Abstract
Purpose: Nasal galantamine hydrobromide (GH)/chitosan complex nanoparticles (CX-NP2) could have an improved therapeutic potential for managing Alzheimer’s disease (AD). The current study aimed to investigate if the complexation reaction between GH and chitosan altered the pharmacological and toxicological profiles of the parent drug; GH.
Methods: The nasal administration of CX-NP2 to male Wistar rats for 12 consecutive days was compared to negative control group, and oral and nasal GH solutions treated groups in 3 mg/kg daily GH dose. Brain acetylcholinesterase (AChE) protein level and activity were assessed. The in vivo toxicity of CX-NP2 was evaluated via monitoring the clinical signs throughout the study. Histopathological examination of brain sections was performed. The intracellular localization of CX-NP2 within brain neurons was investigated using transmission electron microscopy.
Results: GH/chitosan complexation did not negatively alter the pharmacological efficiency of GH. Intriguingly, nasal CX-NP2 exhibited a significant decrease of AChE protein level and activity in rat brains compared to the oral and nasal GH solutions. No toxicity signs or histopathological manifestations were noticed. The nanoparticles were found intracellularly in the brain neurons.
Conclusion: The pharmacological efficacy and in vivo safety of nasal CX-NP2 confirm their promising potential to contribute to the management of AD intranasally.
Introduction
Alzheimer’s disease (AD) is the most widespread dementia type (Carmo Carreiras et al., Citation2013). Symptomatic treatment strategies for AD are used to improve cognition and memory, hence recover a better quality of life. As stated by the cholinergic hypothesis of AD pathogenesis (Contestabile, Citation2011), cholinergic neurons and acetylcholine (ACh) synthesis are highly affected leading to memory impairment and cognition defectiveness. The classic strategy to enhance the cholinergic neurotransmission is to delay ACh degradation in the synaptic cleft by inhibiting acetylcholinesterase using cholinesterase inhibitors (ChEIs). The currently FDA-approved ChEIs are donepezil, rivastigmine, and galantamine (Contestabile, Citation2011).
The blood-brain barrier (BBB) is the gate that drugs and delivery systems circulating in blood have to cross to reach the central nervous system (CNS) (Chen & Liu, Citation2012). Apart from the numerous strategies investigated to overcome the BBB selective permeability (Chen & Liu, Citation2012), direct drug delivery to the brain via intranasal administration has gained an utmost attention (Galgatte et al., Citation2014; Bhatt et al., Citation2015; Ravi et al., Citation2015). The nasal route represents a quite simple and non-invasive way to entirely bypass the BBB (Lochhead & Thorne, Citation2012). The major advantages of the intranasal route include ease of self-administration, non-invasiveness, fast onset of action, avoidance of first pass metabolism, and minimizing the systemic side effects (Lochhead & Thorne, Citation2012).
Among several nanocarriers for brain drug delivery, biodegradable polymeric nanoparticles have gained an utmost attention due to their advantages. They exhibit an improved stability both in vitro and in vivo, and offer a better control over the drug release than liposomes (Bamrungsap et al., Citation2012). From an industrial perspective, the production cost, ease of manufacture, and sterilization feasibility of polymeric nanoparticles should not be overlooked. Chitosan nanoparticles (CS-NPs) gained the attention as biodegradable nanoparticles with good stability (Ong et al., Citation2014). They offer several advantages over other polymeric nanoparticles when used as nasal delivery system targeting the brain (Elgadir et al., Citation2014). Local and systemic toxicity characteristics of chitosan have been addressed previously in the literature (Baldrick, Citation2010). However, the literature lacks studies that investigate the in vivo toxicity of CS-NPs upon intranasal administration specifically for brain drug delivery.
Galantamine hydrobromide (GH) is a multi-mode of action neurotherapeutic drug for managing AD. It is a reversible, competitive inhibitor of AChE. It also binds allosterically to nicotinic acetylcholine receptors (nAChRs); increasing the receptors’ sensitivity to ACh (Dineley et al., Citation2015). Furthermore, it has been found that GH slows down plaque formation and behavioral decline in the 5XFAD mouse model of AD (Bhattacharya et al., Citation2014).
It has been reported that GH oral administration is accompanied by gastrointestinal adverse events that severely affect patient compliance and could even lead to treatment discontinuation (Lilienfeld, Citation2002). Therefore, developing alternative GH formulations designed for intranasal administration is a must. Leonard et al. (Leonard et al., Citation2007) formulated an intranasal dosage form of galantamine lactate with randomly methylated β-cyclodextrin (Me-β-CD) as a permeation enhancer (Leonard et al., Citation2007). They incubated a tissue model consisting of primary human upper airway epithelia with the formulation for 2 h, and then assessed the cytotoxicity (Leonard et al., Citation2007). They concluded that the prepared formulation was non-toxic. However, the safety of cyclodextrins for nasal drug delivery has been questioned over years as most of cyclodextrins may compromise the integrity of nasal mucosa upon chronic use (Irie & Uekama, Citation1997). Even though Leonard et al. proved that Me-β-CD was non-toxic, they conducted the study for only 2 h which is not considered quite enough duration to judge an enhancer’s possible cytotoxicity (Irie & Uekama, Citation1997).
Li et al. (Citation2012) prepared GH-loaded flexible liposomes for intranasal brain delivery of GH. They deliver GH to the brain rapidly upon nasal administration and they were non-toxic to PC-12 cells (Li et al., Citation2012). While the prepared liposomes appeared to have a high drug-carrying capacity, it has been known that regulating the drug-release profile from liposomes is difficult (Akbarzadeh et al., Citation2013). Furthermore, the used preparation method of flexible liposomes was a multistep procedure in addition to involving a solvent evaporation step (Li et al., Citation2012). Liposomes are also known to have shelf-stability issues, relatively-high production cost, and probable drug leakage (Akbarzadeh et al., Citation2013).
Other than the previously mentioned studies, no further attempts have been made to develop an intranasal nanoparticulate formulation of GH. In a previous study conducted by our group (Hanafy et al., Citation2015), complexation as an approach to enhance the entrapment of GH into CS-NPs for AD management intranasally was investigated. The complex formed between GH and chitosan was prepared prior to preparing the GH/chitosan complex nanoparticles (CX-NP2) (Hanafy et al., Citation2015). The complexation prolonged GH release and improved formulation stability at 4 °C. The fluorescent-labeled CX-NP2 were detected in the rat olfactory bulb, hippocampus, orbitofrontal and parietal cortices 1 h after intranasal administration (Hanafy et al., Citation2015).
Since the vast spread of the applications of nanoparticles in drug delivery, the research interest in their toxicity has been increased. Extending the use of such nanoformulations to human applications requires not only appraising their pharmacological efficacy but also their substantial safety. Despite the fact that biodegradable polymers are usually used to prepare the aforementioned nanoparticles, their nanotoxicity should not be overlooked.
