adds to the existing pharmacotherapeutic conundrum. Despite enormous on-going research in the area, a search for a successful delivery system is far from over. Additionally, off-target side effects leading to unacceptable cytotoxicity to normal healthy tissues and development of multi-drug resistance narrows down the application of conventional chemotherapeutic agents in combating cancer (Sahoo et al., Citation2007). The finest solution to overcome the said problem is through nanoparticle (NP)-based therapeutics. Polymeric NPs have been widely accepted as an efficient nanoscale drug delivery vehicle due to sustained release characteristic, excellent endocytotic uptake efficiency, passive tumor-targeting, high encapsulation efficiency, delivery of a wide range of cytotoxic agents, as well as favorable safety profile (Faraji & Wipf, Citation2009).
Nano-based therapeutics upon entering the systemic circulation accumulates in solid tumor. The phenomenon is referred to as enhanced permeability and retention (EPR) effect which is caused by leaky angiogenic vessels and poor lymphatic drainage and has been used to explain why macromolecules and nanoparticles are found at higher ratios in tumors compared to normal tissues. Small molecules (less than 40 kDa) are rapidly cleared by diffusion while large molecules and nanoparticles (up to 500 nm size) are retained in the interstitial space due to the absence of drainage (Dufort et al., Citation2012). The utilization of passive targeting in delivery of anticancer drugs has shown promising result in significantly increased mean survival time and even complete tumor eradication in tumor-bearing mice.
Controlling both particle size and encapsulation efficiency of drug-loaded nanoparticles stands as primary aspect to apply nanoparticles to drug delivery systems. Properly formulated polymeric nanoparticles with an acceptable size range remain in the blood circulation for a prolonged period of time resulting in an enhanced interaction with the tumor tissues.
One of the best known biodegradable carriers for controlled and sustained release is poly-lactide-co-glycolide (PLGA). PLGA NPs for delivery of therapeutics are of prime focus as it provides unique features such as biocompatibility, prediction of biodegradation kinetics, ease of fabrication, protection of entrapped therapeutics in biological environment rendering stability, and regulatory approval which has attracted its attention for a variety of biomedical applications. Moreover, both hydrophilic and lipophilic drugs can be successfully encapsulated in the PLGA matrix (Barichello et al., Citation1999). PLGA has been used for delivery of drugs for both oral (Jiao et al., Citation2002) and parenteral (Fonseca et al., Citation2002; Panagi et al., Citation2001) routes. FDA has already approved some of the formulations using PLGA which are currently being marketed and others are at various stages of clinical trials (Panagi et al., Citation2001).
TPT is a water-soluble semisynthetic derivative of camptothecin which is a potent inhibitor of DNA topoisomerase I and has shown good antitumor activity against a wide variety of solid tumors including breast cancer (Yu et al., Citation2012), ovarian cancer (Wethington et al., Citation2008), cervical cancer (Brave et al., Citation2006), as well as small cell lung cancer (Nicum & O’Brien, Citation2007). But, all the members in the camptothecin analogues are faced with a severe “stability” problem. The hydrolysis of the active lactone ring to the inactive carboxylate reduces the drug efficacy, and meanwhile the inactive form can lead to side effects (Beretta & Zunino, Citation2007). The transformation between the two forms is pH dependent. Unfortunately, the equilibrium favors the inactive form at physiological pH.
Thus, the project is aimed at improving the entrapment efficiency of a highly hydrophilic drug topotecan into PLGA nanoparticles and also keeping the equilibrium towards lactone fractions of TPT. Hence a double emulsion–evaporation technique was employed to entrap the chemotherapeutic after sequentially optimizing different parameters to achieve desired particle size, PDI, and entrapment efficiency. Circumvention of these pharmaceutical caveats would add on to the clinical dimension of TPT leading to an enriched cancer chemotherapeutic armamentarium.
Materials and method
TPT was purchased from 21 CEC PHARMA, London, UK, poly (d,l-lactide-co-glycolide) (PLGA, 50:50, 0.4 dL/g; MW 45 000) was purchased from Purac-Purosarb Biochem, Holland, THe Netherlands, PVA (polyvinyl alcohol, MW 25 000) purchased from Aldrich Chemical Inc. (Munich, Germany). All the reagents utilized were purchased from Merck, Mumbai, India. All the chemicals were used without further purification.
Preparation of TPT-loaded PLGA nanoparticles (TPT NPs)
TPT NPs were prepared by double emulsion solvent evaporation technique with slight modification (Bilati et al., Citation2005). The internal aqueous phase (IAP) containing TPT and minute quantities of 0.1 N HCl (to maintain pH 4) was dispersed into organic phase (OP) containing PLGA dissolved in dichloromethane (DCM) under sonication (Sonopuls, Bandelin, Germany) at (20–30 W, 35–40% duty cycle). This W1/O emulsion was then slowly dropped into the external aqueous phase (EAP) containing a suitable concentration of surfactant (PVA) under sonication and a secondary emulsion (W1/O/W2) was formed. The secondary emulsion was then kept for stirring (200 rpm) for 3 h to evaporate the organic solvent. The obtained nanoparticles were then centrifuged at 13 000 rpm for 20 min and washed three times with Milli-Q (Waters Technologies Corporation, Milford, MA). The obtained pellet was re-dispersed in 2–3 ml of Milli-Q water (Waters Technologies Corporation, Milford, MA). The dispersion was then kept for lyophilization (Lab Conco., LPYH, Lock 6, Kansas City, MO, freeze dryer) to get nanoparticles using an optimized freeze dried (Jain et al., Citation2010) process. Trehalose of 5% w/v was used as a cryoprotectant.
