Abstract
Abstract: In this work, positively charged, micelle-forming polymers were synthesized and used as a model vector to deliver antisense oligodeoxynucleotide (ASODN) into melanoma cells. Polymers and polymer/ASODN complexes were characterized by DLS according to size, charge, and critical micelle concentration. Nanosize and spherical complexes were observed by AFM. Complexes did not reveal significant toxicity to melanoma cells. Antiproliferative effect of the complexes was observed by immunocytochemical staining and estimated as 56.8% with N/P:9. High amount of apoptosis and very small amount of necrosis were estimated. According to the results, these positively charged polymers forming micelle-like structures seem promising as ASODN carriers.
INTRODUCTION
Gene delivery has great potential to cure many genetic and acquired diseases. Non-viral gene delivery is especially advantageous because it could avoid unacceptable immune responses. Various cationic polymers have shown promising effects in facilitating gene delivery as they readily complex with DNA to form polyplexes by neutralizing the negatively-charged anionic phosphate groups and hence improve transfection. Poly(ethylene imine) (PEI) is able to effectively complex even large DNA molecules [Citation1,Citation2], leading to homogeneous spherical particles with a size of 100 nm or less that are capable of transfecting cells efficiently in vivo as well as in vitro. It offers a significantly more efficient protection against nuclease degradation than other polycations, e.g. poly(L-lysine), possibly due to their higher charge density and more efficient complexation. However, further research is needed to rationalize the relationship between polymeric molecular architecture and the structure of polymer/DNA complexes formed [Citation3].
Amphiphilic block copolymers composed of hydrophilic and hydrophobic segments can form a micellar structure with a hydrophobic compact inner core and a hydrophilic swollen outer shell in selective solvents, which is thermodynamically favorable for one block but unfavorable for the others [Citation4]. These micelles are an interesting group of self-assembled nanocarriers for drug delivery, owing to the prospects of biocompatibility and large solubilization capacity for poorly watersoluble molecules. Poly(ethylene glycol)-b-poly(ε-caprolactone) (PCL-b-PEG) and poly(ethylene glycol)-b-poly(L-lactide) (PLL-b-PEG) diblock copolymers are very promising due to their stability and biocompatibility. Micelles formed of these copolymers have been utilized in encapsulation of different lipophilic drugs [Citation5]. Note that it is also possible to prepare nanocapsules or nanoparticles from these diblock copolymers in drug or active agent delivery. In Chang et al.'s study, PEG-PLA in the form of ultrathin membrane nanocapsules (80–150 nm) prepared as red blood cell (rbc) substitutes containing hemoglobin (Hb) and enzymes were reported [Citation6].
Copolymers formed between cationic polymers such as PEI and the aforementioned diblock copolymers offer the benefit of electrostatic complexation while enhancing solubility and stability of the polyplexes. Insertion of hydrophilic segments (PEG) into diblock copolymers provides a hydrophilic corona that creates a steric barrier against self-aggregation, shielding of cationic charge groups, and inhibits the association with plasma proteins and other cellular components. Moon et al. [Citation7] described the synthesis of diblock, triblock, and 4 arm star-shaped block PEG–PTMC copolymers and the characteristics of micelle as a potential drug delivery vehicle. Addition of a positively charged unit to these copolymers can allow their use as gene delivery vehicles.
ASODNs can either hybridize with specific mRNA, which would inhibit the transcription of genetic information into protein synthesis, or activate the RNase H that can digest the mRNA resulting in the pathway blockage. These oligonucelotides targeted to oncogenes are widely used in order to provide specific and effective means to prevent the growth of cancer cells. c-myc, a member of the myc gene family, is a key regulator of cell growth that has been found altered by amplification or point mutation in a large subset of tumors, including leukemia, melanoma, prostate, breast, and colon carcinomas [Citation8,Citation9]. The efficacy of these oligonucleotides in inhibiting gene expression depends on their ability to penetrate targeted cells. The uptake of them occurs through active transport, which, in turn, depends on the temperature, the structure, and the concentration [Citation10]. Several approaches have been explored to enhance cellular uptake and permeability in order to increase their therapeutic efficacy, including conjugation to polycations, targeting cell surface receptors, coupling to cholesterol, and encapsulation into lipid particles such as microspheres and liposomes. The use of vectors in antisense drug delivery in vivo seems to improve their biological effects.
Growth inhibition of SK-MEL-30 human melanoma cells by antisense c-myc oligonucleotides delivered by poly(N-Isopropylacrylamide)/poly(ethyleneimine) copolymer was reported by our group. By this polymer, we have only investigated the c-myc inhibition by MTT assay [Citation11]. In this study, we have reported the synthesis and characterization of PEI coupled PCL-mPEG and PLL-mPEG diblock copolymers having the ability to form micelle-type structures for potential use in delivery of c-myc antisense oligonucleotide to melanoma cells. All polymers were investigated by Zeta Sizer for their size and charge characteristics. Protonation ability of polymers was followed by this technique using different pH values. Also, complexation ability of ASODNs with prepared positively charged polymers was investigated by measuring their sizes and charges in different N/P ratios. AFM was also used to examine the corresponding micelle-type structures. After physicochemical characterization of polymer ASODN complexes, we have investigated the c-myc inhibition efficiency of these complexes. Apoptosis and necrosis indexes were evaluated. Results showed that these particular carriers would be potential candidates in the uptake of oligonucleotides to melanoma cells.