The aim of this study was to investigate if the complexation reaction between GH and chitosan altered the pharmacological and toxicological profiles of the parent drug; GH. The pharmacological efficacy of the prepared CX-NP2 was assessed via determining their effect on AChE level and activity in rat brain. The safety and in vivo toxicity of nasal CX-NP2 compared to the same GH dose as an oral or a nasal solution was evaluated. Furthermore, transmission electron microscopy (TEM) was used to investigate the intracellular localization of CX-NP2 within the brain neurons.
Materials and methods
Materials
Galantamine hydrobromide (GH) was purchased from Shaanxi Jintai Biological Engineering Co., Ltd (Xi'an City, China). Medium Mwt chitosan (Mw= 200 kDa, degree of acetylation >90%) was purchased from Carl Roth GmbH Co. (Karlsruhe, Germany). Sodium tripolyphosphate (TPP) was purchased from Loba Chemie Pvt. Ltd. (Mumbai, India). Rat Acetylcholinesterase (AChE) ELISA Kit was purchased from WKEA Med Supplies Corp. (Changchun, China). AChE assay kit was purchased from Abcam Co. (Cambridge, MA). Protein Biuret Method Colorimetric Assay kit was purchased from Biodiagnostic Co. (Giza, Egypt). Concentrated hydrochloric acid, glacial acetic acid, and diethyl ether were purchased from S.D. Fine Chemicals, Ltd. (Mumbai, India). Glutaraldehyde 50% solution (biological grade) was purchased from Fisher Scientific UK Ltd. (Loughborough, UK). Sodium hydroxide was purchased from Chemajet Chemical Co. (Egypt). Polysorbate 80 (Tween 80), potassium dihydrogen phosphate, disodium hydrogen phosphate, sodium chloride, potassium chloride, formalin solution (35%), ethanol 99%, and ethanol 95% were purchased from El-Nasr Pharmaceutical Chemicals Co. (Qaliubiya, Egypt). All chemicals were of analytical grade.
Methods
Preparation of galantamine hydrobromide/chitosan complex nanoparticles (CX-NP2)
GH/chitosan complex nanoparticles (CX-NP2) were prepared according to our previous study (Hanafy et al., Citation2015). Briefly, GH (7 mg) was added to 10 ml chitosan solution in 1% v/v acetic acid (2 mg/ml) and the pH was adjusted to 4.5. The solution was stirred over a magnetic stirrer (Daihan Scientific Co., Korea) for 24 h at room temperature producing GH/chitosan complex. After 24 h, Tween 80 (0.5% w/v) and 4 ml TPP solution (1.5 mg/ml) were added. The dispersion of CX-NP2 was stirred for 30 min at 1200 rpm. To concentrate the nanoparticles dispersion, it was centrifuged at 15 000 rpm for 45 min (4 °C) using K241R benchtop centrifuge (Core Life Sciences, Inc., CA). The nanoparticles were resuspended in 50 μl 0.9% w/v sterile saline solution to yield a final GH concentration of 30 μg/μl (pH 6.5-7).
Animal study and protocol
Adult healthy male Wistar rats (4–5 months old, 180–220 g) were used in this study. They were housed in standard metal cages (3 to 4 subjects per cage) at 23 ± 2 °C. The animals were acclimatized for two weeks before starting the experiment. Water was allowed ad libitum, while standard diet pellets were supplied as 20 g/100 g body weight/day. All animal procedures were conducted according to the published guidelines by the Ethical Committee for Care and Use of Laboratory Animals (Faculty of Pharmacy & Drug Manufacturing, Pharos University in Alexandria) and approved by the Research Ethics Committee of Faculty of Pharmacy & Drug Manufacturing, Pharos University in Alexandria. Appropriate measures were taken to minimize discomfort in adherence to the “Principles of Laboratory Animal Care” (NIH publication #8523, revised in 1985) (Bethesda, Citation1985).
Dose administration
For comparison with the nasal CX-NP2, GH oral solution in distilled water (4 μg/μl) and GH nasal solution in 0.9% w/v sterile saline (30 μg/μl) were also prepared. The animals were divided into four groups. Treatments were administered once daily at 9:00 AM for 12 consecutive days (Wu et al., Citation2012). summarizes the number of animals in each group, and drug dosing protocol. Geerts et al. (Geerts et al., Citation2005) have reported that GH doses between 1.5 and 5 mg/kg/day are appropriate to reach brain levels leading to AChE inhibition accompanied by allosteric potentiating effects at the nicotinic ACh receptors. Therefore, a middle dose (3 mg/kg/day) was selected in this study.
Table 1. Dosing of animal groups encompassed in the study.
Just before intranasal administration, rats were sedated by ether inhalation. GH dose was given by inserting a micropipette tip attached to a 5–50 μl micropipette (Accumax Co., Gujarat, India) in one nostril (∼5 mm inside nostril) (Botner et al., Citation2012). The rat was held upright and the administered solution was allowed to flow with normal inhalation (Priprem et al., Citation2011). The rat was held in the same position for 20–30 s to maximize the delivery of the dose to the deeper nasal mucosal surfaces and to prevent flooding (Elnaggar et al., Citation2015). The upright position was preferred over the supine position to create challenging and simulating conditions to the administration in humans. Administration was performed in a single nostril, and alternated between the two nostrils every day (Migliore et al., Citation2010).
Determination of AChE protein level and activity in rat brains
Sample preparation
On the 13th day, rats were ether anesthetized, euthanized by cervical dislocation, and decapitated. The whole brains along with the olfactory bulbs were removed, rinsed with ice-cold 0.9% saline solution, weighed, and stored at –80° C. The brains were homogenized five times with w/v ice-cold PBS (pH 7.2 – 7.4) on ice. Brain homogenates were centrifuged (15 000 rpm, 4 °C for 10 min). Supernatants were aliquoted into eppendorf tubes and stored at –80 °C till used in ELISA measurement, protein analysis and AChE activity assessment.
Determination of AChE protein level using ELISA
For quantitative analysis of the protein level of AChE in the brain lysates, rat AChE colorimetric ELISA kit was used. The protocol supplied with the kit was followed throughout the assay. The absorbance of the yellow color produced at the end of the procedure was measured at λmax 450 nm using a Benchmark microplate reader (Bio-Rad Laboratories, CA). Standard curve was constructed to calculate AChE concentration; which was expressed as pg/g total protein.
Determination of AChE activity
Quantitative estimation of AChE activity was performed according to the protocol supplied with a commercial AChE assay kit; which relies on the colorimetric assay described by Ellman et al. (Citation1961). The produced absorbance of the yellow color developed at the end of the assay was determined spectrophotometrically at 412 nm using a UV-Visible spectrophotometer (Shimadzu Corporation, Japan). The color intensity is directly proportional to the AChE activity in the brain sample (Ellman et al., Citation1961). The activity was expressed as nanomoles of acetyl thiocholine iodide hydrolyzed/minute (U)/g total protein.