LC-MS/MS method for analysis of TPT
A newly developed HPLC-MS method was used for the analysis. The chromatographic system (Shimadzu HPLC System, Tokyo, Japan, SIL-HTC Auto sampler) included a mass detector with analytical column (C-18, Hypersil Gold) having dimensions of (150 × 2.1, 5 µm), i.e., column was 150 mm long, with an internal diameter of 2.1 mm and a pore size of 5 μm. Mobile phase used was 20:80:0.1 (% v/v/w) mixtures of 2 mM ammonium acetate, acetonitrile, and formic acid, respectively. The run time was 2.5 min and the column flow rate was set at 0.3 ml/min. The ions detected in case of TPT were 422.1 and 377.0 (molecular ion and daughter ion, respectively).
Preparation of TPT standard solution
Topotecan stock solution of 1 mg/ml of was prepared by dissolving it in dimethyl sulfoxide (DMSO). The stock solution was stored at −20 °C till further analysis. Required concentration of working solution was prepared by diluting the standard stock solution with DMSO. The final concentration of working solution was 5, 10, 25, 50, 75, and 100 µg/ml.
Optimization of process variables
Selection of surfactant concentration in EAP
Selection of suitable concentration of surfactant is necessary to obtain nanoparticles in the desired size range (<200 nm). For achieving this, nanoparticles were formulated by the above-described method, keeping other parameters constant (0% NaCl in EAP, 25 mg/ml polymer concentration in OP, sonication time: 30 s for primary emulsion and 1 min for secondary emulsion). The PVA concentration was varied in the range of 1–3% w/v in EAP. The optimized nanoparticles were then characterized in terms of particle size and PDI using zeta sizer (Malvern Instruments, Malvern, UK).
Effect of salt concentration (NaCl) in EAP
The suitable concentration of NaCl was selected based on nanoparticles with highest entrapment efficiency as well as optimum particle size, PDI, and zeta potential. TPT NPs were then prepared using different concentrations of NaCl ranging from 0 to 4% w/v in EAP.
Effect of polymer concentration in OP
Different concentrations of PLGA in OP (25–100 mg/ml) were employed to obtain nanoparticles. The optimum concentration of PLGA was selected in terms of particle size and entrapment efficiency.
Effect of sonication cycle
The primary and the secondary emulsion were subjected to different sonication times (30 s–2 min for primary emulsion and 1–3 min for secondary emulsion) and its effect on particle size and PDI was studied. Other experimental parameters optimized (as described above) were kept constant.
Detailed characterization of optimized TPT NPs
The following techniques were used to characterize the optimized nanoparticles.
Particles size and zeta potential measurement
Particle size was determined using dynamic light scattering (DLS17) technique with zeta sizer (Nano-ZS, Malvern Instruments, Malvern, UK) and analyzed by “DTS Nano” software (GraphPad Software Inc., San Diego, CA). Freeze-dried samples were dispersed in deionized water at 25 °C under gentle shaking, sonicated for 30 s in ice bath and filtered through 0.45 µm membrane filter in order to eliminate multi-scattering phenomenon and experimental errors. Average particle diameter (Δdm), polydispersity index (p¡), and zeta potentials (ζ) were recorded. All measurements were done in triplicate using disposable polystyrene cuvettes (Malvern Instruments, Malvern, UK).
Entrapment efficiency
One milligram of freeze-dried TPT NPs were dissolved in 1 ml of DMSO as it dissolves both TPT and PLGA. The resultant solution was used without further dilution and analyzed by the developed LC-MS method.
The entrapment efficiency was calculated by using the following formula:
Determination of lactone and carboxylate fraction of TPT
As TPT existed in two interconvertible forms, i.e., lactone and carboxylate, it was necessary to differentiate the two forms during the analysis. The chromatographic conditions followed for determination of the two forms were the same as mentioned in the section “Optimization of process variables”, while the reconstitution solution consisted of methanol: ammonium acetate (2 mM) at a ratio of 20:80. The pH of the reconstitution solution was maintained at three different pHs, i.e., 4, 6.5, and 8 while the chromatographic conditions remained the same for the analysis.
Determination of lactone and carboxylate fraction in TPT NPs.
To have a better estimation of the form available, the analysis of TPT NPs was done at neutral pH by dissolving nanoparticles in DMSO as all the components of nanoparticles including drug, PLGA, etc., were soluble in DMSO and the mobile phase was kept acidic (pH – 4.0), which could easily differentiate the amount of lactone and carboxylate form of TPT in the nanoparticles.
Morphology of nanoparticles
The surface morphology of nanoparticles was studied with the help of transmission electron microscope (TEM) (TOPCON 002B) operating at 200 kV, capable of point to point resolution. Properly diluted samples (1/100 in water) were used for TEM observations. The samples were treated on copper grids with a salt of heavy metal, i.e., 1% uranyl acetate for negative staining, followed by drying of the sample.
Differential scanning calorimetry (DSC)
In order to characterize the thermal behavior and physical state of drug loaded nanoparticle, excipients, and neat drug, DSC study was performed (Mirza et al., Citation2012). In brief, a heat influx instrument (DSC-7, Perkin Elmer Pyris 6 Instrument, PerkinElmer, Inc., Waltham, MA) equipped with a cooling system was used which was calibrated using indium as a standard. A nitrogen purge at a flow rate of 60 ml/min was used to provide an inert gas atmosphere in DSC cell. Five milligrams of samples were crimped in an aluminum pan and run against a hermetic empty reference pan. Heating program was run from 30 to 350 °C (highest melting points of organic substances) at a rate of 10 K min−1. Generally, scan rates are taken between 1 and 10 K min−1. Low scan rates are preferable in terms of peak resolution and investigation of the sample having close peaks while high scan rates increase the sensitivity of the measurement as they lead to the exchange of heat within a comparatively short time period. The instrument automatically calculates onsets and peak of melting point and enthalpy of fusion.