MATERIALS AND METHODS
Materials
Methoxy poly(ethylene glycol) (Mn: 5000, Fluka, Germany; Mn: 2000, Sigma-Aldrich, Germany), poly(ethylene glycol) (PEG, Mn: 2000 and 5000 Sigma-Aldrich, Germany), ε-caprolactone (ε-CL, Aldrich, USA), stannous octoate ((SnOct2), Sigma, USA), diethyl ether, chloroform, and dichloromethane were used as received. L-Lactide (Aldrich, USA) was recrystallized with toluene. Branched polyethyleneimine (PEI, MW: 2000, Aldrich, Germany) was used as received. Carbonyldiimidazole (CDI) was obtained from Sigma (Germany). TNBS (2,4,6-trinitrobenzene sulfonic acid) and pyrene were purhased from Sigma-Aldrich (Germany). A 15-mer AS ODN (5′-AACGTTGAGGGGCAT-3′) that was complementary to the translation initiation region of c-myc oncogene was obtained from Iontek Ltd. (Turkey) and used in all experiments. Purity was >95% provided by high performance liquid chromatograpy.
Methods
Preparation of positively charged PCL-mPEG and PLL-mPEG diblock copolymers. PCL-mPEG and PLL-mPEG diblock copolymers carrying two different methoxy PEG molecules (Mn: 2000 and 5000) were prepared by microwave irradiaton using Milestone S.r.l MicroSYNTH microwave synthesis unit. Details for synthesis and characterization were reported elsewhere [Citation12]. For the preparation of PEI-carrying polymers, diblock copolymers (PCL-mPEG, PLL-mPEG) in 4:1 ratio were used because of their water solubility. In the coupling procedure carbonyldiimidazole was used to activate the hydroxyl group of polymers. The polymer sample was dissolved in dioxane in a concentration of 8×10−3 M. Then, 0.08 M CDI was added to the medium and the reaction mixture was stirred for 3 h at 37°C. At the end of the reaction, the mixture was precipitated from diethyl ether. This procedure was repeated three times and the obtained product was dried in vacuum overnight. Coupling of the activated polymer to the PEI by amino groups was then performed. CDI-activated polymer was dissolved in borate buffer (pH:8.5) and PEI (2000, branched) was added to the medium in 1:1 ratio (mole). The reaction mixture was incubated at room temperature for 24 h. Unreacted polymers were removed using an ultrafiltration system (Millipore) by taking into account the molecular weight of corresponding polymers as reported elsewhere [Citation12]. The product was liophilized for further use.
TNBS assay. In gene delivery applications, the complexation of polymer and genetic material (here, oligonucleotide) depends on the amount of amino groups of polymer and phosphate groups of ODN. It is not possible to know the exact amount of amino groups of branched PEI (MW: 2000) molecule used here. Therefore, there is a need to estimate the amount of primary amine groups for complexation studies. Amino-group content of PEI-bound polymers was investigated by the TNBS (2,4,6-trinitrobenzene sulfonic acid) method [Citation13]. To do this, a calibration curve was obtained using glycine amino acid in different concentrations (0.5, 0.1, 0.05, 0.01, 0.001 mg/ml) at pH: 8.5. TNBS was added in a ratio of 0.1% and the reaction mixture was incubated at 40°C for 2 h. Following incubation, each sample was measured at 335 nm by UV-Visible Spectrophotometer and recorded. Amine group content of each polymer was estimated from the calibration curve. In addition to this method, gel permeation chromatography (GPC) was also used to confirm polymer formation by using PEG as standard.
Preparation of polymer/ASODN complexes. Stock solutions of ODN (5.68.10−6 M) and polymer (1 mg/ml) were prepared in a phosphate buffer. Complexes were prepared by adding ASODN solution to the polymer solution in desired amounts to achieve different N/P ratios. Here, polymer amounts were used according to their amine content estimated by TNBS assay. After mixing of ASODN and polymer with mild shaking, the sample was left at room temperature for 30 min to equilibrate before use.
Dynamic Light Scattering (DLS). DLS measures the intensity correlation function of light scattered from a polymeric solution. All DLS measurements were performed using a Malvern Instruments NanoS Nanosizer. Samples analyzed were contained in a 1 cm-path length cell, and the data were analyzed using Malvern Instruments Dispersion Technology Software. The polymer refractive index was taken to be 1.5 with an absorbance of 0.01. The viscosity and refractive index of water were taken as 0.8877 cPa and 1.330, respectively. Six measurements were performed on each sample, with an average of 10 runs taken for each measurement, each within 1 min. We have estimated the critical micelle concentration by using data obtained by this instrument. In addition, changes in zeta potentials and sizes of PEI-coupled polymers were evaluated in different pHs. Polymer/ASODN interaction was followed by the same instrument in different N/P ratios at physiological pH.
Atomic Force Microscopy (AFM) Complex solutions were deposited onto freshly cleaved mica surfaces and dried at room temperature for 5 min. The images were observed by Tapping Mode using the AFM microscope (SPM9600, Shimadzu, Japan) with 10 nm silicon nitride tips. Analysis was performed to investigate the size and images of selected polymers and ASODN complexes.
Cell Culture. SK-MEL30 melanoma cells were obtained from Hacettepe University, Biochemistry Department. The cells were cultured in Dulbecco's Modified Medium without L-glutamine (DMEM, Sigma, Germany) supplemented 10% Fetal Bovine Serum (FBS, Sigma, Germany), and 1% penicilin-streptomycin (Sigma, Germany) at 37°C and 5% CO2 atmosphere. The cells were subcultured twice a week, using dissocation medium, trypsin-EDTA, pH 7,4 as buffer system. All tests were triplicated. Statistical significance of diferences between test values was estimated using Nonparametric Kruskal-Wallis test. p < 0.1.