Estimation of total protein
Aliquots of brain homogenate supernatants were assayed for total protein using Biuret method, which was selected as it is sensitive yet involves a single incubation period (Sapan & Lundblad, Citation2015). Brain supernatants (25 μl) or standard serum albumin (25 μl) were mixed with 1 ml of Biuret reagent and incubated for 10 min at 37 °C. The absorbance (A) was determined at 550 nm using a UV-Visible spectrophotometer. The protein concentration was calculated using EquationEquation (1)(1) .
(1)
Toxicity assessment of intranasal CX-NP2
Clinical signs, body weight, and food consumption observations
Throughout the study, the health status of the rats was monitored. Mortality, morbidity, and behavioral changes were noted. In addition, the animals were weighed daily at a fixed time (Xu et al., Citation2013). The %change in body weight from the baseline (determined on day 1) was calculated using EquationEquation (2)(2) :
(2)
During the acclimatization period, the optimum food intake for rats was determined by supplying a pre-weighed amount of standard food pellets. On the next day, the unconsumed pellets were weighed and the final weight was supplied thereafter (Yun et al., Citation2015). The feeding time was fixed and the changes in the food consumption were reported throughout the study.
Serum biomarkers and differential white blood cells count
Serum urea and creatinine levels are indicative of kidney functions, while aspartate transaminase (AST) and alanine transaminase (ALT) serum levels mirror the liver functions. They were determined using Cobas C311 analyzer (Roche Diagnostics, GmbH, Germany). The percentage change in serum biomarkers from the baseline (determined on day 1) was calculated using EquationEquation (3)(3) :
(3)
To evaluate the immunocompatibility of CX-NP2, blood samples were collected from the control group and the nasal CX-NP2 treated group and analyzed for the total leukocytes, neutrophils, lymphocytes, monocytes, eosinophils, and basophils using an Automated Hematology Analyzer (Advia 120, Bayer, Germany).
Brain histological examination using light microscopy
On the 13th day, three rats from each group were ether anesthetized and sacrificed by cervical dislocation. The whole brains were excised and sliced into 3 mm coronal sections. To prepare the brain sections for hematoxylin and eosin (H&E) staining, the procedure reported by Zhang & Xiong (Citation2014) was adopted. Brain sections were fixed in 10% formalin solution for two days and then dehydrated in graded concentrations of alcohol (50, 70, 95, and 99%), each for 24 h. Then, a clearing process was conducted by embedding the brain tissues into xylene for 24 h. Brain sections (5 μm) were obtained using a rotary microtome (RM2135, Leica Biosystems Co., Wetzlar, Germany) and stained using a standard H&E staining protocol (Feldman & Wolfe, Citation2014).
Neuronal localization and toxicity assessment of CX-NP2 using electron microscopy
On the 13th day of the study, three rats from each of the negative control and the nasal CX-NP2 treated groups were randomly selected. A perfusion surgery was performed following the protocol described by Gage et al. (Citation2012). The animals were ether anesthetized throughout the surgery. The chest was operated and the diaphragm was cut off to expose the heart. A cannula was passed into the root of the ascending aorta. An incision was made in right atrium and ∼50 ml of 0.9% sterile saline solution (prewarmed to 37 °C) was injected slowly through the cannula until the outflow from the incised right atrium was running clear. Then, 50 ml of the fixative solution (5 ml 50% glutaraldehyde mixed with 37 ml 0.2 M phosphate buffer and the volume was brought to 100 ml with distilled water) was injected. Fixation tremors, observed within seconds, and gradual stiffness of the whole body were indicative of a proper perfusion procedure. The entire procedure took about 2 min. After the perfusion was completed, the brain was dissected out and transferred to a Petri dish containing the fixative solution. The orbitofrontal cortex was dissected and prepared for electron microscopy. Ultrathin sections (70 nm) were cut using an ultratome (Leica Ultracut R, Leica Biosystems Co., Wetzlar, Germany) and examined using TEM (JEM-1010, Joel Ltd., Tokyo, Japan).
Statistical analysis
The reported data were analyzed using GraphPad Prism 5.0 software (GraphPad Software Inc., CA). Data were represented as the mean ± standard deviation (SD). The mean values of the study groups were compared using one-way analysis of variance (ANOVA) followed by Tukey’s post-test for pairwise comparison. Statistical significance was judged at the 0.05 probability level unless otherwise specified.
Results and discussion
The present study was designed to investigate if the complexation of GH with chitosan (nasal CX-NP2) alters the brain AChE protein level and activity, in comparison with the same GH dose (3 mg/kg) either orally or as a nasal solution. The administered GH solution or dispersion amount was varied according to the animal’s body weight to eliminate any interfering factors. In addition, the toxicity, side effects, and brain neuronal localization of CX-NP2 were examined.
Determination of AChE protein level and activity in rat brains
It was important to check if the complexation reaction had altered the pharmacological efficacy of the parent GH. It was thought that relying solely on the quantitative estimation of GH brain uptake could be misleading. Measuring GH brain levels would not guarantee its pharmacological efficiency; as GH-chitosan interaction might alter GH binding to the brain AChE enzyme via steric hindrance or any other mechanism; thus affecting the entire pharmacological profile of GH (Tumiatti et al., Citation2008). The protein level and activity of brain AChE were determined and considered potential markers of CX-NP2 efficacy (Nordberg et al., Citation2009). Sacrificing and collecting the brains was performed on the 13th day, 24 h after the last administered dose, to investigate if the nanoparticles might have a prolonged effect and long brain residence time.
At the end of the treatment period, all treated groups showed a statistically significant decrease in AChE protein level compared to the negative control group (p < 0.05). However, nasal CX-NP2 appeared to result in a markedly decreased AChE protein level in comparison with both oral GH solution (p < 0.05) and nasal GH solution (p < 0.01) ().
Figure 1. (A) AChE protein levels determined in rat brains after administering oral GH solution, nasal GH solution, or nasal CX-NP2, compared to negative control rats, using ELISA technique (data are expressed as mean ± SD, n = 6). (B) Brain AChE activity measured colorimetrically at λmax 412 nm for the same study groups (data are expressed as mean ± SD, n = 6). Significant differences are indicated (*, p < 0.05; **, p < 0.01; ***, p < 0.001) (AChE, acetylcholinesterase; GH, galantamine hydrobromide).
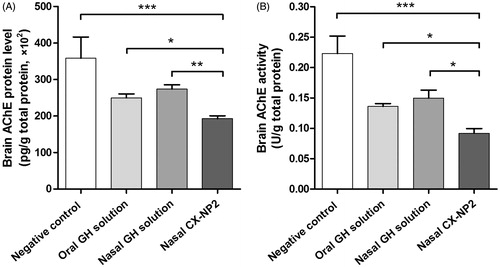
A comparable order of magnitude was observed when the brain AChE activity was assessed. Nasal CX-NP2 significantly decreased the brain AChE activity compared to the negative control group (p < 0.001). Moreover, nasal CX-NP2 significantly inhibited the brain AChE activity in comparison with the same dose (3 mg/kg/day) administered either orally or as a nasal solution (p < 0.05) ().
