Abstract:
Liposome-encapsulated hemoglobin (LEH) based on a novel, synthetic, non-phospholipid was developed, and evaluated for cerebral energy metabolism in a 40% hemorrhage rat model. The markers of tissue energetics were monitored by 1H- and 31P-magnetic resonance spectroscopy (MRS). After hemorrhage, 1H-MRS showed an increase in the levels of lactate and pyruvate. These markers returned to baseline values following LEH resuscitation. Both LEH and saline were able to exert a neuron-protective effect as indicated by the recovery of N-acetylaspartate. 31P MRS showed a fall in phosphocreatine after hemorrhage, which upon LEH or saline resuscitation returned to the baseline values. Similarly, inorganic phosphate increased after bleeding, but returned to normal after resuscitation. LEH resuscitation also recovered β-ATP levels, but saline resuscitation provided only a modest recovery. The results indicate the utility of MRS to monitor cerebral metabolism in hemorrhage/resuscitation. The data is also supportive of the new LEH formulation as an oxygen carrier.
INTRODUCTION
In addition to the technical requirements of pre-transfusion processing, storage, and cross-matching of blood, safety and adequate availability of blood remain a constant challenge in transfusion medicine. Artificially assembled oxygen carriers are being developed to mimic the perfusion characteristics afforded by the red blood cells (RBCs). An ideal oxygen carrier would be hemoglobin that is encapsulated and is supplemented with the oxidoreductive system of RBC [Citation1,Citation2]. One approach to compartmentalize hemoglobin is to encapsulate hemoglobin in liposomes [Citation3–8]. This encapsulated product has been variably termed hemoglobin vesicles (HbV), neo-red cells (NRC), or liposome-encapsulated hemoglobin (LEH) and contains highly concentrated (>36 g/dl) purified hemoglobin within the phospholipid membranes. Hemoglobin vesicles or liposome-encapsulated hemoglobin (LEH) mimic membrane-enclosed cellular structure of red blood cells [Citation4,Citation7,Citation9]. Compared to free modified hemoglobin preparations, LEH is characterized by spatial isolation of hemoglobin by an oxygen permeable lipid layer that eliminates the toxicity associated with free modified or unmodified hemoglobin. In addition, co-encapsulation of reductants, antioxidative enzymes, and oxygen-affinity modifiers inside liposomes is readily possible to enhance resuscitative capacity of LEH.
A major impediment in the development of LEH has been the low encapsulation efficiency of hemoglobin inside the vesicles. To increase the encapsulation of proteins inside liposomes, anionic lipids, such as dimyristoyl- and dipalmitoyl- phosphatidyl glycerol (DMPG and DPPG), are usually incorporated in the lipid composition [Citation10,Citation11]. However, anionic liposomes rapidly interact with the biological system subsequent to their opsonization with complement and other circulating proteins [Citation12–14]. Such an interaction has at least two acute consequences: a rapid uptake by the reticuloendothelial system (RES) and toxic effects, such as pseudoallergy that is manifested as vasoconstriction, pulmonary hypertension, dyspnea, drop in circulating platelets and leukocytes, etc. [Citation15,Citation16]. Since these reactions are mostly dependent on lipid dose, the problem is more challenging when huge quantities of liposomes need to be administered, such as in the use of LEH as a resuscitative fluid in acute blood loss. In this work, we report an evaluation of a LEH containing a simple anionic non-phospholipid by cerebral magnetic resonance spectroscopy (MRS) in a rat hemorrhagic shock model.
METHODS
Materials
The chemicals were obtained from Sigma-Aldrich (St. Louis, MO) and/or various suppliers through VWR Scientific (West Chester, PA) and were used without further purification. Tetradecenyl succinic anhydride was a kind gift from Vertellus Specialties Inc. (Indianapolis, IN). For liposome preparations, the phospholipids were purchased from Lipoid (Ludwigshafen, Germany), Avanti Polar Lipids (Alabaster, AL), or NOF Corporation, (Tokyo, Japan). Cholesterol was obtained from Calbiochem (Gibbstown, NJ). Outdated red blood cell units were a kind gift from Oklahoma Blood Institute (Oklahoma City, OK). For NMR and mass spectroscopy, analytical services in the Chemistry Department of the University of Oklahoma (Norman, OK) were used.
