Abstract
Abstract: Fibers comprised of reconstituted type I collagen were prepared by a gravity filament forming process and crosslinked with 0.1% glutaraldehyde. These fibers have a crosslinking index of about 90% (89.89 ± 1.82%) with higher denature temperature (74.43 ± 0.08°C) as compared to that without glutaraldehyde treatment (52.1 ± 0.17°C). The ultimate tensile strength of the collagen fibers increases from 99.4 ± 12.9 to 174.4 ± 9.0 MPa after glutaraldehyde-crosslinking.
L929 fibroblast cells were seeded and cultured using these newly developed collagen fibers. The fibroblast cells proliferated well and covered all surface areas of the collagen fiber. These collagen fibers have a great potential for application in 3-D tissue engineering.
INTRODUCTION
Type I collagen, a major structural protein in vertebrates, is comprised of two α1(I) and one α2(I) chains intertwining into a unique triple-helix structure. Collagen exists in the extracellular matrix (ECM) of connective tissue as cross-striated fibrils that are formed by the self-assembly of collagen molecules [Citation1]. Because it provides an excellent support for cell growth, type I collagen has been utilized for culturing cells and tissue repair.
Many different forms of collagen products, including gel, membrane, sponge, and conduit, have been fabricated for clinical applications [Citation2–4]. Collagens in the form of filament or fiber are of great use since they can be applied as is or as the starting material for constructing various medical devices.
In the past, collagen fibers were prepared from mature, corium-derived insoluble type I collagen for repairing the injured tendon and ligament [Citation5–7]. These collagen fibers are derived from the acid-soluble fraction of Type I collagen thus containing non-helical telopeptides, which often evoke immune response when implanted in the host. Type I collagen after the removal of telopeptide is non-immunogenic, or possesses a negligibly low level of immunogenicity [Citation8]. In this study, we utilized atello- collagen from calf skin as the source for preparing collagen fibers. A wet gravity filament-forming process was used with 70% ethanol as a coagulation bath at a low shear rate. The properties and applications as cell carrier of these collagen fibers are reported here.
MATERIALS AND METHODS
Materials
Gultaradehyde, dimethyl sulfoxide (DMSO), and most chemical reagents were purchased from Merck (Whitehouse Station, NJ, USA). Ascorbic acid, β-glycerophosphate, and 3-[4, 5-dimethylthiazol-2-yl]-2, 5-diphenyltetrazolium bromide (MTT) were obtained from Sigma (St. Louis, MO, USA). Fetal calf serum and DMEM (Dulbecco’s Modified Eagle Medium) were purchased from GIBCO BRLR (Glasgow, UK).
Preparation of Collagen Fibers
Type I collagen was prepared from the bovine skin with 0.5 M acetic acid and pepsin treatment as described in a previous paper [Citation9]. The purified collagen was stored in 0.5 M acetic acid at 4°C, and dialyzed against 0.02 M PBS (150 mM NaCl; 20mM disodium hydrogen phosphate, pH 7.4) before use.
Collagen fibers were fabricated using the gravity coagulation technique similar to the one used to fabricate poly(caprolactone) developed by Williamson et al. [Citation10] and the apparatus used in this study is depicted graphically in .
The neutral collagen solution (6 mg/ml) was extruded through a syringe needle (0.38 mm inner diameter) by a syringe pump (Model KDS-101, KDS Scientific, USA) at a flow injection rate of 30 μl/min. The collagen solution was extruded into a coagulation solution of phosphate buffer containing 70% ethanol at 37°C. After 15 min, the fibers were subjected to a gradient hydration process by decreasing ethanol concentration from 70% to 10%. Finally, the fibers were immersed in PBS at 37°C for 24 hr for collagen reconstitution.
The resulting fibers were subsequently crosslinked by 0.1% glutaraldehyde in PBS 37°C for 24 hr and then air-dried in laminar flow and stored in an air-tight bottle at −20°C. Each step of this collagen fiber preparation was conducted under a sterile environment.
Characterizations of Collagen Fibers
Scanning Electron Microscope (SEM). Collagen-fiber was crosslinked with 2.5% glutaraldehyde in 0.1M sodium cacodylate (pH 7.4), dehydrated using a series of ethanol solutions, and critical-point-dried with carbon dioxide. The dried sample was coated with a thin layer of gold (∼30 nm) using an Agar Sputter Coater and examined under a JEOL-5300 scanning electron microscope.
Determinations of Swelling Index, Crosslinking Index, and Mechanical Properties. Samples of dry collagen fibers were weighed (W1) and then placed separately in a 25-mL beaker containing 10 ml PBS buffer pH 7.0 at 37°C. After 24 hr, the samples were removed from the immersing solution, wiped with filter paper, and reweighed (W2). The swelling index was calculated as follows: Swelling index = (W2-W1)/W1.