Based on the targeting potential of nasal CX-NP2 and the distribution to different brain regions; which was evidenced in the fluorescence visualization experiment in our previous study (Hanafy et al., Citation2015), we could expect their long-term clinical superiority over the oral and nasal GH solutions; that could be seen if the treatment period was longer. In particular, the effect of nasal CX-NP2 on AChE protein level might be clinically desirable for long-term treatment efficiency. In addition, obtaining such promising results despite sacrificing the animals 24 h after the last administered dose suggests the nanoparticles’ prolonged residence time. This is expected to be advantageous if a less frequent GH dosing regimen is aimed at.
The results of some studies (Davidsson et al., Citation2001; Darreh-Shori et al., Citation2008; Nordberg et al., Citation2009) indicate that GH leads to a significant upregulation of AChE protein level (increased number of AChE enzyme); which might be clinically undesirable. These studies referred particularly to the initiation of a feedback upregulation mechanism as a result of AChE inhibition by GH. The underlying mechanisms that have led to the differences between the obtained results and the previously published ones are currently unknown. However, there are two possibilities that are presently favored. Firstly, 12 days’ treatment in the current study could be too short to elicit the feedback upregulation. For instance, Nordberg et al. (Citation2009) conducted their study to determine the effect of GH on AChE protein levels and activity in cerebrospinal fluid (CSF) for 13 weeks. Secondly, most of these studies reported on the increased AChE protein level in CSF (Darreh-Shori et al., Citation2008; Nordberg et al., Citation2009), while the present study reports on AChE protein level in the brain tissue. Therefore, further experimental and clinical long-term researches are required to elucidate this dilemma.
It is thought that the significantly improved efficacy of the nasal CX-NP2 could be due to several factors, most importantly the prolonged GH release from the nanoparticles (only 58.07% ± 6.67 after 72 h) (Hanafy et al., Citation2015).
As reported by Goh et al. (Citation2011), administering GH orally in rats exhibited a high bioavailability (83 ± 13%) and 1.7:1 ± 0.2 brain/blood ratio 1 h after administration. However, GH is metabolized by hepatic cytochrome P450 enzymes, > 60% of its metabolites are excreted via urine, and it has a short half-life (t1/2) in rats (∼1.9 h) (Mannens et al., Citation2002). Consequently, the capability of CX-NP2 of prolonging GH release and decreasing its metabolism is thought to contribute to not only the improved AChE inhibition, but also the significantly decreased AChE protein level in the brain. Decreasing GH metabolism is a long-term pharmacological response that requires a prolonged residence in the brain tissues. The altered AChE protein level could be used as a marker reflecting the changes in the expression of AChE in the brain’s cholinergic neurons (Darreh-Shori et al., Citation2004).
The prepared CX-NP2 could have been transported across the olfactory and respiratory epithelia reaching the olfactory bulb and trigeminal nerve fibers, respectively (Lochhead & Thorne, Citation2012). Subsequently, the nanoparticles might have spread to different brain regions. It can be inferred that applying CX-NP2 intranasally helped completely bypassing the BBB; which was reflected on their significantly improved efficacy. Besides, it has been reported that encapsulating drugs into CS-NPs helps overcoming P-gp efflux transporters; which increases the drug permeation through nasal mucosa. The obtained results were in congruence with the published data by Haque et al. (Citation2012) and Md et al. (Citation2013).
The complexation of GH with chitosan was thought to be advantageous over applying the free GH solution intranasally. It might have helped protecting GH against the harsh mucosal enzymatic and chemical degradation. Besides, CX-NP2 mucoadhesiveness to the nasal mucosa (Jain et al., Citation2013) delays the mucociliary clearance; which improves GH permeability. In addition, chitosan has been reported to be able to transiently open the tight junctions between the epithelial cells, thus enhancing the drug permeation to the brain and CSF (Jain et al., Citation2013). On the contrary, conventional GH nasal solution rapidly exits the nasal tract and is much more liable for mucosal enzymatic degradation (such as cytochrome P450), P-gp efflux transporters, and mucociliary clearance (Md et al., Citation2015).
Moreover, it has been reported that nanoparticles might act as intracellular drug reservoir for sustained drug release (Hu et al., Citation2013). Electron micrographs indicating the localization of CX-NP2 within the neurons will be shown later. The nanoparticles were within intracellular vesicles, possibly lysosomes, or free in the cytosol.
In conclusion, the contribution of the properties and in vivo behavior of CX-NP2 should be considered in order to justify their remarkable pharmacological efficacy over the oral GH solution.
Toxicity assessment of intranasal CX-NP2
Considering solely the toxicity of the bulk material used to prepare the nanoparticles is not the solid basis that might reflect the corresponding nanoparticles toxicity. Usually nanoparticles tend to show an entirely different behavior in vivo because of their unique small size and surface properties; which could be responsible for their toxicological events compared to their corresponding bulk material (Kreuter, Citation2014).
It was essential to investigate if the complexation reaction between GH and chitosan has affected the safety profile and immunocompatibility of the parent GH. Therefore, the effects of CX-NP2 nasal administration to male Wistar rats for 12 days on body weight, food consumption, various blood parameters, and brain histopathology were investigated.
Clinical signs, food consumption and body weight observations
Throughout the treatment period, detailed physical examinations for clinical signs of morbidity were monitored. No deaths were reported in any of the study groups. On the 13th day, a statistically significant decrease in food intake of oral GH solution treated group was observed (15 ± 1.21 g/day on the 1st day versus 12 ± 0.89 g/day on the 13th day, p < 0.05) (). On the other hand, no statistically significant changes in food consumption were observed in nasal GH solution and nasal CX-NP2 treated groups. The generally decreased food consumption in the oral GH solution treated group was accompanied by incidences of diarrhea that persisted for 3–5 days in three animals.
Table 2. Comparison of the average food consumption determined for all the study groups on the 1st and 13th day of the treatment period.
Body weight was assessed for each group on the 1st, 7th, and 13th day of treatment. No statistically significant differences in %change in body weight were observed between both the nasal GH solution and nasal CX-NP2 treated groups, and the negative control group (p > 0.05) (). On the other hand, on day 13, weight loss incidences were observed in the oral GH solution treated group, and the %change in body weight was significantly lower than the control group (p < 0.05) ().
Figure 2. The percentage change in body weight of rats determined on the 7th and 13th day of study period for oral GH solution, nasal GH solution, or nasal CX-NP2 treated groups, compared to the negative control group (data are expressed as mean ± SD, n = 6). Significant differences are indicated (*, p < 0.05). (GH, galantamine hydrobromide).