Synthesis of 2-Carboxyheptadecanoyl heptadecylamide (CHHDA)
The scheme for the synthesis of CHHDA is shown in . Tetradecenylsuccinic anhydride (4.3 g, 17.8 mmole) and hexadecylamine (2.93 g, 10.4 mmole) were allowed to react in presence of pyridine (4.5 ml) at 80°C for 3 h. The reaction mixture was extracted into dichloromethane and washed with 10% hydrochloric acid. The organic phase was concentrated to obtain a white solid of unsaturated CHHDA in 96% yield. The double bonds in unsaturated CHHDA were catalytically reduced over palladium/charcoal (5%, 40 mg) at atmospheric pressure for 16 h (yield 78%). 1H NMR (300 MHz, CDCl3): δ 5.72 (br, 1H, NH, exchanged with D2O), 3.25 (q, 2H, CH2, J = 3.6), 2.80–2.35 (m, 4H, CH2), 1.60–1.40 (m, 4H, CH2), 1.35–1.15 (m, 50 H, CH2), 0.87 (t, 6H, CH3). 13C NMR (75 MHz, CDCl3): δ 176.04, 171.43, 41.62, 37.41, 31.56, 31.51, 29.34, 29.30, 29.16, 29.00, 22.34, 13.85. ESI HRMS calculated for C34H68NO3 (M++1) 538.42, found 538.40.
Isolation of Stroma-free Hemoglobin
Concentrated stroma-free hemoglobin was isolated from the outdated red blood cell units (Sylvan Goldman Center, Oklahoma Blood Institute, Oklahoma City, OK) using a previously described method [Citation17]. To enhance the stability of hemoglobin during the isolation process, the RBCs were purged with sterile carbon monoxide gas (CO), which converts hemoglobin into a relatively more stable carbonylhemoglobin (CO-Hb). The carbonylated RBCs (20 g/dl, 300 ml) were mixed with dichloromethane (DCM, 60 ml) for 10 min. The precipitate was allowed to settle, and the supernatant was re-extracted with DCM three times to remove all the lipid material that is soluble in organic phase. The residual DCM in the aqueous phase containing CO-Hb solution was removed under vacuum in an R-210 rotavapor (Buchi Corporation, New Castle, DE) at 40°C. The resultant CO-Hb solution was further heated at 60°C for 1 hr to denature and precipitate any methemoglobin in the solution. The precipitate was removed by centrifugation at 8,000 rpm for 20 min at 4°C in a Sorvall RC-5B refrigerated Superspeed centrifuge. The supernatant was purified from fine remnants of particulate matter by sequentially passing it through tangential-flow FiberFlow capsules having 100 and 50 nm cut-off (Minntech, Minneapolis, MN). Finally, the purified hemoglobin solution was concentrated to about 38 g/dl using a 30 kDa cut-off Prep/Scale-TFF filter cartridge (Millipore, Billerica, MA). The final preparation was characterized for oxygen affinity, CO-Hb content, MetHb concentration and endotoxin.
Preparation of Liposome-encapsulated Hemoglobin (LEH)
The LEH was prepared in several steps described in detail elsewhere [Citation18]. A brief description of the procedure is given below.
A thin film of 1,2-disteroyl-sn-glycero-3-phosphatidylcholine (DSPC), cholesterol (CHO), anionic lipid, 1,2-disteroyl-sn-glycero-3-phosphoethanolamine-N-[monomethoxy poly(ethylene glycol) (5000)] (DSPE-PEG5000) and vitamin E in 38.85:38.85:20:0.3:2 mol% was obtained. The lipid film was rehydrated with sodium hydroxide solution equimolar to the carboxyl groups present in the anionic lipid to maintain total lipid concentration to 12 mM. The pH of the lipid suspension was adjusted to 7.0. This suspension of large multilamellar vesicles was subjected to 8 Freeze-thaw (FT) cycles. An FT cycle consisted of snap-freezing the suspension in liquid nitrogen followed by immediate thawing in a 58°C water bath. After the final FT cycle, the pro-liposome suspension was shell-frozen in a lyophilization flask and subjected to a 48 h lyophilization cycle in a Triad Lyophilizer (Labconco, Kansas City, MO).
Oxygen affinity of hemoglobin was modified by adding pyridoxal-5′-phosphate (PLP, 2.5 molar times of hemoglobin) to the carbonylhemoglobin solution used for rehydration of lyophilized pro-liposomes. Strict aseptic conditions were maintained throughout the preparation in a laminar flow hood. Final size reduction of hemoglobin containing pro-liposomes was carried out by high-pressure homogenization in Emulsiflex-C3 at 20K psi for 4 cycles, each cycle separated by at least 30 min. The processing temperature was maintained at about 20°C. Further processing of LEH was performed according to the method earlier published [Citation19,Citation20]. The free hemoglobin was separated from encapsulated hemoglobin by tangential-flow filtration through 50 nm hollow fiber filter using PBS (pH 7.4) as the diluting solvent. The purified LEH was post-inserted with PEG-lipid by mixing an aqueous solution of DSPE-PEG5000 with a dilute dispersion of LEH under conditions where PEG-lipid concentration remains below its critical micelle concentration. To convert encapsulated carbonylhemoglobin into oxyhemoglobin, the PEGylated LEH was exposed to a bright visible light from a 500W halogen lamp under saturating oxygen atmosphere at 4–8°C. The conversion was monitored spectrophotometrically. The dilute oxygenated LEH was further subjected to tangential-flow filtration using 50 nm filter to eliminate remaining free hemoglobin. Finally, the LEH was concentrated and stored at 4°C.