MTT assay. For MTT experiments, cells were cultured in 96-well plates at a density of 5×103 per well and grown overnight at 37°C, 5% CO2 atmosphere. The cell culture medium was removed and then replaced. Copolymer/ASODN complexes formed at different N/P ratios were added into each well. Cells were incubated for 72 h. MTT reagent (5 mg/ml) was added into each well, and the cells were cultured for a further 4h incubation. After that, 100 μl 10% sodium dodecyl sulfate (in 0.01 N HCl) were added to each well and incubated overnight. Then, plates were read in Elisa Microplate Reader at 570 nm. Dark blue formazan crystals are formed if the cells are alive.
Cell transfection. Cells were seeded on cover glasses in 6 well plates at a density of 10×103 per well and grown overnight at 37°C, 5% CO2 atmosphere. The cell medium was removed and washed with PBS three times. After polymer/ASODN complexes formed at N/P ratio: 1, 3, 6, and 9 were added into each well for 24 hours. Only ASODN was used as a control.
Immunocytochemical detection of c-myc expression in cell culture. SK-MEL 30 human melanoma cells (10×103 cells per well) were placed on cover glasses in DMEM without L-glutamine in 6-well plates and incubated at 37°C in 5% CO2 atmosphere. Then these cells were washed with distilled water and polymer/ASODN complexes in different N/P ratio (1, 3, 6, and 9) were applied to cells for 24 hours. Following that, slides stained for c-myc antigen were cytocentrifuged, fixed in cold acetone at −20°C for 10 minutes, and treated with hydrogen peroxide (0.3% in methanol) for 10 min to block the endogenous peroxidase activity. Then they were washed in PBS and incubated with c-myc antibody (Santa Cruz, 1:100 dilution) at room temperature for 1 h. As a negative control, preimmune mouse serum, instead of primary antibody, was used. For immunohistochemical demonstration of the c-myc protein, an avidin-biotin-peroxidase technique was used [Citation14]. The peroxidase-antiperoxidase technique [Citation15] was used to demonstrate the c-erbB-2 protein. The immunoreactivity of the c-myc antibody was confined to the cytoplasm and nuclear of SKMEL-30 cells. We counted the number of c-myc positive cytoplasmic and nuclear staining cells in all fields found at 400× final magnification. c-myc positive cells were seen to be brown under a light microscope. For each slide, three randomly selected microscopic fields were observed and at least 1000 cells/field were evaluated.
Analysis of apoptosis and necrosis. Double staining was performed to quantify the number of apoptotic cells in the culture, based on scoring apoptotic cell nuclei. SKMEL-30 cells (20×103 cells per well) were placed in DMEM using 24-well plates. After treating with polymer/ASODN complex at N/P ratio of 1,3,6 and 9 (in aqueous solutions) for a 24-hour period, both attached and detached cells were collected, washed with PBS, and then stained with Hoechst dye 33342 (2 μg/mL), propodium iodide (PI) (1 μg/mL) and DNAse free-RNAse (100 μg/mL) for 15 min at room temperature. Then, 10–50 μL of cell suspension was smeared on the slide and cover slip for examination using fluorescence microscopy. In addition, attached cells were washed with PBS and stained in plates in the same manner. While the nuclei of normal cells were stained light blue, apoptotic cells were stained bright blue by the Hoechst dye. The apoptotic cells, from their nuclear morphology, were identified as a nuclear fragmentation or chromatin condensation. Necrotic cells were stained red by PI. Necrotic cells lacking plasma membrane integrity allow PI dye to cross the cell membrane, meaning that PI dye does not cross the non-necrotic cell membrane. The results of the counted number of apoptotic and necrotic cells in 10 randomly chosen microscopic fields were expressed as a ratio of apoptotic and necrotic to normal cells. The number of apoptotic and necrotic cells were determined by Fluorescence Inverted Microscope (Leica, Germany) using DAPI and FITC filters.
RESULTS
Polymer Characterization
As mentioned in earlier, PCL-mPEG and PLL-mPEG diblock copolymers were synthesized by microwave irradiaton and polymer fromation was confirmed by FTIR and 1H-NMR. Molecular weight data were obtained from GPC and also 1H-NMR spectra. All data were reported elsewhere [Citation12]. Here, we have prepared the PEI-bound form of these diblock copolymers. After a series of purfication steps by ultrafiltration, pure PEI-bound polymers were obtained and characterized by different techniques. Addition of PEI into the diblock copolymer structure was confirmed by TNBS assay and GPC as explained below.
shows representative unimodal GPC traces of PCLPEG5000 and PCLPEG5000/PEI. The addition of PEI to the diblock copolymer caused higher molecular weight value as seen here and the obtained polymers after PEI conjugation seem close to monodispersitiy.
Determination of Amino-group Content
The PEI molecule used in this study has a branched structure containing tertiary, secondary, and primary-amino groups, which are not easy to determine with direct methods. It is important to estimate the amino content of the corresponding polymers since the quantity of especially primary amino-groups is required for the complexation with genetic material by electrostatic interaction. Here, we have performed TNBS assay and obtained approximate values. Each sample reacted with TNBS was measured at 335 nm by UV-Visible Spectrophotometer and amine group content of each polymer was estimated from the calibration curve (y=12816x+0.3117, R2=0.9871). In this way, the success of PEI-coupling to polymers was also demonstrated. Amine content of each polymer was obtained as 0.14, 0.10, 0.15, 0.15 mg/ml for PCL-mPEG2000/PEI, PCL-mPEG5000/PEI, PLL-mPEG2000/PEI, PLL-mPEG5000/PEI, respectively.