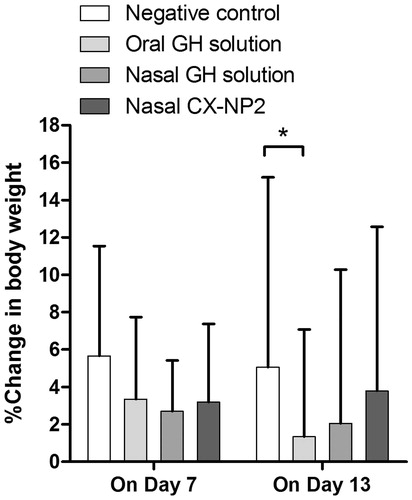
The obtained observations could indicate that administering GH nasally is accompanied by fewer incidences of gastrointestinal side effects. It has been reported by Goh et al. (Citation2011) that GH has a low-protein binding (about 18%) and a large volume of distribution (5.5 l/kg); which account for the accompanying cholinomimetic side effects due to its distribution to peripheral tissues such as nausea, vomiting, diarrhea, gastrointestinal cramping, and muscle weakness (Moffat et al., Citation2011). The significant weight loss and decreased food intake observed in the oral GH solution treated group reflect the potential side effects of administering GH orally which could be too intense in humans that the treatment should be discontinued (Hager et al., Citation2014).
Serum biomarkers and differential white blood cells count
Serum urea and creatinine concentrations essentially reflect the glomerular filtration rate. Deviations of their levels from the normal values compared to the baseline could be indicative of renal impairment (Zensi et al., Citation2009). Although the kidney is not the main target organ by CX-NP2, the effect of nasal CX-NP2 on kidney functions was evaluated to investigate any possible, direct or indirect, mechanism of toxicity. Urea and creatinine were measured in the sera of rats on the 1st and 13th days of treatment (). It is indicated in that the values of percentage change in serum urea and creatinine levels of the nasal CX-NP2 treated group were insignificantly different from those of the control group (p > 0.05). Moreover, at the end of the study, statistically significant increases of both urea and creatinine levels from the baseline were observed in the oral GH solution treated group versus the control group (p < 0.01).
Figure 3. The percentage change of serum biomarkers for kidney and liver functions between the 1st and 13th day of treatment period for oral GH solution, nasal GH solution, or nasal CX-NP2 treated groups, compared to the negative control group. (A) the percentage change in serum urea, (B) the percentage change in serum creatinine, (C) the percentage change in serum ALT, and (D) the %change in serum AST Data are expressed as mean ± SD, n = 6. Significant differences are indicated (*, p < 0.05; **, p < 0.01; ***, p < 0.001) (ALT, Alanine transaminase; AST, Aspartate transaminase; GH, galantamine hydrobromide).
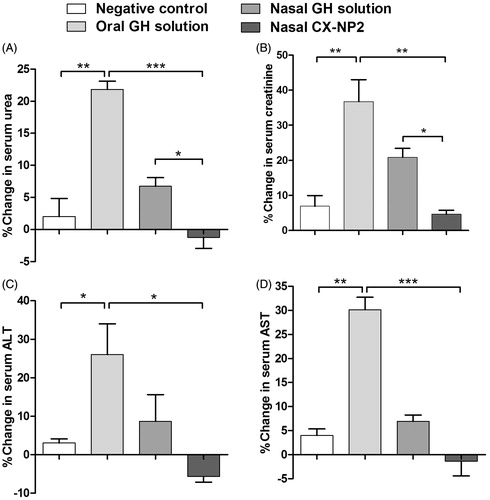
Table 3. Serum biomarkers for kidney and liver functions determined for all the study groups on the 1st and 13th day of the treatment period.
On the other hand, slightly elevated urea and creatinine levels were observed in nasal GH solution treated group versus the control group, yet considered statistically insignificant to the negative control group (p > 0.05) (). The aforementioned elevation in the percentage change of serum urea and creatinine in the nasal GH solution group might be due to the leakage of a fraction of the nasally administered GH solution to systemic blood circulation via nasal mucosa. However, negligible drug leakage is expected upon applying nasal CX-NP2 because of their mucoadhesiveness (Alam et al., Citation2012; Md et al., Citation2013). As it has not been reported previously that GH oral administration might have such elevating effect on serum urea and creatinine levels, the explanation of these results requires further investigation.
On the other hand, biochemical parameters of liver functions were assessed to evaluate the toxicity of the nasal CX-NP2 in comparison with the oral GH solution, nasal GH solution, and negative control groups (). Analyzing serum aminotransferases (AST and ALT) is a frequently used measure of hepatotoxicity. However, normally AST and ALT are present not exclusively in the liver, but also in the kidney, heart, and other tissues, where they play a significant role in energy metabolism and gluconeogenesis (Ozer et al., Citation2008). Upon cellular damage, AST and ALT tend to leak out of cells into extracellular fluids, and subsequently to the blood circulation (Ozer et al., Citation2008).
indicate the average percentage change in ALT and AST serum levels on the 13th day from the baseline determined on the first day of treatment (before administering the first dose). It is apparent that the values of %change in ALT and AST levels of the nasal CX-NP2 treated group were insignificantly different from those of the control group (p > 0.05). In fact, it appears that ALT and AST levels slightly decreased in the nasal CX-NP2 treated group by the end of the treatment period. This observation might be due to the bulk material of CS-NPs, which necessitates further investigation.
Furthermore, at the end of the study, statistically significant increases of both ALT and AST levels from the baseline were observed in the oral GH solution treated group versus the control group (p < 0.05, p < 0.01, respectively). Moreover, slightly elevated ALT and AST levels were reported for nasal GH solution treated group versus the control group by the end of treatment period, yet considered statistically insignificant (p > 0.05) (). This might be due to the leakage of a fraction of the nasally administered GH solution to systemic blood circulation via nasal mucosa. The aforementioned leakage is thought to be negligible in case of nasal CX-NP2 because of their superior mucoadhesiveness and permeation to CNS directly (Alam et al., Citation2012; Md et al., Citation2013).
WBCs are used as markers that reveal immunological responses to nanoparticles (Zhang et al., Citation2011). The elevation of WBCs count could be indicative of an inflammation encountered whether directly or indirectly (Liu et al., Citation2015). Insignificant changes in differential WBCs count were reported (p > 0.05) (Supplementary Table). It can be concluded that the repeated nasal administration of CX-NP2 did not trigger immunological responses; which indicates the biocompatibility of the prepared nanoparticles.
Brain histological examination using light microscopy
To reveal the acute brain histopathological toxicity of repeated CX-NP2 nasal administration, careful histological examination of the olfactory bulb, hippocampus, orbitofrontal and parietal cortices was performed and compared to the corresponding sections of the negative control group. It has been reported that the regeneration of the olfactory nasal epithelium is initiated within 24 h upon damage (Harkema et al., Citation2006). Furthermore, Illum et al. (Citation1994) reported that exposure of rat nasal mucosa to 0.5% w/v chitosan solutions over 1 h caused insignificant changes in mucosal cell morphology. Therefore, histological examination of olfactory nasal mucosa was not considered necessary.
It has been reported that transfer of nasally administered nanoparticles to the brain involves crossing the nasal epithelial cells to the neuronal components of olfactory bulb and trigeminal nerve, with a subsequent spreading to distant CNS locations (Ong et al., Citation2014). Therefore, olfactory bulb sections were examined for histopathological observations.