Characterization of LEH
The LEH preparations were characterized for hemoglobin content, methemoglobin, size, oxygen affinity, and lipid concentration. Hb encapsulation was estimated by monitoring the absorbance of the OBG lysate of LEH at 540 nm [Citation21]. The phospholipid concentration was determined by Stewart assay [Citation22]. Methemoglobin content was also measured [Citation23]. Oxygen affinity (p50) was measured in a Hemox-analyzer (TCS Scientific, New Hope, PA). Briefly, a 50 μl sample of LEH was dispersed in a 4 ml phosphate buffer (pH 7.4) containing albumin and an antifoaming agent. The mixture was incubated at 37°C for 5 minutes and aspirated into the cuvette for dual wavelength spectrophotometry. The sample was purged with N2 gas to PO2 < 2.0 mm Hg, before allowing air to attain PO2 >140 mm Hg. The results were analyzed by HAS software provided with the instrument.
The particle size was determined by photon correlation spectroscopy using a Brookhaven particle size analyzer equipped with Mas Option software. Zeta potential of preparations was measured in a Zeta PLUS Zeta potential analyzer (Brookhaven Instruments Corp., Holtsville, NY). Zeta potential of LEH was estimated, both before (non-PEGylated LEH) as well as after (PEGylated LEH) PEG post-insertion. Preparations equivalent to about 40 μg of phospholipid in 1.5 ml of 0.22 μm-filtered de-ionized water were scanned at 25°C for 10 runs, each run consisting of 20 cycles. Zeta potential values were obtained as millivolt ± standard error of mean.
Rat Model of Hemorrhagic Shock and Resuscitation
The animal experiments were performed according to the NIH Animal Use and Care Guidelines and were approved by the Institutional Animal Care Committee of the University of Oklahoma Health Sciences Center, Oklahoma City. The rat hypovolemic exchange transfusion model has been described earlier [Citation24]. A constant volume protocol was used for induction of shock because it has been suggested that it imitates a clinical scenario more closely than the constant-pressure protocol [Citation25]. The left femoral artery of male Sprague Dawley rats (225–450 g) was cannulated with a polyethylene catheter. The catheter consisted of Tygon tubing (0.02″ × 0.06″) coupled to a 2.5″ PTFE tip (28G). The catheter was subcutaneously tunneled, secured at the nape and filled with heparin (1000 U/ml). After closing the surgical area, the rats were given 2 days to recover from the procedure. On the day of the study, the rats were anesthetized by isoflurane anesthesia (2 % in 1.8 L/min medical air) and instrumented to digitally monitor rectal temperature and arterial blood pressure (iWorx, Dover, NH). Mean arterial blood pressure and hematocrit were monitored and presented as percent change compared to the baseline values.
For MRS, the anesthetized animal was positioned inside the MRI system. The imaging study was conducted according to the flow chart shown in . After allowing the animal to stabilize, spectroscopy was performed to obtain a baseline spectrum. Imaging was performed at the Nuclear Magnetic Resonance Core Facility (Oklahoma Medical Research Foundation) in a 7 Tesla-30 cm horizontal bore magnet (Bruker Bio-Spin MRI, Germany). With the help of a syringe pump (Fusion 100, Chemyx Inc.), 40% of circulating blood was withdrawn using the femoral artery catheter at 0.5 ml/min. The total blood volume was estimated as 5.7% of total body weight. A 2.5 hr stabilization period was allowed post-bleeding, before spectroscopy was performed to obtain post-bleed spectrum. At the end of post-bleed spectroscopy, the animals received isovolemic resuscitation with either saline or LEH (0.5 ml/min). A control group of animal was not resuscitated with any fluid. After resuscitation, another 2 h period was allowed before third spectroscopy to obtain post-resuscitation spectrum.
Magnetic Resonance Spectroscopy (MRS) and Spectral Analysis
The animals were anesthetized using 2% isoflurane in medical air. During the entire data acquisition the animal body temperature was maintained to 37°C using heating pads. Baseline spectra were obtained prior to femoral artery cannulation. The anesthetized animals were placed in an MR probe (72 mm multi-rung volume coil for transmission, and a rat head coil for reception) in a prone position. Morphological T2-weighted images (20 transverse slices with a thickness of 1 mm and taken in a field of view of 3.5 × 3.5 cm2) were acquired using a spin echo method, with a repetition time (TR) of 2400ms, an echo time (TE) of 64ms and 6 averages, for a total acquisition time of 10 min. 1H magnetic resonance spectroscopy (MRS) data was acquired using a point resolved spectroscopy (PRESS) method, in a 3 × 3 × 3 mm3 voxel with a TR of 2500 ms, a TE of 24 ms, VAPOR water suppression and 256 averages, for a total acquisition time of 15 min. 31P MRS data was acquired using a 31P surface coil (2 cm diameter), single-pulse method, with a TR of 2500ms and 256 averages, for a total acquisition time of 10 min.