Light Scattering Measurements
The charge and size of the polymer has great influence on the stability, DNA-binding, and release efficiency of polyplexes, and thus on targeting efficiency. Several studies reported the importance of positive charge of different polymers to be used in gene delivery [Citation1–3,Citation19–22].
DLS detects the mean dimension of particles in aquous soluiton based on the Stocks-Einstein equation. Measurements were made for positively charged diblock copolymers and polymer/ASODN conjugates.
Determination of cmc. As explained in the Methods section, the micelle forming ability of PCL-mPEG and PLL-mPEG diblock copolymers and their PEI-bound forms were investigated by a Zeta Sizer. shows intensity vs. concentration curves of diblock copolymers. Up to a certain concentration value (0.05 mg/ml), intensity did not reveal any significant increase; however, after this value, a sharp increase in intensity was observed for all polymers. This certain concentration can be accepted as criticial micelle concentration (cmc) [Citation16]. Note that by this concentration value a remarkable decrease in size was also observed, probably caused by ordered micelle structure formation. This increase was observed for lactide-based copolymers, which meant that miscellar structure forms more easily with these polymers. Addition of PEI to the diblock copolymer structure did not affect very much the micelle formation for each of the polymers. Micelle formation is expected to be easy for diblock copolymers since there is only one hydrophilic and one hydrophobic block. In the PEI-bound case, the total polymer chain posseses two hydrophilic chains in both ends and one hydrophobic in the middle. Therefore, a micelle formation may be suggested, as in . We did not obtain very different cmc values because all diblock copolymers and their PEI-bound forms do not possess different structures in terms of hidrophobic and hydrophilic balance. For the PEI-bound polymers, the concentration was not different form 0.05 mg/ml. Zhang et al. reported the CMCs of poly(D,L-Lactide)-b-mPEG copolymers in the range of 3.5–0.5 mg/L changing by PDLLA length in the polymer chain [Citation17]. Gyun et al. found CMC of methoxy propyleneglycol/caprolactone micelles as between 3.47–0.63.10−7 mol/lt [Citation4].
Size and charge characterization of PEI-coupled diblock copolymers. The following results can be drawn from size and charge analyses: For diblock copolymer, PCL-mPEG2000, size was determined as 138 nm without PEI. Size of PEI-including copolymer (PCL-mPEG2000-PEI) at pH: 2 and 10 were determined as 89 and 204 nm, respectively (). However, at pH:7.4, the size of this polymer was 184 nm. By increasing pH, PEI units (especially between pH: 2–8) become protonated and start to swell (proton sponge effect), and this causes an increase in the hydrodynamic size of the polymer. This behaviour does not occur after pH: 8, which is the upper pKa value of PEI. Charge of this polymer was also evaluated (). At acidic pHs, the polymer gained a positive charge as expected (+6.57 at pH:2 and +13,17 at pH: 4), since PEI has a great protonation capacity in acidic medium towards even higher pH values (+16.8 at pH: 7.4 and (+16.1 at pH: 8) [Citation1]. However, at very basic medium (such as pH: 10), the polymer was not positively charged any more (close to zero).
For PCL-mPEG5000-PEI, while size was 100 and 195 nm at pH:2 and 10, respectively, it was 175 nm at pH:7.4. The size of the diblock form of this polymer was 176 nm at pH:7.4, proving no negative effect on particle formation of PEI-additon to the structure. This polymer has similar behavior to PCL-mPEG2000PEI having smaller size and charge in lower pH values. It was positively charged until pH: 8 (+10.2), but negatively charged after this pH (-2.38 at pH: 10). PLL-mPEG2000-PEI was positively charged until pH:7.4 (+ 4.86, +24.6, +14.1, +18.8 at pH: 2, 4, 7, 7.4, respectively), but negatively charged at pH: 10 (-8.29). Size increased to 163 nm (at pH: 7.4); after that, this increase reached up to 336 nm at pH: 10, probably caused by aggregation. The size of the diblock form of this polymer was 137 nm at pH:7.4, which is little different from PEI-bound from (163 nm). PLL-mPEG5000-PEI was positively charged until pH:7.4 (+4.87, +16.6, +16.5, +18.3 at pH: 2, 4, 7.4, 8), but negatively charged at pH 10 (-8.41). Size was 162 nm at pH: 7.4, whereas it was 111, 109, 163 and 235 nm at pH: 2, 4, 8 and 10, respectively. This revealed that good micelle formation was achieved at physiological pH. Note that the size of PLL-mPEG5000 was 154 nm. The particle sizes of all polymers were in a similar range. Measured sizes here are probably much higher than the original value because the Zeta Sizer instrument measures the hydrodynamic size of the particles. PEI addition to the polymer structure was confirmed by these analyses. It was also demonstrated that the PEI molecule had high protonation capacity, which makes PEI very attractive as an efficient gene delivery vector [Citation1,Citation2].