H&E-stained sections of the olfactory bulb obtained from the brains of the negative control group demonstrated six well-defined layers from outside inwards; olfactory nerve layer, glomerular layer, external plexiform layer, mitral cell layer, internal plexiform layer, and granular cell layer (). Upon investigating the corresponding olfactory bulb sections of the nasal CX-NP2 treated group, they showed the same histological layers as those of the control group. Cells appeared to be normal with no manifestations of toxicity such as cytoplasmic vacuolations, disorganization, and apoptosis ().
Figure 4:. (A) Photomicrographs of H&E-stained sections in the olfactory bulb from the negative control group (a→c) and nasal CX-NP2 treated group (a′→c′) showing six well-defined layers: 1, olfactory nerve layer; 2, glomerular layer; 3, external plexiform layer; 4, mitral cell layer; 5, internal plexiform layer; and 6, granular cell layer. (a, a′, magnification ×100; b, b′, c, c′, magnification ×400) (B) Photomicrographs of H&E-stained sections in the orbitofrontal cortex, parietal cortex, and hippocampus of the negative control group (1 → 4) and nasal CX-NP2-treated group (1′→4′). (Magnification ×400).
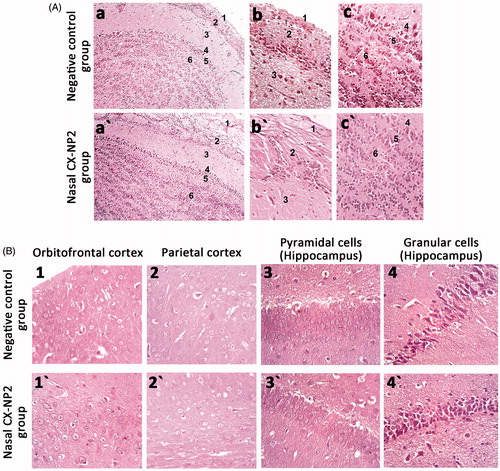
Upon investigating the localization of the fluorescent-labeled CX-NP2 in different rat brain regions 1 h after nasal administration, they were detected in the orbitofrontal cortex, parietal cortex, and hippocampus (Hanafy et al., Citation2015). Therefore, representative sections from nasal CX-NP2 treated group brains have been examined for histopathological observations and compared to the corresponding sections from the negative control group brains ().
H&E-stained sections of orbitofrontal and parietal cortices from both the negative control group and nasal CX-NP2-treated group showed large pyramidal cells with typical large nuclei (). No histopathological manifestations were observed.
On the other hand, rat hippocampus is composed of Cornu Ammonis (CA) and dentate gyrus (Abo El-Khair et al., Citation2014). CA is formed of four regions; CA1 – CA4, each appears in three layers; molecular, pyramidal, and polymorphic. CA4 region projects into the concavity of dentate gyrus; which is formed of granular cells (Abo El-Khair et al., Citation2014).
Hippocampal sections showing the pyramidal and granular cells were obtained from the negative control group. Pyramidal cells were arranged in compact layers with typical nuclei while granular cells were organized in compact laminae with dark nuclei (). Upon investigating the corresponding hippocampal sections of the nasal CX-NP2 treated group, both the pyramidal and granular cells showed normal organization without histopathological observations ().
The present findings suggest the potential safety of repeated administration of nasal CX-NP2 did not lead to brain histopathological manifestations neither locally in the olfactory bulb nor in other brain region including orbitofrontal cortex, parietal cortex and hippocampus.
Neuronal localization and toxicity assessment of CX-NP2 using electron microscopy
Di Cara et al. (Citation2007) reported that systemic administration of GH increases the extracellular ACh levels in frontal cortex of rats. Moreover, Yano et al. (Citation2009) have found that GH increases the ACh levels in the prefrontal cortex of mice to a similar extent to donepezil in spite of the fact that donepezil is 3–15 times more potent than GH at inhibiting brain AChE (Geerts et al., Citation2005). Therefore, sections of the orbitofrontal cortex from the control group and nasal CX-NP2 treated group were examined using TEM on the 13th day of treatment period. Location of the nanoparticles within neurons and ultrastructural abnormalities were reported.
Neurons are histologically identified by their large and round nuclei and a cytoplasm supplied with Nissl bodies, Golgi bodies, smooth endoplasmic reticulum, and mitochondria (Salama et al., Citation2013). As indicated in , the orbitofrontal cortex sections from the negative control group showed normal neurons. The distribution of nuclear chromatin was homogenous and the nuclear membrane was intact. Mitochondria, endoplasmic reticulum and other organelles were with normal profiles. In addition, the neuronal nerve fibers were intact and well-characterized. Upon investigating the orbitofrontal sections from CX-NP2 treated group, no significant ultrastructural changes or abnormalities were noticed. The neurons appeared to be normal with intact nuclear membrane and normal organelles.
Figure 5. Electron micrographs of (A) a neuronal cell from the orbitofrontal cortex of a rat in the control group on the 13th day of the study showing normal nuclear structure and intact organelles; (B) CX-NP2 in vitro with a diameter of 48.3 to 68.3 nm; (C)→(E) neuronal cells from the orbitofrontal cortex of a rat in the nasal CX-NP2 treated group on the 13th day of treatment period showing CX-NP2 with a diameter of 43.9 to 82.4 nm within lysosomes (indicated and magnified by the square boxes).
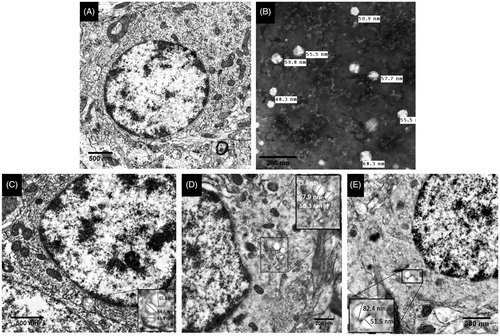
The in vitro investigation of CX-NP2 by TEM indicated their spherical to nearly spherical structure with a central dense white color. Their diameter ranges from 48.3 to 68.3 nm (). Examining orbitofrontal sections from the nasal CX-NP2 treated group revealed similar vesicular structures with a diameter ranging from 43.9 to 82.4 nm (). The nanoparticles were either within intracellular vesicles, possibly lysosomes, () or free in the cytosol (). The noticed observations were similar to those reported in a study conducted by Zensi et al. (Citation2009) to detect the brain neuronal localization of albumin nanoparticles upon their injection intravenously.
Despite the uptake of CX-NP2, the neurons remained viable. Therefore, it can be inferred that the nanoparticles’ uptake was physiological rather than because of cellular apoptosis or increased membrane porosity (Zensi et al., Citation2009). To reach brain regions such as the orbitofrontal cortex, the nanoparticles should be transcytosed via the nasal epithelial cells and diffused through the brain so as to be internalized by neurons (Zensi et al., Citation2009). Uptake of nanoparticles via this pathway involves the formation of endosomes. Presence of some nanoparticles free in the cytosol could indicate that the formed endosomes do not necessarily fuse with lysosomes. The endosomes might release the nanoparticles directly into the cytoplasm (Zensi et al., Citation2009). Interestingly, finding CX-NP2 within the orbitofrontal cortex neurons 24 h after the last administered dose reflects their prolonged residence time; which could be beneficial to aim for a less frequent dosing regimen.