Fourier transform infrared spectroscopy (FTIR)
FTIR spectrometer as an analytical tool shows that the final formulation retains its chemical groups with nucleus indicates no fluctuation at drug stability. The FTIR of TPT NPs, excipients, and neat drug were recorded on the Perkin Elmer calorimeter (PerkinElmer, Inc., Waltham, MA) using the potassium bromide (KBr) disc technique as per the method described in (Mirza et al., Citation2011). Base line was corrected and scanning was done from 4500 to 400 cm−1.
In vitro release study
Briefly, 10 mg of nanoparticles was put in an eppendorf containing 1 ml of PBS (pH 7.4). The tubes were placed in a shaker at 37 °C at 200 rpm (Mishra et al., Citation2011). At specific intervals, the nanoparticles were separated by centrifugation, the release medium containing TPT was transferred to another tube and 1 ml of the medium was added. The supernatant containing drug was analyzed by HPLC-MS method (as discussed in the section Entrapment efficiency). Each measurement was done in triplicate.
The kinetics of TPT release from the nanoparticle suspension was determined using different kinetics models including: zero-order release kinetics (Varelas et al., Citation1995), first-order release kinetics (Gibaldi & Feldman, Citation1967), and Higuchi model (Higuchi, Citation1961). Release profiles were further submitted to kinetic analysis via the Korsmeyer–Peppas (Korsmeyer et al., Citation1983) equation to study the release mechanism.
Stability of TPT entrapped in nanoparticles in release medium and DMEM media
To have a proper insight about the stability of TPT either in solution form or encapsulated form in release media and physiological conditions, neat TPT (1 mg) and TPT NPs (10 mg) were incubated in PBS (pH 7.4) and complete DMEM media (pH 7.4, containing 10% FBS) at 37 °C. For neat TPT, at pre-determined time periods (1, 3, 6, 12, and 24 h), 20 µl of sample aliquots were collected, centrifuged at 10 000 rpm for 10 min and the supernatant was injected into LC-MS column for determination of lactone and carboxylate fraction of TPT, followed by the addition of fresh medium (PBS, pH 7.4) to make up the volume.
For TPT NPs, approximately 10 mg of nanoparticles were incubated in 1 ml of PBS and DMEM media (pH 7.4, 37 °C). At definite time points (1, 3, 6, 12, and 24 h), 100 µl of nanoparticles dispersion were collected and lyophilized immediately. The lyophilized sample was dissolved in DMSO followed by injection into the column for the simultaneous determination of lactone and carboxylate form of TPT. The analysis was carried out according to the method described in the section “Effect of salt concentration (NaCl) in EAP”.
Cell proliferation assay
Cells
Lewing lung cancer (LLC) cell line was obtained from National Centre for Cell Sciences, Pune, India. The cells were maintained in complete medium containing Dulbecco’s modified Eagle’s medium (DMEM; PAA, Pasching, Austria), 10% fetal bovine serum (FBS; PAA, Austria), and antibiotics (antibiotic–antimycotic solution; PAA, Pasching, Austria).
In vitro anticancer activity
LLC cells at a concentration of 5000 cells/100 µl of DMEM medium/well in a 96-well plate were seeded, incubated at 37 °C, 5% CO2 and allowed to grow for 24 h. After 24 h, the medium was removed from the wells and different concentrations of drug as well as formulation were added in triplicates to the cells. Untreated cells (containing only the media) acted as the control group. After definite period of incubation, the medium was removed from all the wells, 100 µl of 0.05 mg/ml MTT reagent (3 -(4,5-dimethylthiazol-2-yl)-2,5-diphenyltetrazolium bromide) was added to each well and the plates were kept in incubator for 4 h. Following this, the reagent was removed and the blue formazan crystals were solubilized in 100 µl of dimethyl sulfoxide (DMSO). The absorbance of the DMSO extract was measured at 570 nm using an ELISA plate reader. The background absorbance was taken at 630 nm. The value of absorbance is a measure of number of live cells. The corresponding values for OD for different control, free drug, and optimized formulation were recorded.
In vivo antitumor efficacy studies
Swiss albino mice of 5–7 weeks old, each weighing around 25–30 g were used for the study. The study was conducted in Ehrlich’s ascites tumor (EAT) bearing Swiss albino mice. EAT cells (1 × 106) were subcutaneously inoculated in the hind right hind flank of the mice to grow solid tumor. Mice were divided into three groups, containing five animals in each group. Once the tumor reached a desired range of 250 mm3, a dose of 20 mg/kg was administered to the groups of mice on days 0, 8, 15, and 22. The first group was injected with PBS intravenously (iv) which served as the control group. The second group received an i.v. injection of TPT in solution form while the third group received TPT NPs.
Tumor volume was measured at pre-determined time periods (1, 7, 14, 21, and 28 d) by digital verniercaliper and was calculated by using the following formula:
Results and discussion
TPT NPs were prepared using the modified double emulsion solvent evaporation method. Initial parameters included 1% (w/v) PVA and no NaCl was taken in EAP, 50 mg/ml PLGA in OP. The sonication time was fixed at 30 s to obtain primary emulsion and 1 min for secondary emulsion. The particle size of the formulated TPT NPs was found to be 299.66 ± 9.29 nm, with PDI of 0.312 ± 0.019 and zeta potential of −19.36 ± 0.49. The entrapment efficiency obtained was 1.99 ± 0.86%.
Detailed characterization of optimized TPT NPs
Sequential optimization, while taking only one parameter at a time, was performed. The variables evaluated included % w/v of PVA and % w/v NaCl used in EAP, PLGA concentration (mg/ml) in OP, and time of sonication. Using the above-mentioned technique, the key process variables were assessed in order to achieve optimal preparation conditions.