Polymer/ASODN conjugates. For size and charge of polymer/ASODN conjugates, polyplexes were prepared in different N/P ratios (1, 3, 6, 9) by taking into account the amino content calculated from TNBS analysis. All polymers revealed sizes in a similar range because of the similarity of resulting structures. According to (PCL-mPEG2000-PEI/ASODN), mixing of ODN leads to a small increase in the diameter (polymer's diameter was 184 nm). As the concentration of polymer increases (increase in N/P ratio), the size of polyplexes decreased to 120 nm (at N/P:9). Thus, N/P:6 seems the best ratio for good complexation because of the smallest polydispersity. This can be seen also from , representing volume intensity distribution profiles plotted against hydrodynamic diameters. Note that size is not the only determinant in efficient transfection.
Figure 5. Light scattering profiles of PCL-mPEG2000-PEI/ASODN complexes at different N/P ratios (1, 3, 6, 9).
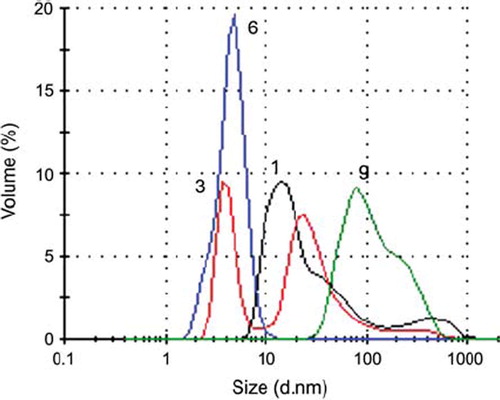
Table 1. Charge and size of polymer/ASODN complexes measured by DLS in different N/P ratios
PCL-mPEG5000-PEI/ASODN: charge of polymer showed a similar trend with the previous polymer sample. Here, the smallest complex size (∼140 nm) was obtained at N/P:6 and 9, in which polyplexes had an extra positve charge (+4–5 mV). PLL-mPEG2000-PEI/ASODN: increasing of size by N:P ratio may cause aggregation of complexes, so that up to N:P:6 seems efficient enough. At this ratio, complex has extra surface charge. PLL-mPEG5000-PEI/ASODN: size of polyplexes increased up to N/P: 6. N/P: 9 seems the best ratio at which size is smallest and charge is highest.
Atomic Force Microscopy (AFM)
shows SPM image of PCL-mPEG2000/PEI/ASODN complexes in different N/P ratios. Complexes were prepared by adding ODN solution to the polymer solution in desired amounts to achieve different N/P ratios. After mixing of ASODN, the sample was left at room temperature for 30 min to equilibrate before use. Sapmles were dropped onto freshly celaved mica and dried at room temperature. As clearly seen here, complexes formed spherical particles, which can be supposed to be micelle. In some regions, a kind of aggregates were formed, which can be overcome by preparation of more diluted sample. However, in all ratios very small structures were obtained (between 10–30nm). More uniform particles were formed in N/P ratio of 6. In N/P:1, very small particles were obtained but at the same time they seemed to have a tendency to be aggregated. In N/P ratio 1 and 9, more polydispersity was observed comparing to N/P: 6, as also seen in DLS profiles (). The size observed by AFM seems inconsistent with the ones obtained by DLS, but this was expected since the DLS instrument measures the hydrodynamic volume of the sample. The results showed that formation of spherical structures obtained by polymer alone was not affected in the presence of ASODN.
MTT Assay
ASODN did not reveal any toxicity towards SKMEL-30 cells. Polymers (100µM) without ASODN showed toxicities caused by positive charge on the polymer surface. Cell viability after polymer/ASODN transfection was obtained as seen in While positive charge enhanced toxicity directly, size increase did not affect this as much as charge. MTT assay of polymer/ASODN complexes depending on time was also evaluated. No significant difference was observed in cell viability between 48 and 72 hour incubation time. Similar results were obtained for the poly(N-Isopropylacrylamide)/poly(ethyleneimine) complexed with the same ASODN in terms of polymer toxicity and incubation time [Citation11].
Cell Transfection by Polymer/ASODN Complexes and c-myc Expression
c-myc expression was followed by immunocytochemical staining, as mentioned earlier. Polymer/ASODN complexes in different N/P ratios were transfected into SKMEL-30 cells seeded on cover glass. Naked oligonucletide was used as a control and revealed only 5% c-myc inhibition. By the polymers without ASODN cell inhibition was also observed (4.3–7.7%), but this was most likely because of the toxic effect of unoccupied positively charged polymer chains. Results are shown in and c-myc activites are illustrated in . Most of the complexes showed a certain amount of cell inhibition as seen in . As previously reported in several studies, transfection efficiency decreased with decreasing positive charge density [Citation3]. In the low N/P ratio range, it is expected that some of the ASODNs are not complexed with polymer. That means complexes are not going to be formed and therefore this will cause reduced transfection. Zhao et al. reported that lowering the N/P ratio to below 1 resulted in lower transfection efficiency [Citation18].
Table 2. c-myc expression index of SKMEL-30 cells obtained by immunocytochemistry staining method (p=0.071)
Figure 8. Immunocytochemistry images of stained SKMEL-30 cell with c-myc antibody. (A) Nucleus of cell (stained with c-myc antibody), where brown spots indicate c-myc positive cells as a control (non-treated with polymer/ASODN complex). (B) Cells transfected with PCL-mPEG5000/PEI at N/P: 9; blue spots show c-myc negative cells. (C) Cells transfected with PCL-mPEG2000/PEI at N/P: 9; arrow shows c-myc positive cells and some of cells nuclei blue as c-myc negative cell. (D) Cells transfected with PLL-mPEG2000/PEI at N/P: 3; arrow shows c-myc-positive cell. (E) Cells transfected with PCL-mPEG5000/PEI at N/P: 1; arrow showss c-myc positive cell. (F) Cells transfected with PLL-mPEG5000/PEI at N/P: 3; arrow shows c-myc positive cell. Images were recorded with ×400 magnification and with an Olympus research microscopy.