This study has raised some questions seeking answers in the future research work. A pharmacokinetic study in rats should be performed to explore the residence time of GH in the rat brains after the last administered dose. This study will assess understanding the intracellular reservoir function of CX-NP2 and the possibility of their administration less frequently than conventional oral GH.
Conclusion
The presented results suggest the superiority of nasal CX-NP2 as a platform contributing to the management of AD intranasally. GH/chitosan complexation did not seem to negatively alter the pharmacological efficiency of the parent drug; GH. Intriguingly, nasal CX-NP2 exhibited a remarkable decrease of AChE protein level and activity in rat brains compared to the conventional oral and nasal GH solutions; which is considered clinically favorable. Additionally, CX-NP2-treated group showed no clinical signs of toxicity or brain histopathological manifestations, indicating their biocompatibility. Presence of CX-NP2 within brain neurons suggests their act as intracellular drug reservoirs. These results, in conjunction with their preparation simplicity and the relatively low cost of chitosan polymer, render CX-NP2 a promising nasal brain delivery system for AD management.
Supplementary material available online
Supplementary Table
Acknowledgements
The authors are grateful to Prof. Ebtehag El-Ghazawi, Emeritus Professor of Histology, Department of Histology, Faculty of Medicine, Alexandria University, for her assistance with the histopathology part of this study. The assistance from Dr. Taher Darreh-Shori, Associate Professor of Neurobiology, Department of Neurobiology, Division of Translational Alzheimer Neurobiology, Karolinska Institutet, Sweden, for interpreting the pharmacological in vivo results is appreciatively acknowledged.
Declaration of interest
The authors report no conflicts of interest. The authors alone are responsible for the content and writing of the paper. The authors report no acknowledgements for financial or editorial support. This study was funded by the authors themselves.
References
- Abo El-Khair D, El-Safti F, Nooh H, El-Mehi A. (2014). A comparative study on the effect of high cholesterol diet on the hippocampal CA1 area of adult and aged rats. Anat Cell Biol 47:117–26
- Akbarzadeh A, Rezaei-Sadabady R, Davaran S, et al. (2013). Liposome: classification, preparation, and applications. Nanoscale Res Lett 8:102–10
- Alam S, Khan ZI, Mustafa G, et al. (2012). Development and evaluation of thymoquinone-encapsulated chitosan nanoparticles for nose-to-brain targeting: a pharmacoscintigraphic study. Int J Nanomedicine 7:5705–18
- Baldrick P. (2010). The safety of chitosan as a pharmaceutical excipient. Regul Toxicol Pharmacol 56:290–9
- Bamrungsap S, Zhao Z, Chen T, et al. (2012). Nanotechnology in therapeutics: a focus on nanoparticles as a drug delivery system. Nanomedicine 7:1253–71
- Bethesda M. (1985). Committee on care and use of laboratory animals. Guide for the care and use of laboratory animals. Institute of Laboratory Animals. Resources commission on life sciences. Revised edition. NIH Publication NO. 86-23. Bethesda (MD): National Institute of Health
- Bhatt R, Singh D, Prakash A, Mishra N. (2015). Development, characterization and nasal delivery of rosmarinic acid-loaded solid lipid nanoparticles for the effective management of Huntington's disease. disease. Drug Deliv 22:931–9
- Bhattacharya S, Haertel C, Maelicke A, Montag D. (2014). Galantamine slows down plaque formation and behavioral decline in the 5XFAD mouse model of Alzheimer's disease. PloS One 9:e89454
- Botner S, Friedman A, Sintov A. (2012). Direct Delivery of intranasal insulin to the brain via microemulsion as a putative treatment of CNS functioning disorders. J Nanomed Nanotechol 3:136
- Carmo Carreiras M, Mendes E, Jesus Perry M, et al. (2013). The multifactorial nature of Alzheimer's disease for developing potential therapeutics. Curr Top Med Chem 13:1745–70
- Chen Y, Liu L. (2012). Modern methods for delivery of drugs across the blood-brain barrier. – barrier. Adv Drug Deliv Rev 64:640–65
- Contestabile A. (2011). The history of the cholinergic hypothesis. Behav Brain Res 221:334–40
- Darreh-Shori T, Hellström-Lindahl E, Flores-Flores C, et al. (2004). Long-lasting acetylcholinesterase splice variations in anticholinesterase-treated Alzheimer's disease patients. J Neurochem 88:1102–13
- Darreh-Shori T, Kadir A, Almkvist O, et al. (2008). Inhibition of acetylcholinesterase in CSF versus brain assessed by 11C-PMP PET in AD patients treated with galantamine. Neurobiol Aging 29:168–84
- Davidsson P, Blennow K, Andreasen N, et al. (2001). Differential increase in cerebrospinal fluid-acetylcholinesterase after treatment with acetylcholinesterase inhibitors in patients with Alzheimer's disease. Neurosci Lett 300:157–60
- Di Cara B, Panayi F, Gobert A, et al. (2007). Activation of dopamine D1 receptors enhances cholinergic transmission and social cognition: a parallel dialysis and behavioural study in rats. Int J Neuropsychopharmacol 10:383–99
- Dineley KT, Pandya AA, Yakel JL. (2015). Nicotinic ACh receptors as therapeutic targets in CNS disorders. Trends Pharmacol Sci 36:96–108
- Elgadir MA, Uddin MS, Ferdosh S, et al. (2014). Impact of chitosan composites and chitosan nanoparticle composites on various drug delivery systems: a review. J Food Drug Anal 23:619–29
- Ellman GL, Courtney KD, Andres V, Featherstone RM. (1961). A new and rapid colorimetric determination of acetylcholinesterase activity. Biochem Pharmacol 7:88–95
- Elnaggar YS, Etman SM, Abdelmonsif DA, Abdallah OY. (2015). intranasal piperine-loaded chitosan nanoparticles as brain-targeted therapy in Alzheimer's disease: optimization, biological efficacy, and potential toxicity. J Pharm Sci 104:3544–56
- Feldman AT, Wolfe D. (2014). Tissue processing and hematoxylin and eosin staining. Methods Mol Biol 1180:31–43
- Gage GJ, Kipke DR, Shain W. (2012). Whole animal perfusion fixation for rodents. J Vis Exp 65:35–64
- Galgatte UC, Kumbhar AB, Chaudhari PD. (2014). Development of in situ gel for nasal delivery: design, optimization, in vitro and in vivo evaluation. Drug Deliv 21:62–73