Initial processing of the MRS data (line broadening and Fourier transform) was conducted with the Paravision 4.0 software (Bruker BioSpin MRI, Germany). Each spectrum was then phased, calibrated on the water peak (4.78 ppm, 1H MRS) or the phosphocreatine peak (0.0 ppm, 31P MRS) and the area under each peak of interest was calculated, using a Mathematica-based program. For 1H MRS data, the peaks of interest were creatine (Cr, 3.03 ppm), lactate (Lac, 1.32 ppm), pyruvate (Pyr, 2.4 ppm), N-acetyl-aspartate (NAA, 2.02 ppm), choline (Cho, 3.22 ppm), and glutamine/glutamate (Glu/Gln, 3.77 ppm). The 31P peaks of interest were, phosphocreatine (PCr, 0.0 ppm), ATP γ, α, and β (at −2.37, −7.48 and −16.2 ppm, respectively), phosphomonoesters (PME, 6.87 ppm), inorganic phosphate (Pi, 4.72 ppm) and phosphodiesters (PDE, 3.2 ppm) [Citation26,Citation27].
RESULTS AND DISCUSSION
The overall therapeutic goal of resuscitation in hemorrhagic shock is to maintain perfusion and metabolic status in the tissues [Citation28]. The usual practice of infusing crystalloids or colloids corrects the salt-imbalance and the circulatory volume deficit in hemorrhagic shock, but falls short of increasing the oxygen-carrying capacity in circulation. Hemoglobin-based oxygen carriers not only increase oxygen-carrying capacity, but also the cardiac preload [Citation29]. For several reasons, Hb-based substitutes of RBCs are being developed as oxygen therapeutics for use in accidental blood loss, major surgery, or organ transport [Citation30,Citation31]. Besides being free of infectious organisms, these products may be stored for a relatively long period of time and used as universal product in recipients of all blood types. Liposome-encapsulated hemoglobin has recently commanded particular attention because of its lower toxicity compared to the molecularly modified forms of free hemoglobin. It is an oxygen carrier that mimics the membrane- enclosed cellular structure of red blood cells with respect to both the oxygen transport and its eventual metabolic disposition in the reticuloendothelial system (RES) [Citation4,Citation7,Citation9]. Moreover, encapsulated hemoglobin has better cardiovascular/hemodynamic profile as compared to the unencapsulated forms of hemoglobin [Citation32–36].
An important issue in resuscitation with oxygen carriers is the relationship between the oxygen-carrying capacity in circulation and oxygen utilization in the perfused tissue bed. It has not been clearly established whether the enhanced oxygen-carrying capacity in blood is reflected in an improved oxygen delivery and tissue energetics in a hypoxic organ. In addition to the hemodynamic parameters, monitoring oxygen delivery and tissue metabolism is of paramount significance in hemorrhagic shock. Earlier, we pioneered the use of state-of-the-art small animal positron emission tomography (PET) and a positron-emitting isotope of oxygen, oxygen-15 (15O) as a physiological tracer for determining cerebral oxygen metabolism. We evaluated the capacity of LEH to improve cerebral metabolic rate of oxygen (CMRO2) in a 40% hypovolemic shock model of rat. We also compared the performance of LEH with saline, shed blood, and 5% HSA as control resuscitation fluids. The results revealed that 15O-PET technology can be successfully employed to evaluate oxygen carriers and blood substitutes, and that LEH resuscitation delivers oxygen to cerebral tissue and improves oxygen metabolism in brain [Citation24].
Several other groups of investigators have used indirect techniques of measurement, and have shown that LEH is capable of correcting oxygen deficit and improve systemic markers of metabolism [Citation35,Citation37–39]. A correction in volume deficit may not necessarily mean recovery in cellular metabolism. In this work, we utilized magnetic resonance spectroscopy to evaluate cerebral energy metabolic status in a rat model of hemorrhagic shock resuscitated with an improved formulation of LEH. The LEH formulation consisted of phospholipids and cholesterol supplemented with 28% of a novel anionic non-phospholipid. The characteristics of the LEH are detailed in .
Table 1. Properties of LEH.