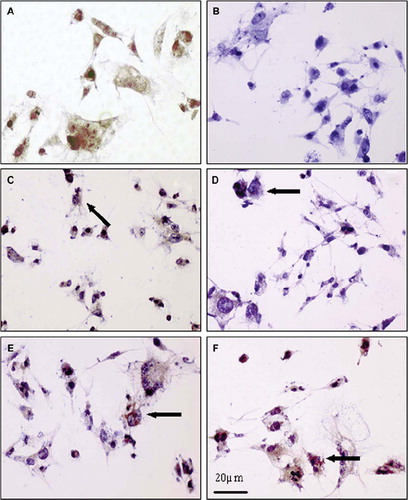
shows c-myc positive cells seen as brown spots transfected with only naked oligonucleotide. The following images were taken after transfection by the complexes formed between polymers and ASODN. As seen in these images, complex formation caused lower c-myc activity, especially at lower N/P ratios and higher particle sizes. The decrease in complex size and increase in complex charge allowed enhanced oligo uptake into the cells; therefore inhibited c-myc activity was seen as blue spots in the . It seems that the charge of the complexes is more effective on the c-myc expression more than size difference. Most of the complexes actually are in a similar size range especially seen in AFM images. c-myc expression was inhibited as 56.8% and 49.1% with transfection by PCL-mPEG2000/PEI in N/P: 9 and PLL-mPEG2000/PEI in N/P: 3, respectively ( and ). When the complex charge reached a very high value, toxicity was observed. Polymers without oligonucleotide revealed a similar trend with the control group.
Apoptotic and Necrotic Effect of Complexes
Apoptosis results are in a consistency with c-myc activity. Apoptotic cell ratio was obtained as 3% and this ratio was increased by transfection of polymer/ASODN complexes depending on the charge and particle sizes (). As seen in the control experiment, all of the cell nuclei were stained by Hoechst 33342 as light blue representing non-apoptotic cells (). Polymer/ASODN complexes caused apoptosis as seen in bright blue spots (). The highest apoptotic effect was observed in high N/P ratios. This might be because of the free positive charge on the complex facilitating cell entrance and endosomal escape. For most of the polymers, N/P: 9 seems a good ratio for the efficiency. Although toxicity of the polymers without oligonucleotide were increased by size and charge, apoptotic effect was observed in lower values. Apoptosis results seem in consistency with MTT assay.
Table 3. Apoptotic and necrotic indexes of SKMEL-30 carcinoma cells; result obtained with double staining method (p=0.085 for Apoptotic indexes, p=0.069 for Necrotic indexes).
Figure 9. Fluorescence inverted microscopy image of SKMEL-30 carcinoma cells. (A) Nucleus of cells (stained with Hoescht 33342), where blue spots indicate nucleus of non-apoptotic cells as a control. (B) Shining and smashed nucleus (shown with arrow) of apoptotic cells in PCL-mPEG2000/PEI (N/P;9) containing medium. (C) Fluorescence microscopy image of cells (stained with Hoescht 33342), where formation of green cells demonstrates non-necrotic cells (photo taken under FITC filter) as a control. (D) Nucleus of SKMEL-30 cells (stained with PI and Hoescht 33342), where dense red spots indicate nucleus of necrotic cells and green cells indicate non-necrotic cells. Images were recorded with ×400 magnification. Photos A and B were taken under DAPI filter and C and D taken under FITC filter.
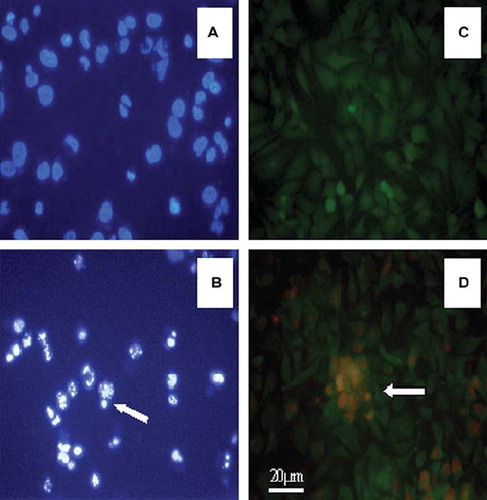
In the necrotic effect study, naked ASODN did not cause necrosis; however, 100μM polymers revealed in between 10%–15% necrosis. Complexation between polymer/ASODN affected necrosis in a way that was increased by the N/P ratio and therefore by the charge of the complexes. As seen in , the higher the positive charge, the more necrotic cells were obtained. Necrotic cells appeared in red (PLL-mPEG2000/PEI/ASODN complex at N/P:9), while the others were in green (stained by Hoechst 33342). represents the control group not containing polymers and appears in green.
DISCUSSION
This study reported the use of PEI-coupled PCL-mPEG and PLL-mPEG diblock copolymers in ASODN delivery to SKMEL-30 cells. Diblock copolymers were synthesized by microwave irradiation and characterized as reported elsewhere [Citation12]. Here, the PEI-bound form of these diblock copolymers was prepared and polymer formation was demonstrated by TNBS assay and GPC.