Selection of surfactant concentration in EAP
PVA has been reported to be a surfactant of choice for nanoparticles formulation. In our case, granulometric distribution appeared to become narrower as the amount of PVA was increased from 1% w/v to 2% w/v but widens as it is increased up to 3% w/v (). PVA of 2% w/v was sufficient to stabilize the emulsion droplets while a further increase of PVA concentration to 3% w/v resulted in increased viscosity of the aqueous phase resulting in the production of larger particles. Average particle sizes observed were between 254.96 and 299.66 nm. The zeta potential observed was negative and sufficient to provide stability. Hence, 2% w/v PVA was selected for the next sequential step. A minimum number of surfactant molecules are required to achieve small particle size and narrow size distribution for a given volume ratio of solvents. The surfactant added during the emulsification process effectively stabilizes the emulsion nanodroplets by sufficiently covering the organic/aqueous interfacial area of all the droplets leading to a decreased coalescence.
Table 1. Effect of different (1) PVA concentration and (2) sonication time on size, PDI, and zeta potential..
Effect of salt concentration (NaCl) in EAP
As per general observation, addition of salt or sugar into the continuous phase contributes to suppression of the initial burst (Jiang et al., Citation2002) which in turn also increases the entrapment efficiency. In our set of results, a fall in particle size was observed when the concentration of NaCl was increased up to 2% w/v, a staggered result from 2% w/v to 4% w/v was observed and then a sharp increase at 5% w/v which was attributed to the addition of ionic agent (NaCl) in EAP (). While a conspicuous enhancement in entrapment efficiency (nearly 25 times) was observed with the addition of 4% w/v of NaCl. However, no further enhancement in entrapment efficiency was observed when NaCl concentration was increased to 5% w/v. Hence, a salt concentration of 4%w/v was selected for further optimization. Salts affect drug loading by changing the aqueous solubility of both the drug and the organic solvent. In the emulsification solvent evaporation method for preparing nanoparticles, aqueous solubility of the organic solvent is generally low and the organic-to-aqueous phase volume ratio is small, thus organic solvent loss takes place by partitioning to the aqueous phase followed by evaporation from the aqueous phase at the water–air interface. Thus, the organic solvent has to dissolve in the aqueous phase before it evaporates. Anything added to the external phase during nanoparticle preparation that affects organic solvent solubility in the external phase can affect drug loading. This may also be explained by the ions binding water in their hydration shells, thus removing free water available for dissolving the organic solvent. This makes the aqueous environment enthalpically or entropically less favorable for insertion of a non-electrolyte and results in a decrease in its solubility (Grant & Higuchi, Citation1990). NaCl was found to increase DCM’s activity coefficient in water, thus leading to a salting-out effect of DCM (Brendel & Sandler, Citation1999).
Table 2. Effect of (1) polymer concentration and (2) salt (NaCl) concentration on size, PDI, zeta potential, and entrapment efficiency.
However, the addition of NaCl in the EAP resulted in a decrease in zeta potential values. The value decreased from −19.63 ± 0.465 mV to −2.4 ± 0.04 mV. The drop in the zeta potential values may be due to the adsorption of ions from the dispersion media onto the particle surface resulting in the decreasing thickness of electrical double layer (http://www.diss.fuberlin.de/diss/servlets/MCRFileNodeServlet/FUDISS_derivate_000000002344/06_7Chapter7.pdf?hosts=).
Effect of polymer concentration in OP
The PLGA concentration is a critical factor influencing the encapsulation efficiency. As shown in , the encapsulation efficiency was increased from 50.94% to 56% when the PLGA concentration in the organic phase was increased from 50 to 100 mg/ml. But due to the saturation of the organic phase, no further increase in the encapsulation efficiency was observed when the polymer concentration was further increased to 200 mg/ml. Taking both the size and the encapsulation efficiency of the particles into consideration, PLGA concentration of 100 mg/ml seemed optimal. It is generally observed that encapsulation efficiency increases with increasing polymer concentration (Li et al., Citation1999). The contribution of a high polymer concentration to the encapsulation efficiency can be interpreted in two ways. First, when highly concentrated, the polymer precipitates faster on the surface of the dispersed phase and prevents drug diffusion across the phase boundary (Rafati et al., Citation1997). Second, high concentration increases viscosity of the solution and delays the drug diffusion within the polymer droplets (Bodmeier & McGinity, Citation1988). The increase in particle size with an increasing polymer concentration was observed by other authors also for PLA and PLGA polymers (Chorny et al., Citation2002; Quintanar-Guerrero et al., Citation1996). This was probably caused by the increasing viscosity of dispersed phase (polymer solution), resulting in a poorer dispersibility of the PLGA solution into the aqueous phase. There is a high viscous resistance against the shear forces during the emulsification. Coarse emulsions are obtained at higher polymer concentrations, which lead to the build of bigger particles during the diffusion process.
Effect of sonication cycle
The addition of energy is a fundamental step in preparation of particulate nanosystems. The preparation procedure gave spherical particles in all cases (observation from TEM result). Another important factor observed was that an increase in sonication time leads to a narrower granulometric distribution-monomodal distribution profile. The final size of the nanoparticles depends on the globule size throughout the emulsification process. No differences in zeta potential were observed (−2.70 ± 0.22 to −2.50 ± 0.04 mV) (). The basic scientific principle governing the size of nanodroplets is that the external energy source (sonication) provides shear stresses to the organic phase, which results in the formation of nanodroplets. The size of the droplets is inversely correlated to the magnitude and time of shear stresses. Any change in processing or materials parameters that reduces the amount of shear stresses will increase the nanodroplet size. The most direct influence on the shear stresses in the organic phase is exercised by the energy density (external energy applied per unit total volume). Increasing the energy density directly increases the shear stresses and results in more efficient droplet breakdown and hence a reduction in nanodroplet size. The viscous forces in the organic and aqueous phase oppose the shear stresses in the organic phase. Reducing the organic phase viscosity results in a net increase in shear stress felt by the organic phase. This decreases the nanodroplet size. Small particles have important surface-to-volume ratios that bind the drug proportionally than larger particles (Aggarwal et al., Citation2009). Time of sonication also leaves a positive impact in particle size distribution, i.e., with sufficient energy, a monomodal distribution may be obtained.