Hematocrit and Mean Arterial Blood Pressure (MAP)
Through the entire study, animals were kept anesthetized and their vital signs were noted periodically. Core body temperature was successfully maintained at 37±1°C by using a warm water blanket. Hematocrit in animals subjected to hypovolemia dropped 27.5% from 42.2 ± 0.76 to 30.6 ± 0.72. There was no significant difference in the values of hematocrit in various groups at baseline and post-bleed stages. The supernatant in the hematocrit tube after centrifugation of samples from LEH-resuscitated animals showed the presence of LEH in plasma. shows the changes in MAP in animals with hemorrhagic shock and resuscitation. After induction of hypovolemia, MAP decreased in correlation with the amount of blood loss. The MAP values at baseline and after hemorrhage were not significantly different among saline and LEH groups. Saline resuscitation improved MAP only marginally, and the MAP values were still significantly different from the normal baseline values. On the other hand, LEH consistently improved MAP to about 95% of the baseline blood pressure. The outcome may be reflective of the capacity of LEH to transport oxygen. Perhaps the ability to transport oxygen subdues physiological feedback mechanisms in response to acute blood loss.
LEH Tends to Restore Cerebral Energy Metabolism
The effectiveness of LEH on cerebral energy metabolism was evaluated in a 40% hemorrhagic shock model in rats using proton and phosphorous magnetic resonance spectroscopy (1H-MRS and 31P-MRS). The representative 1H and 31P spectrum of a voxelated region encompassing part of the cortex and corpus striatum is shown in . The peak assignments were performed based on Pfeuffer et al. [Citation40] and Braunona et al. [Citation26] for 1H and 31P spectra, respectively. The entire duration of the study was about 6 h. The survival in the non-resuscitated group was only 62% of the rats (8/13). All animals given saline transfusion survived the 6 h duration. There was 9% casualty in LEH-transfused rats (1/11), which occurred within 5 minutes of resuscitation and was later found related to a bad LEH preparation exposed to environmental factors for a prolonged period under refrigeration.
Figure 4. Both 1H and 31P spectra were acquired at the same location, encompassing part of the cortex and corpus striatum (voxel shown as square).

The levels of markers of anaerobic metabolism, like lactate and pyruvate, increased post-bleeding but recovered closer to baseline levels after resuscitation with LEH (). The observations that hemorrhage results in the appearance of a lactate peak in 1H MRS, and that the lactate peak disappears after LEH resuscitation, suggest that LEH resuscitation is able to alter post-bleeding anaerobic metabolism in brain to an aerobic one (). On the other hand, saline resuscitation could not affect such metabolic alteration. Similarly, pyruvate was restored by LEH resuscitation, but remained high in the cases of saline infusion ().
Figure 5. The ratios of various metabolites with respect to creatine obtained from 1H spectroscopy: (a) lactate; (b) lactate plus lipid; (c) pyruvate; (d) glutamate-glutamine; (e) N-Acetyl aspartate; and (f) choline.
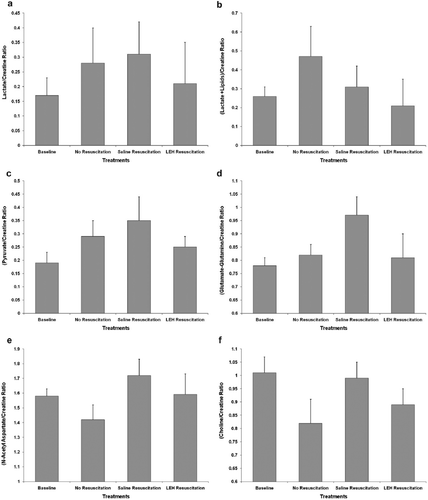
Glutamate-glutamine (Glu-Gln) metabolism is indicative of protein synthetic activity. Because the restoration of protein synthesis is a lagging indicator of metabolic status, it is possible that the impact of resuscitation was expected to be subdued in spectroscopic findings. Even then LEH resuscitation was found to maintain Glu-Gln peaks closer to the baseline as compared to the saline resuscitation (). N-acetyl aspartate (NAA) is another lagging marker that is almost exclusively present in intact neurons, but absent in other brain cells [Citation41]. Any reduction in NAA correlates with neuronal death and a decline in neuron density. After bleeding, we observed a slight decrease in NAA levels that were restored both by LEH as well as saline resuscitation (). From the results it appears that mere correction of volume deficit and ensuing improvement in cerebral blood flow may be sufficient to correct NAA levels; however, this conclusion may remain a mere speculation, unless a larger study with more severe hemorrhage is conducted.
Yet another indicator of cerebral metabolism in MRS is choline (Cho) at 3.2 ppm. The intensity of Cho peak is indicative of cellular density [Citation41]. For instance, cellular tumor regions show high 1H MRS Cho and necrotic regions show low Cho. In our hemorrhagic shock model, the Cho peak was significantly subdued after bleeding, but was restored close to baseline by LEH resuscitation (). Saline resuscitation, on the other hand, appears to over-compensate the Cho peak in this model. The significance of this is not clear at present, but further studies are desirable to adequately address this observation.