DLS was used to investigate the charge and size characteristics of polymers and also polymer/ASODN complexes. The micelle forming ability of PCL-mPEG and PLL-mPEG diblock copolymers and their PEI-bound forms was investigated by this technique and it was estimated that a sharp increase in intensity after 0.05 mg/ml was observed for all polymers, which can be accepted as cmc. It was observed that the charge of the polymers increased, especially in the acidic medium, because of the great buffering capacity of the PEI molecule. Obtained micelle-like structures and complexes were confirmed by DLS in terms of polydispersity and optimal N/P ratio was found as 6. AFM images of polymer/ASODN complexes showed that spherical structures were formed also in the presence of ASODN. However, the sizes of the corresponding structures were in the range of 10–30 nm, approximately, which were different from the ones obtained by DLS. This was because of the DLS technique measuring the hydrodynamic size of the resultant complexes that were higher than the original ones.
According to MTT assay, polymers not carrying ASODN showed some toxicity because of the free positive charge of PEI. MTT assay for the polymer/ASODN complexes depending on charge and incubation time was consistent with another study performed by poly(N-Isopropylacrylamide)/poly(ethyleneimine) [Citation11].
Expression of c-myc was followed by immunocytochemical staining. Naked oligonucletide showed only 5% c-myc inhibition. A small amount of inhibiton (4.3– 7.7%) was also observed for the polymers without ASODN, probably because of the toxicity related to positive charge. Most of the complexes showed a certain amount of cell inhibition, as seen . For example, c-myc inhibiton ratio was estimated as 56.8% and 49.1% with PCL-mPEG2000/PEI in N/P: 9 and PLL-mPEG2000/PEI in N/P: 3, respectively. For the efficient inhibition, lower N/P ratios did not seem as appropriate as the higher ones because of insufficient complex formation.
Several cationic polymers containing hydrophilic PEG blocks have been used as transfecting vectors for different genes [Citation19–20]. In the case of PEI as a cationic part, the high transfecting efficiencies observed have been attributed to the strong buffering capacity under the physiological pH conditions. PEI buffers inside the endosomes due to the transfected polycations pump protons into the endosomes; this is called the proton sponge effect. This causes an increase in the osmotic pressure inside the endosome, which destabilizes the endosome, resulting in the escape of the gene complexes from the degradation inside the lysosomal environment.
Gebhart et al. [Citation21] and Ochietti et al. [Citation22] have reported that grafting of a hydrophobic polymeric block to a cationic PEI block can lead to the enhancement of transfection efficiency and control of favorable biodistribution of the complexes in animal models. They have suggested that by tuning the amphiphilicity of copolymers the binding with gene molecules can be made more effective, resulting in a favorable size range of the nanopolyplexes. They have also speculated that amphiphilicity together with the variation of block size would allow one to examine the effect of hydrophobic interaction on the nanostructure and net charge density of the oligonucleotide polyplexes. The relationship between such information with cell transfection and cytotoxicity needs to be studied for more efficient results [Citation19].
In this study, c-myc activity was not demonstrated by only MTT and immunocytochemical staining. Apoptosis and necrosis indexes were also evaluated. Here, complexes caused apoptosis in the cells while it was obtained only 3% by the polymer alone. For most of the polymers, N/P: 9 seems a good ratio for the efficiency. On the other hand, necrosis caused by the polymers increased by the N/P ratio depending on the unoccupied positively charged chains. It was concluded that the optimal N/P ratio has to be estimated in order not to cause necrosis but at the same time good apoptosis related to c-myc inhibition. According to the results, the most efficient N/P ratio seems to be between 6 and 9.
CONCLUSIONS
In this study we have prepared polymers and polymer/ASODN complexes and used DLS and AFM to characterize the size and charge characteristics. We have investigated these propeties in different N/P ratios. After all, these complexes were evaluated by their c-myc activity towards SK-MEL 30 cells together with apoptotic and necrotic effect.
Since a very small oligonucleotide was used in this study, shrinkage of genetic material was not expected that much, which was in the case of using large DNA chains. DLS experiments showed that the N/P ratio affected the uniformity of the complexes, as also seen in AFM images. All polymers seem to have the ability of micelle forming as demonstrated by DLS and AFM. We obtained very small particles after complexation, but the most uniform ones were obtained especially in N/P:6. The cell culture studies showed that N/P:9 was also accepable in terms of c-myc transfection and inhibition. Complexes showed apoptotic activity, while a small amount of necrotic behavior was observed, possibly because of positive charges. Since all polymers are similar in terms of structural properties, they did not reveal a great difference in corresponding inhibition activity. According to the results, these polymers seems to have a potential to improve intracellular availability for c-myc directed antisense strategies.
Declaration of interest: The authors report no conflicts of interest. The authors alone are responsible for the content and writing of the paper.
REFERENCES
- Godbey, W.T., Wu, K.K., Mikos, A.G. (2001). Poly(ethylenimine)-mediated gene delivery affects endothelial cell function and viability. Biomaterials, 22:471–480.
- Piskin, E., Dinçer, S., Turk, M. (2004). Gene delivery-intelligent but just at the beginning. J. Biomater. Sci. Polymer Edn, 15:1181–1202.
- Park T.G. . (2006). Current status of polymeric gene delivery systems. Advanced Drug Delivery Reviews, 58:467–486.
- Gyun, S.I., Yeon, K.S., Moo, L.Y., Soo, C.C., Kiel, S.Y. (1998). Methoxy propyleneglycol/caprolactone amphiphilic block copolymeric micelle containing indomethacin. I. Preparation and characterization. J of Controlled Release, 51:1–11.