Particles size and zeta potential measurement
The particle size and its distribution play an important role in determining the drug release kinetics, cellular uptake, and biodistribution of the NP, thus influencing the in vitro as well as in vivo therapeutic effects of the drug-loaded NP against malignancies (Misra & Sahoo, Citation2010). The size and the zeta potential of the optimized formulation were 187.33 ± 7.50 and −2.50 ± 0.04, respectively.
Entrapment efficiency
The entrapment efficiency of TPT from optimized TPT NPs was found to be 56 ± 1.2 % as calculated by following the mentioned formula.
Determination of lactone and carboxylate fraction of TPT
A prominent peak of lactone form of TPT was obtained when the pH of the reconstitution solution and the mobile phase both were acidic (pH 4). When the reconstitution solution was made neutral (pH 7–8) and the mobile phase was kept as acidic (pH 4), both the lactone and the carboxylate form co-existed. Whereas, when both the reconstitution solution and the mobile phase were basic (pH 10), TPT showed the prominent peak of carboxylate form.
The amphipathic character of TPT is strongly pH dependent. Among two reversible forms, an active lactone form exists at pH below 5 and an inactive carboxylate form exists at and above neutral pH. When the pH decreases below the pKa of these drugs, their amino group becomes protonated. This protonation increases the drug's aqueous-solubility and decreases its log D. When the pH decreases from 8 to 5, the drugs become more hydrophilic and more soluble by >100-fold. TPT has only one tertiary amine (one pKa).The hydrolytic property of TPT was best described by Ci et al. (Ci et al., Citation2012) which is a two-step process, the ring-opening of the lactone structure and ionization of the carboxylate
Kopen is relatively small with the value of about 0.002. The equilibrium constant could be written as K = [C−][H+]/[L], if the difference between activity and concentration is neglected. Since K = KopenKa, we have pK = pKopen + pKa. The form “C” could be regarded as an “acid”. It has been reported that pKa ( = −logKa) of an acid was increased in organic solvents or organic/water mixtures. It is thus not hard to understand that the pKa and thus pK of TPT would be increased in the presence of organic solvents. Therefore, the equilibrium moves to the left and leads to a higher lactone fraction.
Determination of lactone and carboxylate fraction in TPT NPs
The result of the study is given in , which indicates the presence of significantly higher fraction of lactone form of TPT in the nanoparticles.
In this study, polymeric nanoparticulate delivery systems were developed with poly (lactide-co-glycolide) in order to maintain the drug in its active lactone form. The degradation product of PLGA consists of two highly soluble acids, i.e., lactic acid and glycolic acids, hence an acidic environment is predicted as the polymer degrades. But to maintain the drug in its active lactone form, a low micro-environmental pH is also necessary. Maintenance of a low micro-environmental pH in the nanoparticle formulation depends on the formulation parameters employed and the specific excipients used during the formulation procedure to maintain the appropriate pH. Hence, in this study, we have maintained an acidic pH in the internal aqueous phase (IAP) containing the drug using 0.1 N HCl (pH 4). Low pH in the interior of nanoparticles stabilizes the drug to remain in its active lactone form. For the conversion of the lactone form of the drug to the carboxylate form, it must first be solubilized. Hence, low environmental pH may be a probable mechanism for the slow dissolution of the formed lactone crystals to the respective carboxylate form.
Morphology of nanoparticles
TEM studies were performed to further confirm the size and shape of the NPs. The images show that the average sizes of the particles were 100–200 nm (). The images further showed that the particles were discrete with a spherical outline. Notably, the shape of a particle seems to be of great importance.
Differential scanning calorimetry (DSC)
No characteristic TPT peak was observed when the drug was entrapped in NP (). This indicates that the drug was molecularly dispersed in an amorphous state, which favors easy diffusion of drug molecules through the polymeric matrix, resulting in sustained release of the drug from the NP (Sanders & Ashworth, Citation1961). Endothermic peak (232.549 °C) of TPT is dispersed, which vanishes completely when entrapped into NPs. It was evident from the thermograms that TPT was in amorphous state whether it was present in core or outer shells of the particle. The amorphous form of a drug has a higher thermodynamic and chemical potential while the crystalline state has the lowest total energy content.
Figure 3. (A) Differential scanning calorimetric (DSC) thermograms of dummy NPs, TPT-NPs, trehalose, pure TPT, and PLGA; (B) overlay of FTIR spectra of dummy NPs, pure TPT, and PLGA.
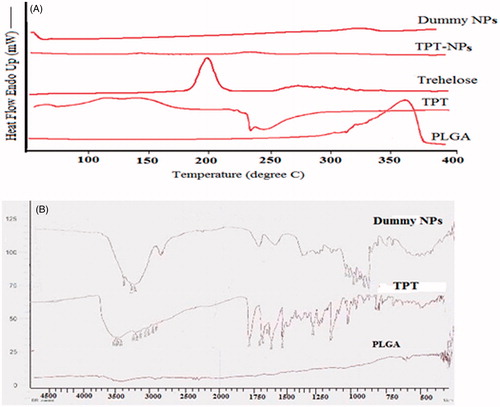
Organic substances usually show a melting range and an increased melting range could be correlated with impurities or less ordered crystals but the various instrumental analyses used in the current work have ensured lowest levels of impurities. A manual method of measuring melting range is peak width (i.e., difference between onset and maximum). Melting enthalpy (ΔH) is another characteristic of the crystal order if the influence of impurities is ignored. For the less ordered crystal or amorphous state, the melt of the substance does not require or just requires less energy than the perfect crystalline substance which is needed to overcome the lattice force. As a result, the higher melting enthalpy values should suggest higher ordered lattice arrangement and vice versa. In the developed NPs, there was not any ordered structure hence ΔH was negligible between any two points. Thermogram of dummy NP was almost similar to TPT NP`s thermogram in terms of melting peak and ΔH.