The information obtained after 31P MRS more or less endorsed the efficacy of LEH in improving tissue metabolism. The levels of phosphocreatine (PCr) fell after bleeding, which is an indication of PCr consumption as a source of energy via creatine kinase pathway (). An increase in inorganic phosphate (Pi) levels post-bleeding () suggests breakdown of adenosine triphosphate (ATP). Both these markers return to their baseline levels after LEH, as well as saline resuscitation. The differences between the two resuscitation fluids in this regard were insignificant. However, there was a difference in ATP metabolism between the animals resuscitated with saline or LEH (). For comparative purposes, the changes in ATP levels were monitored by following the β-ATP peak because γ-ATP and α-ATP peaks in the spectrum show some overlap from α- and β-adenosine diphosphate (ADP). The fall of β-ATP after bleeding correlates well with the post-bleed increase in Pi increment (). It recovers, and even crosses baseline levels after LEH resuscitation. The improvement in β-ATP levels after saline resuscitation was modest as compared to that observed after LEH administration. The critical requirement of oxygen in ATP synthesis is demonstrated by these results.
Figure 6. Values of various phosphorus metabolites obtained from 31P spectroscopy: (a) phosphocreatine; (b) inorganic phosphate; (c) total ATP; (d) β-ATP; (e) α-ATP; and (f) phosphomonoesters.
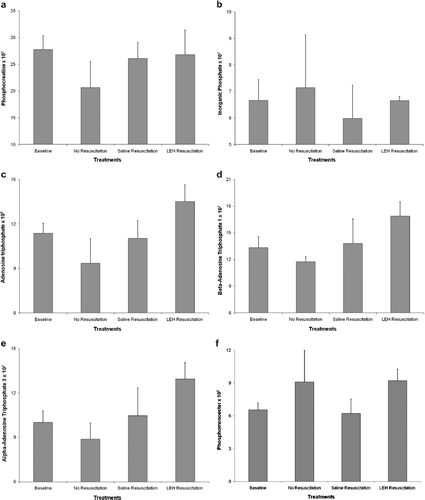
Phosphomonoesters or PME in brain tissue is a precursor of cell membrane phospholipids, and a high PME level indicates enhanced synthesis of phosphorylcholine and phosphorylethanolamine. In tumors, for instance, an increase PME levels are frequently reported, but the changes in PME levels after treatment as a reflection of therapeutic benefit are not found to be consistent and remain controversial [Citation42]. In a hemorrhagic shock model in porcine, elevation in muscle PME levels was found predictive of observed mortality [Citation43,Citation44]. As expected, the post-bleed spectrum in our animal model reported an increase in cerebral PME (). This increase resolved in animals receiving resuscitation with saline, but remained elevated in animals resuscitated with LEH. In light of all other physiological and metabolic improvements conferred by LEH resuscitation, we believe that the elevated PME levels post-LEH resuscitation are reflective of a large amount of lipid dose administered in the animals. The reason for this assumption is the fact reported by several investigators that contribution in the 31P PME peaks is from several elements inclusive of phospholipid-related metabolites [Citation45,Citation46]. It will be interesting to see a group of control animals infused with plain liposomes for confirmation of this hypothesis.
CONCLUSIONS
Clearly, MRS may be of some utility in monitoring tissue energetics after resuscitation. A drawback of the technique appears to be the long data acquisition time (∼1 h) inclusive of the time necessary to perform appropriate shimming operations. Keeping small animals under anesthesia for such a prolonged time after bleeding and resuscitation may not be a recommendable step. Our results demonstrate that the trend followed by the metabolites is indicative of a transition of aerobic energy metabolism to anaerobic pathway after bleeding, and its return to aerobic pathway after resuscitation, more so with LEH resuscitation. In order to obtain statistically significant (p<0.01 or 0.05) differences in the results, it may be necessary to either enhance the severity of shock (from 40% to ∼70% bleeding, or to prolong the duration of shock. The typical signatures of hemorrhagic shock, like a high mortality in the non-resuscitated group [Citation44,Citation47] and a significant drop in ATP level (expected only after complete PCr depletion), were absent in our study. This suggests that with a 40% blood withdrawal, the shock was not of a severe nature. Even then the following important observations were recorded:
The appearance of the post-bleed lactate peak; under normal conditions the lactate peak is not detected in 1H MRS.
The trends that various metabolites follow and the correlation amongst them represent a known energy metabolism pattern.