- Forresr, M.L., Yanez, J.A., Remsberg, C.M., Ohgami Y., Kwon G.S., Davies, N.M. (2008). Paclitaxel prodrugs with sustained release and high solubility in poly(ethylene glycol)-b-poly(ε-caprolactone) micelle nanocarriers: pharmacokinetic disposition, tolerability, and cytotoxicity. Pharmaceutical Research, 25, 1:194–206.
- Chang, T.M., Powanda, D., Yu, W.P. (2003). Analysis of polyethylene-glycol-polylactide nano-dimension artificial red blood cells in maintaining systemic hemoglobin levels and prevention of methemoglobin formation. Artif Cells Blood Substit Immobil Biotechnol., 31(3):231–47.
- Moon, S.K., Hoon, H., Byung, S.K., Gilson, K., Hai Bang, L. (2008). Polymeric nano-micelles as drug carrier using polyethylene glycol and polytrimethylene carbonate linear and star-shaped block copolymer. Current Applied Physics., 8:646–650.
- Vlassov, V.V., Balakireva, L.A., Yakubov, L.A. (1994). Transport of oligonucleotides across natural and model membranes. Biochim Biophys Acta, 1197(2):95–108.
- Yakubov, L.A., Deeva, E.A., Zarytova, V.F., Ivanova, E.M., Ryte, A.S., Yurchenko, L.V., Vlassov, V.V. (1989). Mechanism of oligonucleotide uptake by cells: involvement of specific receptors? Proc. Natl. Acad. Sci. USA 86:6454–6458.
- Dang, C.V. (1999). c-myc target genes involved in cell growth, apoptosis, and metabolism. Mol Cell Biol, 19(1):1–11.
- Dinçer, S., Oskay, E.K., Piskin A.K., Zeybek, N.D., Pişkin E. Growth inhibition of SK-MEL-30 human melanoma cells by antisense c-myc oligonucleotides delivered by poly(N-isopropylacrylamide)/poly(ethyleneimine) copolymer, J of Tissue Eng and Reg Medicine, DOI: 10.1002/term.239.
- Karagöz, A., Dinçer, S. Microwave-assisted synthesis of poly(ε-caprolactone)-block-poly(ethylene glycol) and poly(lactide)-block-poly(ethylene glycol), Macromolecular Symposia, DOI: 10.1002/masy.200900170.
- Habeeb, A. (1996). Determination of free amino groups in proteins by trinitrobenzenesulfonic acid. Analytical Biochemistry, 14:328–336.
- Pavelic, K., Pavelic, Z.P., Denton, D., Reising, J., Khalily, M., Preisler, H.D. (1990). Immunohistochemical detection of c-myc oncoprotein in parafin embedded tissues. J. Exp. Pathol., 5:143–153.
- Pavelic, Z.P., Pavelic, K., Carter, C.P., Pavelic, L.J. (1992). Heterogeneity of c-myc expression in histologically similar infiltrating ductal carcinomas of the breast. J. Cancer Res. Clin. Oncol., 118:16–22.
- Hussain, H., Tan, B.H., Mya, K.Y., Liu, Y., He, C.B., Davis, T.P. (2009). Synthesis, micelle formation, and bulk properties of poly(ethylene glycol)-b-poly(pentafluorostyrene)-g-polyhedral oligomeric silsesquioxane amphiphilic hybrid copolymers. J of Polym Sci Part A:48(1):152–163.
- Zhang, J., Jiang W., Zhao, X., Wang, Y. (2007). Preparation and caracterization of polymeric micelles from poly (D, L-lactide) and methoxypolyethyelene glycol block copolymers as potential drug carriers. Tsinghua Science and Technology, 12(4):493–496.
- Zhao X., Pan F., Zhang, Z., Grant, C., Yinghua, M., Armes S.P., Yiqing T., Lewis, A.L., Waigh, T., Lu J.R. (2007). Nanostructure of polyplexes formed between cationic dblock copolymer and antisense oligodeoxynucleotide and its influence on cell transfection efficiency. Biomacromolecules, 8(11):3493–3502.
- Thomas, M., Lu, J.J., Ge, G., Zhang, C., Chen, J., Klibanov, A.M. (2005). Full deacylation of polyethyleneimine dramatically boosts its gene delivery efficiency and specificity to mouse lung. Proc. Natl. Acad. Sci. U.S.A. 102:5679–5684.
- Van-de-Wetering, P., Moret, E.E., Schuurmans-Nieuwenbroek, N.M., Van-Steenbergen, M.J., Hennink, W.E. (1999). Structure-activity relationships of water-soluble cationic methacrylate/methacrylamidepolymers for non-viral gene delivery. Bioconjugate Chem, 10:589–597.
- Gebhart, C.L., Sriadibhatla, S., Vinogradov, S., Lemieux, P., Alkahov, V., Kabanov, V.A. (2002). Design and formulation of polyplexes based on pluronic-polyethyleneeimine conjugates for gene transfer. Bioconjugate Chem, 13:937–944.
- Ochietti, B., Guerin, N., Vinogradov, S.V., St-Pierre, Y., Lemieux, P., Kabanov, A.V., Alakhov, V.Y. (2002). Altered organ accumulation of oligonucleotides using polyethyleneimine grafted with poly(ethyleneoxide) or Pluronic as carriers. J. Drug Targeting, 2:113–121.