Fourier Transform Infrared Spectroscopy (FTIR)
FTIR studies were used to characterize any chemical interaction that occurred between drug and polymer during NP formulation. Due to the complex interaction of atoms within the molecule, IR absorption of the functional groups may vary over a wide range. However, it has been found that many functional groups give characteristic IR absorption at specific narrow frequency range. Multiple functional groups may absorb at one particular frequency range but a functional group often gives rise to several characteristic absorptions. The FTIR spectra of TPT, PLGA, and TPT NPs are shown in . Characteristic peaks due to different functional groups in native TPT appeared at 1754 cm−1, 1745 cm−1, 1740 cm−1, 1658 cm−1, 1649 cm−1, 1596 cm−1, 1584 cm−1, 1507 cm−1, and others in the fingerprint region. There were no characteristic peaks in the case of PLGA. Interaction between TPT and PLGA was evident in NPs. Characteristic peaks in the fingerprint region in the case of TPT NPs were either dispersed or diminished. A substantial interaction between both the moieties was evident from the study.
In vitro release study
Major advantage of any encapsulated system is to reach the target site and remain there for a prolonged period of time. Such a behavior is often predicted from the in vitro release. The release profile of the TPT-loaded PLGA NPs is shown in . Drug release studies of TPT NPs showed biphasic release pattern. It exhibited an initial release of approximately 78.29% in 7 d and then remaining fraction of drug was released (nearly 90.95%) in 15 d. As TPT is highly soluble in the continuous phase (EAP), the drug tends to migrate toward the exterior of the nanoparticles resulting in heterogeneous drug distribution ultimately leading to higher burst release. The initial release rate may be due to smaller particle size of nanoparticles which is associated with smaller diffusion path, hence the drug accessible to the solid/dissolution medium interface could lead to higher diffusion to the particle surface (Dunne et al., Citation2010). The release rate in the second phase was comparatively slow which indicates that the drug is entrapped in the polymer.
As a matter of fact, initial burst release is due to discharge of surface bound drug molecules present on the polymeric matrix, followed by sustained release due to subsequent diffusion/erosion of the polymeric matrix, enabling the drug to be released from NP into the aqueous medium (Dilnawaz et al., Citation2010)
To analyze the in vitro release data, various kinetic models were used to describe the release kinetics. The zero-order rates describe the systems where the drug release rate is independent of its concentration. The first-order describes the release from system where release rate is concentration dependent (order is one). Higuchi described the release of drugs from insoluble matrix as a square root of time-dependent process based on the Fickian diffusion, whereas Korsemeyer–Peppas describes the log fraction of drug release with respect to log time. The current system follows the Korsemeyer–Peppas model (Korsmeyer et al., Citation1983) and fitted into the equation:
where Mt is the amount of drug release at time t, Mf is the amount of drug release after infinite time; k is the release rate constant incorporating structural and geometric characteristics of the dosage form, n is the diffusional exponent indicative of the mechanism of drug release. The log value of percent drug dissolved is plotted against log time. For a cylinder shaped matrix, the value of n ≤ 0.45 indicates Fickian release; >0.45 but <0.89 for non-Fickian (anomalous) release; and >0.89 indicates super diffusion type of release. Hence, our release profile appears to be following non-Fickian release fashion.
Stability of TPT entrapped in nanoparticles in release medium and DMEM media
As restoration of bioactive fraction (lactone form) of TPT is essential for imparting good biological response, the fraction of lactone and carboxylate forms in Topotecan in physiological conditions was needed to be studied. The result of the experiment is demonstrated in . It was observed that neat TPT incubated in PBS and DMEM media underwent a rapid hydrolytical conversion of lactone ring to carboxylate one after 24 h resulting in only 28.98% and 12.26% of lactone fraction, respectively. However, it was seen that there was an increased rate of lactone hydrolysis when neat TPT was incubated in serum-containing DMEM medium. This may be due to the accelerated rate of hydrolytic conversion of the lactone ring of topotecan in the presence of albumin (Opanasopit et al., Citation2005). On the contrary, nearly 100% of the lactone ring was protected after 24 h incubation in PBS and DMEM when TPT is entrapped in PLGA nanomatrix. Hence, it can be inferred that the effective entrapment of TPT into PLGA nanoparticles successfully solved the stability issues related to the use of TPT under physiological conditions.
In vitro cytotoxicity studies
The ultimate test of an anticancer encapsulated system is its cytotoxic potency. The MTT assay demonstrated that the TPT NP formulation showed a comparatively lower IC50 value than the native drug IC50 value (at 48 h) obtained for TPT NP was 2.8 ± 0.88 µg/ml, whereas it was 4.2 ± 1.23 µg/ml for native drug. The graphical representation of the result is given in . It was observed that at a concentration of 10 and 20 µg/ml, the cell % viability values obtained for native TPT were found to be 36.18 ± 1.24% and 35.71 ± 1.86%, respectively. There was no much difference in the observed values which may be an indication of saturation of the native drug in the cellular compartment. In contrast to this, a marked difference in cell % viability was observed for TPT NP (32.84 ± 1.02 and 28.63 ± 1.56 for a concentration of 10 and 20 µg/ml, respectively). In general, cellular uptake of the native drug is by diffusion (Aggarwal et al., Citation2009). Studies have shown that after diffusion and intracellular saturation, further internalization of the native drug is prevented. Thus the small fraction of intracellular native drug available during the initial period of drug diffusion was responsible for eliciting the anti-proliferative effect of native TPT. Conversely, enough NP could be internalized into cancer cells by endocytosis to result in sustained drug release from NP on continuous exposure to cancer cells, resulting in significant cell toxicity (Sahoo et al., Citation2004).