In ongoing experiments, we have performed LEH resuscitation in 60% of a hemorrhagic shock model in rats and observed 100% survival without any apparent distress and weight loss over a period of 3 months (data not shown). Our further goal is to non-invasively monitor cerebral glucose metabolism in this animal model and correlate the outcome with the results obtained from cerebral tissue energetic and oxygen metabolic studies.
Declaration of interest: The authors report no conflicts of interest. The authors alone are responsible for the content and writing of the paper.
REFERENCES
- Chang, T.M., . (2000). Two future generations of blood substitutes based on polyhemoglobin-SOD-catalase and nanoencapsulation. Adv Drug Deliv Rev 40(3): 213–8.
- Yu, W.P., T.M. Chang. (1996). Submicron polymer membrane hemoglobin nanocapsules as potential blood substitutes: preparation and characterization. Artif Cells Blood Substit Immobil Biotechnol 24(3): 169–83.
- Awasthi, V. (2005). Pharmaceutical aspects of hemoglobin-based oxygen carriers. Curr Drug Deliv 2(2): 133–42.
- Phillips, W.T., . (1999). Polyethylene glycol-modified liposome-encapsulated hemoglobin: a long circulating red cell substitute. J Pharmacol Exp Ther 288(2): 665–70.
- Takaori, M., A. Fukui. (1996). Treatment of massive hemorrhage with liposome encapsulated human hemoglobin (NRC) and hydroxyethyl starch (HES) in beagles. Artif Cells Blood Substit Immobil Biotechnol 24(6): 643–53.
- Usuba, A., . (1994). Effect of neo red cells on hemodynamics and blood gas transport in canine hemorrhagic shock and its safety for vital organs. Artif Cells Blood Substit Immobil Biotechnol 22(3): 503–16.
- Sakai, H., . (1996). Physical properties of hemoglobin vesicles as red cell substitutes. Biotechnol Prog 12(1): 119–25.
- Farmer, M.C., . (1988). Liposome-encapsulated hemoglobin: a synthetic red cell. Adv Exp Med Biol 238: 161–70.
- Rudolph, A.S. (1995). Encapsulation of hemoglobin in liposomes. Blood Substitutes: Physiological Basis of Efficacy, R.M. Winslow, K.D. Vandegriff, M. Intaglietta. Birkhauser, Boston, 90–104.
- Drummond, D.C., . (1999). Optimizing liposomes for delivery of chemotherapeutic agents to solid tumors. Pharmacol. Rev. 51(4): 691–743.
- Walde, P., S. Ichikawa. (2001). Enzymes inside lipid vesicles: preparation, reactivity and applications Biomol. Eng. 18: 143–177.
- Miller, C.R., . (1998). Liposome-cell interactions in vitro: effect of liposome surface charge on the binding and endocytosis of conventional and sterically stabilized liposomes. Biochemistry 37(37): 12875–83.
- Semple, S.C., A. Chonn, P.R. Cullis. (1998). Interactions of liposome and lipid-based carrier systems with blood proteins: Relation to clearance behavior in vivo. Adv. Drug Delivery Rev. 32: 3–17.
- Szebeni, J. (1998). The interaction of liposomes with the complement system. Crit Rev Ther Drug Carrier Syst 15(1): 57–88.
- Awasthi, V.D., B. Goins, W.T. Phillips. (2007). Insertion of poly(ethylene glycol)-lipid reduces the liposome-encapsulated hemoglobin-induced thrombocytopenic reaction. Am J Pharmacol Toxicol 2: 98–105.
- Szebeni, J., . (2000). Liposome-induced pulmonary hypertension: properties and mechanism of a complement-mediated pseudoallergic reaction. Am J Physiol Heart Circ Physiol 279(3): H1319–28.
- Sakai, H., . (1993). Purification of concentrated hemoglobin using organic solvent and heat treatment. Protein Expr Purif 4(6): 563–9.
- Agashe, H., . (2009). Improved formulation of liposome-encapsulated hemoglobin with an anionic non-phospholipid. Journal of Colloid and Interface Science B (in press).
- Awasthi, V.D., . (2004). Kinetics of liposome-encapsulated hemoglobin after 25% hypovolemic exchange transfusion. Int J Pharm 283(1–2): 53–62.
- Awasthi, V.D., . (2004). Neutral and anionic liposome-encapsulated hemoglobin: effect of postinserted poly(ethylene glycol)-distearoylphosphatidylethanolamine on distribution and circulation kinetics. J Pharmacol Exp Ther 309(1): 241–8.
- Tomita, S.E.Y., Santa, M., Yoshida, H., Yasumitsu, Y. (1968). A simple spectrophotometric method for determination of met-hemoglobin in dilute solution. J Nara Med Assoc 19: 1–6.
- Stewart, J.C.M. (1980). Colorimetric determination of phospholipids with ammonium ferrothiocyanate. Anal. Biochem. 104: 10–14.