- Geerts H, Guillaumat P-O, Grantham C, et al. (2005). Brain levels and acetylcholinesterase inhibition with galantamine and donepezil in rats, mice, and rabbits. Brain Res 1033:186–93
- Goh CW, Aw CC, Lee JH, et al. (2011). Pharmacokinetic and pharmacodynamic properties of cholinesterase inhibitors donepezil, tacrine, and galantamine in aged and young Lister hooded rats. Drug Metab Dispos 39:402–11
- Hager K, Baseman AS, Nye JS, et al. (2014). Effects of galantamine in a 2-year, randomized, placebo-controlled study in Alzheimer's disease. disease. Neuropsychiatr Dis Treat 10:391–401
- Hanafy A, Farid R, El Gamal S. (2015). Complexation as an approach to entrap cationic drugs into cationic nanoparticles administered intranasally for alzheimer's disease management: preparation and detection in rat brain. Drug Dev Ind Pharm 41:2055–68
- Haque S, Md S, Fazil M, et al. (2012). Venlafaxine loaded chitosan NPs for brain targeting: pharmacokinetic and pharmacodynamic evaluation. for targetingPharmacokinetic and evaluation. Carbohydr Polym 89:72–9
- Harkema JR, Carey SA, Wagner JG. (2006). The nose revisited: a brief review of the comparative structure, function, and toxicologic pathology of the nasal epithelium. Toxicol Pathol 34:252–69
- Hu L, Sun Y, Wu Y. (2013). Advances in chitosan-based drug delivery vehicles. Nanoscale 5:3103–11
- Illum L, Farraj NF, Davis SS. (1994). Chitosan as a novel nasal delivery system for peptide drugs. Pharm Res 11:1186–9
- Irie T, Uekama K. (1997). Pharmaceutical applications of cyclodextrins. III. Toxicological issues and safety evaluation. J Pharm Sci 86:147–62
- Jain A, Gulbake A, Shilpi S, et al. (2013). A new horizon in modifications of chitosan: syntheses and applications. Crit Rev Ther Drug Carrier Syst 30:91–181
- Kreuter J. (2014). Drug delivery to the central nervous system by polymeric nanoparticles: what do we know? Adv Drug Deliv Rev 71:2–14
- Leonard AK, Sileno AP, Brandt GC, et al. (2007). In vitro formulation optimization of intranasal galantamine leading to enhanced bioavailability and reduced emetic response in vivo. Int J Pharm 335:138–46
- Li W, Zhou Y, Zhao N, et al. (2012). Pharmacokinetic behavior and efficiency of acetylcholinesterase inhibition in rat brain after intranasal administration of galanthamine hydrobromide loaded flexible liposomes. Environ Toxicol Pharmacol 34:272–9
- Lilienfeld S. (2002). Galantamine-a novel cholinergic drug with a unique dual mode of action for the treatment of patients with Alzheimer's disease. CNS Drug Rev 8:159–76
- Liu H-L, Yang H-L, Lin B-C, et al. (2015). Toxic effect comparison of three typical sterilization nanoparticles on oxidative stress and immune inflammation response in rats. Toxicol Res 4:486–93
- Lochhead JJ, Thorne RG. (2012). Intranasal delivery of biologics to the central nervous system. Adv Drug Deliv Rev 64:614–28
- Mannens G, Snel C, Hendrickx J, et al. (2002). The metabolism and excretion of galantamine in rats, dogs, and humans. Drug Metab Dispos 30:553–63
- Md S, Khan RA, Mustafa G, et al. (2013). Bromocriptine loaded chitosan nanoparticles intended for direct nose to brain delivery: pharmacodynamic, pharmacokinetic and scintigraphy study in mice model. Eur J Pharm Sci 48:393–405
- Md S, Mustafa G, Baboota S, Ali J. (2015). Nanoneurotherapeutics approach intended for direct nose to brain delivery. Drug Dev Ind Pharm 41:1922–34
- Migliore MM, Vyas TK, Campbell RB, et al. (2010). Brain delivery of proteins by the intranasal route of administration: a comparison of cationic liposomes versus aqueous solution formulations. J Pharm Sci 99:1745–61
- Moffat AC, Osselton MD, Widdop B. (2011). Clarke's analysis of drugs and poisons. London: Pharmaceutical Press
- Nordberg A, Darreh-Shori T, Peskind E, et al. (2009). Different cholinesterase inhibitor effects on CSF cholinesterases in Alzheimer patients. Curr Alzheimer Res 6:4–14
- Ong W-Y, Shalini S-M, Costantino L. (2014). Nose-to-brain drug delivery by nanoparticles in the treatment of neurological disorders. Curr Med Chem 21:4247–56
- Ozer J, Ratner M, Shaw M, et al. (2008). The current state of serum biomarkers of hepatotoxicity. Toxicology 245:194–205
- Priprem A, Chonpathompikunlert P, Sutthiparinyanont S, Wattanathorn J. (2011). Antidepressant and cognitive activities of intranasal piperine-encapsulated liposomes. Adv Biosci Biotechnol 2:108–16
- Ravi P, Aditya N, Patil S, Cherian L. (2015). Nasal in-situ gels for delivery of rasagiline mesylate: improvement in bioavailability and brain localization. Drug Deliv 22:903–10
- Salama EEA, Ali AHA, Aldahmash AM, et al. (2013). The role of vitamin e in cerebral hypoxia: an ultrastructural study. Surg Sci 4:100–6
- Sapan CV, Lundblad RL. (2015). Review of methods for determination of total protein and peptide concentration in biological samples. Proteomics Clin Appl 9:268–76
- Tumiatti V, Milelli A, Minarini A, et al. (2008). Structure-activity relationships of acetylcholinesterase noncovalent inhibitors based on a polyamine backbone. 4. Further investigation on the inner spacer. J Med Chem 51:7308–12
- Wu H, Li J, Zhang Q, et al. (2012). A novel small Odorranalectin-bearing cubosomes: Preparation, brain delivery and pharmacodynamic study on amyloid treated rats following intranasal administration. Eur J Pharm Biopharm 80:368–78
- Xu J, Shi H, Ruth M, et al. (2013). Acute toxicity of intravenously administered titanium dioxide nanoparticles in mice. PloS One 8:e70618
- Yano K, Koda K, Ago Y, et al. (2009). Galantamine improves apomorphine-induced deficits in prepulse inhibition via muscarinic ACh receptors in mice. Br J Pharmacol 156:173–80
- Yun JW, Yoon JH, Kang BC, et al. (2015). The toxicity and distribution of iron oxide-zinc oxide core-shell nanoparticles in C57BL/6 mice after repeated subcutaneous administration. J Appl Toxicol 35:593–602
- Zensi A, Begley D, Pontikis C, et al. (2009). Albumin nanoparticles targeted with Apo E enter the CNS by transcytosis and are delivered to neurones. J Control Release 137:78–86
- Zhang J, Xiong H. 2014. Brain tissue preparation, sectioning, and staining. In: Xiong H, Gendelman H, eds. Current laboratory methods in neuroscience research. New York: Springer, 3–30
- Zhang X-D, Wu D, Shen X, et al. (2011). Size-dependent in vivo toxicity of PEG-coated gold nanoparticles. Int J Nanomedicine 6:2071–81