Antitumor efficacy studies
Swiss albino mice were subcutaneously inoculated with EAT cells to form the solid tumor. When the tumor reached an approximate size of 250 mm3, all the groups of mice were dosed iv with either saline (control), TPT in solution form (20 mg/kg; days 0, 8, 15, and 22), and TPT NPs (20 mg TPT/kg; days 0, 8, 15, and 22).
At day 0, all the mice had a tumor size of 268.22 ± 12.62 mm3. The tumors in the saline group-treated mice grew rapidly to 527.66 ± 48.99 mm3 on 28-d post treatment, while the tumor volume of TPT and TPT NPs-treated group reached to 382.33 ± 45.43 mm3 156.55 ± 37.66 mm3, respectively ().
Figure 7. In vivo antitumor efficacy studies in Ehrlich’s ascites tumor (EAT) bearing Swiss albino mice. Data are represented as the mean ± standard deviation (n = 5).
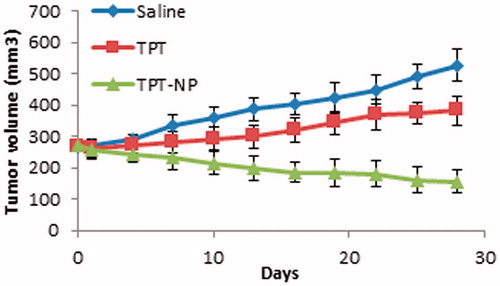
Restoration of active lactone fraction of TPT by encapsulating it in the polymeric nanomatrix and reduced conversion to its carboxylate form would have been a probable mechanism for the better antitumor efficacy of TPT-PLGA NPs. Additionally, the prevalence of acidic microenvironment in the solid tumors prevented the rapid conversion of the active form of the drug to its inactive ones which boosted up the biological activity. Lack of premature release in the biological fluid prior reaching the target cells resulted in sustained release of TPT from TPT NPs which could have resulted in better anti-tumor efficacy as compared with TPT in solution form.
Conclusion
The in vivo success of any formulation is linked to its in vitro behavior and is often affected by the rigors of formulation which it undergoes. Nanoparticulate system per se is known to additionally act as a cargo carrier for targeted delivery and has a responsibility of saving the drug or active pharmaceutical agent (API) from any instabilities both in vitro as well as at off target sites. The current work was aimed at revisiting the nanoformulation approach so as to design and develop a nanosystem for stable and effective delivery of an important water soluble anticancer drug, topotecan. Along with the common characterization like size, PDI, zeta potential, entrapment efficiency, morphology, etc., one of the other major objectives was to commission a system which protects the drug from being converted to its inactive carboxylate form. For this, maintenance of acidic microenvironment in the nanoparticles was established and maintained till the unloading of the drug cargo. The study elicited the production of suitably sized nanoparticles with acceptable PDI, zeta potential as well as increased entrapment efficiency which was obtained by elaborate sequential optimization of the parameters. The cytotoxic potential of the system was established by conducting the MTT assay on LLC cells which yielded appreciable positive outcomes. In addition to the observed effect, significant reduction of tumor volume in TPT NPs-treated group of mice confirmed the better in vivo anti-tumor effect of the nanoparticulate formulation as compared with TPT in solution form. In conclusion, the system can be considered as a potential for successful and effective delivery of the chosen anticancer drug for treatment of lung cancer, a challenging form of solid tumors.
Declaration of interest
The authors report that they have no conflicts of interest. The authors would like to thank Indian Council of Medical Research, Government of India, for providing Senior Research Fellowship.
References
- Aggarwal P, Hall JB, McLeland CB, et al. (2009). Nanoparticle interaction with plasma proteins as it relates to particle biodistribution, biocompatibility and therapeutic efficacy. Adv Drug Deliv Rev 61:428–37
- Barichello JM, Morishita M, Takayama K, Nagai T. (1999). Encapsulation of hydrophilic and lipophilic drugs in PLGA nanoparticles by the nanoprecipitation method. Drug Dev Ind Pharm 25:471–6
- Beretta GL, Zunino F. (2007). Relevance of extracellular and intracellular interactions of camptothecins as determinants of antitumor activity. Biochem Pharmacol 74:1437–44
- Bilati U, Allémann E, Doelker E. (2005). Poly(D,L-lactide-co-glycolide) protein-loaded nanoparticles prepared by the double emulsion method-processing and formulation issues for enhanced entrapment efficiency. J Microencapsul 22:205–14
- Bodmeier R, McGinity J. (1988). Solvent selection in the preparation of poly (DL-lactide) microspheres prepared by the solvent evaporation method. Int J Pharm 43:179–86
- Brave M, Dagher R, Farrell A, et al. (2006). Topotecan in combination with cisplatin for the treatment of stage IVB, recurrent, or persistent cervical cancer. Oncology 20:1401–15
- Brendel ML, Sandler SI. (1999). The effect of salt and temperature on the infinite dilution activity coefficients of volatile organic chemicals in water. Fluid Phase Equilibria 165:87–97
- Chorny M, Fishbein I, Danenberg HD, Golomb G. (2002). Lipophilic drug loaded nanospheres prepared by nanoprecipitation: effect of formulation variables on size, drug recovery and release kinetics. J Control Release 83:389–400