- Matsuoka, T. (1997). Determination of methemoglobin and carboxyhemoglobin in blood by rapid colorimetry. Biol Pharm Bull 20(11): 1208–11.
- Awasthi, V., . (2007). Cerebral oxygen delivery by liposome-encapsulated hemoglobin: a positron-emission tomographic evaluation in a rat model of hemorrhagic shock. J Appl Physiol 103(1): 28–38.
- Bellamy, R.F., P.A. Maningas, B.A. Wenger. (1986). Current shock models and clinical correlations. Ann Emerg Med 15(12): 1392–5.
- Braunova, Z., . (2000). Metabolic changes in rat brain after prolonged ethanol consumption measured by 1H and 31P MRS experiments. Cell Mol Neurobiol 20(6): 703–15.
- Jelicks, L.A., R. Gupta. (1992). 31P-NMR of high-energy phosphates in perfused rat heart during metabolic acidosis. Am J Physiol 263(3 Pt 2): H903–9.
- Hirschl, R.B. (1994). Oxygen delivery in the pediatric surgical patient. Curr Opin Pediatr 6(3): 341–7.
- Baron, B.J., T.M. Scalea. (1996). Acute blood loss. Emerg Med Clin North Am 14(1): 35–55.
- Stowell, C.P. (2002). Hemoglobin-based oxygen carriers. Curr. Opin. Hematol., 9: 537–543.
- Winslow, R.M. (2003). Current status of blood substitute research: towards a new paradigm. J Intern Med 253(5): 508–17.
- Kawano, T., H. Hosoya. (2002). Oxidative burst by acellular haemoglobin and neurotransmitters. Med Hypotheses 59(1): 11–5.
- Nakai, K., . (1994). Acellular and cellular hemoglobin solutions as vasoconstrictive factor. Artif Cells Blood Substit Immobil Biotechnol 22(3): 559–64.
- Rudolph, A.S., . (1997). Liposome encapsulation attenuates hemoglobin-induced vasoconstriction in rabbit arterial segments. J Appl Physiol. 82(6): 1826–35.
- Sakai, H., . (2002). Systemic and microvascular responses to hemorrhagic shock and resuscitation with Hb vesicles. Am. J. Physiol. Heart Circ. Physiol. 283: H1191–H1199.
- Usuba, A., R. Motoki. (1995). Safety and efficacy of encapsulated hemoglobin in hemorrhagic shock. Artificial Red Cells: Materials, Performances and Clinical Study as Blood Substitutes, E. Tsuchida. John Wiley & Sons Ltd., Chichester, England, 65–92.
- Izumi, Y., . (1997). Evaluation of the capabilities of a hemoglobin vesicle as an artificial oxygen carrier in a rat exchange transfusion model. Asaio J 43(4): 289–97.
- Plock, J.A., . (2005). Is hemoglobin in hemoglobin vesicles infused for isovolemic hemodilution necessary to improve oxygenation in critically ischemic hamster skin? Am J Physiol Heart Circ Physiol 289(6): H2624–31.
- Yoshizu, A., . (2004). Hemorrhagic shock resuscitation with an artificial oxygen carrier, hemoglobin vesicle, maintains intestinal perfusion and suppresses the increase in plasma tumor necrosis factor-alpha. Asaio J 50(5): 458–63.
- Pfeuffer, J., . (1999). Toward an in vivo neurochemical profile: quantification of 18 metabolites in short-echo-time (1)H NMR spectra of the rat brain. J Magn Reson 141(1): 104–20.
- Miller, B.L., . (1996). In vivo 1H MRS choline: correlation with in vitro chemistry/histology. Life Sci 58(22): 1929–35.
- Igata, A., T. Asakura, T. Fujimoto. (2000). MRS of the Brain and Neurological Disorders, Terada International, Inc./Institute of Advanced Medical Technology, Tokyo.
- Taylor, J.H., . (2004). Phosphomonoesters predict early mortality in porcine hemorrhagic shock. J Trauma 56(2): 251–8.
- Taylor, J.H., . (2004). Tissue energetics as measured by nuclear magnetic resonance spectroscopy during hemorrhagic shock. Shock 21(1): 58–64.
- Franks, S.E., . (2002). Phosphomonoester concentrations differ between chronic lymphocytic leukemia cells and normal human lymphocytes. Leuk Res 26(10): 919–26.
- Boulanger, Y., M. Labelle, A. Khiat. (2000). Role of phospholipase A(2) on the variations of the choline signal intensity observed by 1H magnetic resonance spectroscopy in brain diseases. Brain Res Brain Res Rev 33(2–3): 380–9.
- Mann, D.V., . (1997). Superiority of blood over saline resuscitation from hemorrhagic shock: a 31P magnetic resonance spectroscopy study. Ann Surg 226(5): 653–61.