- Ci T, Li T, Chang G, et al. (2012). Simply mixing with poly (ethylene glycol) enhances the fraction of the active chemical form of antitumor drugs of camptothecin family. J Control Release 169:329–35
- Dilnawaz F, Singh A, Mohanty C, Sahoo SK. (2010). Dual drug loaded superparamagnetic iron oxide nanoparticles for targeted cancer therapy. Biomaterials 31:3694–706
- Dufort S, Sancey L, Coll JL. (2012). Physico-chemical parameters that govern nanoparticles fate also dictate rules for their molecular evolution. Adv Drug Deliv Rev 64:179–89
- Dunne M, Corrigan O, Ramtoola Z. (2010). Influence of particle size and dissolution conditions on the degradation properties of polylactide- co-glycolide particles. Biomaterials 21:1659–68
- Faraji AH, Wipf P. (2009). Nanoparticles in cellular drug delivery. Bio-org Med Chem 17:2950–62
- Fonseca C, Simoes S, Gaspar R. (2002). Paclitaxel-loaded PLGA nanoparticles: preparation, physicochemical characterization and in vitro anti-tumoral activity. J Control Release 83:273–86
- Gibaldi M, Feldman S. (1967). Establishment of sink conditions in dissolution rate determinations. Theoretical considerations and application to nondisintegrating dosage forms. J Pharm Sci 56:1238–42
- Grant DJ, Higuchi T. (1990). Solubility behavior of organic compounds. New York: John Wiley and Sons
- Higuchi T. (1961). Rate of release of medicaments from ointment bases containing drugs in suspension. J Pharm Sci 50:874–5
- Jain S, Mittal AK, Jain A, et al. (2010). Cyclosporin A loaded PLGA nanoparticle: preparation, optimization, in-vitro characterization and stability studies. Curr Nanosci 6:422–31
- Jiang G, Thanoo B, DeLuca PP. (2002). Effect of osmotic pressure in the solvent extraction phase on BSA release profile from PLGA microspheres. Pharm Dev Technol 7:391–9
- Jiao Y, Ubrich N, Marchand-Arvier M, et al. (2002). In vitro and in vivo evaluation of oral heparin–loaded polymeric nanoparticles in rabbits. Circulation 105:230–5
- Korsmeyer RW, Gurny R, Doelker E. (1983). Mechanisms of solute release from porous hydrophilic polymers. Int J Pharm 15:25–35
- Li X, Deng X, Yuan M, et al. (1999). Investigation on process parameters involved in preparation of poly-dl-lactide-poly (ethylene glycol) microspheres containing Leptospira Interrogans antigens. Int J Pharm 178:245–55
- Mirza MA, Ahmad N, Agarwal SP, et al. (2011). Comparative evaluation of humic substances in oral drug delivery. Results Pharma Sci 1:16–26
- Mirza M, Talegaonkar S, Iqbal Z. (2012). Particle engineering in the perspective of thermodynamics: a case study with itraconazole. Adv Sci Lett 16:148–58
- Mishra N, Tiwari S, Vaidya B, et al. (2011). Lectin anchored PLGA nanoparticles for oral mucosal immunization against hepatitis B. J Drug Target 19:67–78
- Misra R, Sahoo SK. (2010). Intracellular trafficking of nuclear localization signal conjugated nanoparticles for cancer therapy. Eur J Pharm Sci 39:152–63
- Nicum SJ, O’Brien ME. (2007). Topotecan for the treatment of small-cell lung cancer. Expert Rev Anticancer Ther 7:795–801
- Opanasopit P, Yokoyama M, Watanabe W, et al. (2005). Influence of serum and albumins from different species on stability of camptothecin-loaded micelles. J Control Release 104:313–21
- Panagi Z, Beletsi A, Evangelatos G, et al. (2001). Effect of dose on the biodistribution and pharmacokinetics of PLGA and PLGA–mPEG nanoparticles. Int J Pharm 221:143–52
- Quintanar-Guerrero D, Fessi H, Allemann E, Doelker E. (1996). Influence of stabilizing agents and preparative variables on the formation of poly (D,L-lactic acid) nanoparticles by an emulsification-diffusion technique. Int J Pharm 143:133–41
- Rafati H, Coombes A, Adler J, et al. (1997). Protein-loaded poly (DL-lactide-co-glycolide) microparticles for oral administration: formulation, structural and release characteristics. J Control Release 43:89–102
- Sahoo SK, Ma W, Labhasetwar V. (2004). Efficacy of transferring-conjugated paclitaxel-loaded nanoparticles in a murine model of prostate cancer. Int J Cancer 112:335–40
- Sahoo SK, Parveen S, Panda JJ. (2007). The present and future of nanotechnology in human health care. Nanomedicine 3:20–31
- Sanders E, Ashworth CT. (1961). A study of particulate intestinal absorption and hepatocellular uptake. Exp Cell Res 22:137–45
- Varelas CG, Dixon DG, Steiner CA. (1995). Zero-order release from biphasic polymer hydrogels. J Control Release 34:185–92
- Wethington SL, Wright JD, Herzog TJ. (2008). Key role of topoisomerase I inhibitors in the treatment of recurrent and refractory epithelial ovarian carcinoma. Expert Rev Anticancer Ther 8:819–31
- FreieUniversität Berlin. (2006). Cyclosporine nanosuspensions. Available at: http://www.diss.fuberlin.de/diss/servlets/MCRFileNodeServlet/FUDISS_derivate_000000002344/06_7Chapter7.pdf?hosts=[last accessed 27 Oct 2015]
- Yu Y, Wang Z-H, Zhang L, et al. (2012). Mitochondrial targeting topotecan-loaded liposomes for treating drug-resistant breast cancer and inhibiting invasive metastases of melanoma. Biomaterials 33:1808–20