The crosslinking index, defined as the percentage of free amino groups reacted with crosslinking agent, was determined by ninhydrin assay. The sample was first lyophilized for 24 hr and then weighed. Subsequently, the lyophilized collagen fibers were heated with a ninhydrin solution for 20 min and the light absorbance of the solution was recorded with a spectrophometer (Model DU640B; Beckman-Coulter Inc., California, USA) using glycine as standard.
The denaturization temperature of collagen fiber was measured in a Perkin-Elmer differential scanning calorimeter (Model DSC 7; Norwalk, Connecticut). This technique was commonly used in studying the thermal transition of collagenous tissue [Citation11].
For the determination of the mechanical property, a single fiber was cut into a length of 5 cm, and the ultimate tensile stress (UTS), elastic modulus, and elongation at fracture were determined using a universal testing machine (Model H1KS; Housfield, UK) with the strain rate of 10 mm/min. The fiber diameter was measured under an optical microscope and calibrated with an eyepiece graticule.
The shrinkage of collagen fiber during crosslinking was measured and calculated using the method described by Lee et al. [Citation12]. The percent shrinkage, S/L0, can be expressed as equation (1):
where S is the shrinkage length of the crosslinked fiber, L0 is the length of fiber without glutaraldehyde-crosslinking, εfixed and εfresh are the observed strains at fracture of the fibers with and without glutaraldehyde-crosslinking, respectively.
Cell Culturing with Collagen Fibers
To demonstrate the application using these collagen fibers as cell carrier, L929 fibroblasts (2×104 cells/cm3-well) were seeded on collagen fibers (10 mg) and cultured in a growth medium of DMEM/10% fetal calf serum in a humidified air of 10% CO2 incubator at 37°C. On the 1st, 2nd, 4th, and 7th day after cultivation, the cell-containing fibers were transferred to Eppendorff tubes and incubated with 0.5mg/ml MTT at 37°C for 4h. After centrifugation, the supernatant was collected and DMSO was added with vigorous shaking to dissolve the purple Formazan crystals formed. The absorbance was measured with a spectrophotometer at a wavelength of 570nm.
Confocol Microscope Observations
A confocal microscope was used to take the image of cells and collagen fibers in a 3-D network. For nuclear staining, the cell-containing collagen fibers were incubated with PI (10 ug/ml), which forms a stable, highly fluorescent complex with double-stranded DNA. The staining was conducted by immersing the cell-containing fibers in 2.5% glutaraldehyde-PBS, and then incubated with PI for 5 min. For cytoskeleton staining, the cell-containing collagen fibers were incubated with Rhodamine-Phalloidin (to stain F-actin) for 15 min, then washed with PBS and examined under a confocal microscope.
Statistical Analysis
Statistical analysis for determination of differences in the measured properties between groups was performed using one-way analysis of variance and determination of confidence intervals with the Statistical Analysis System program, Version 6.08; SAS Institute Inc., Cary, NC) and presented as mean ± SD.
RESULTS AND DISCUSSION
Morphology of Collagen Fibers
Ethanol is a poor solvent for collagen, and thus can be used as the coagulation solution for fabricating collagen fibers. The neutral collagen solution coagulated and formed fibers () of 279.4 ± 3.3 μm in diameter. When extruded into the ethanol (70% v/v), these collagen fibers initially exhibit wavy surface after being retrieved from the coagulation bath (), and then turned into fibrillar structure after the gradient hydration process (). shows the gross appearance of the dry collagen fibers crosslinked with glutaraldehyde. The collagen fibers exhibit yellowish color due to the formation of Schiff base. The average diameter of the wet glutaraldehyde-crosslinked fibers is 292.2 ± 4.5 μm.
Figure 2. (a) Photograph of the glutaraldehyde-crosslinked collagen fibers. The scanning electron micrographs of (b) without glutaraldehyde-crosslinked collagen fibers; (c) the surface of the fiber was coagulated in 70% (v/v) ethanol for 15 min; and (d) the surface of the fiber was prepared after the gradient hydration process.

Properties of Collagen Fibers
The amount of the free amino group of collagen fibers decreased significantly after being treated with glutaraldehyde as indicated by the crosslinking index (89.89 ± 1.82) (P < 0.05). It is believed that both inter- and intra-molecular crosslinks occur and stabilize the collagen fibrils. As determined from DSC measurement, the denaturization temperature of the crosslinked fiber is about 74.43 ± 0.08°C, much higher (P < 0.05) than the collagen fibers (52.1 ± 0.17°C) before crosslinking. As shown in the stress-strain curves (), all collagen fibers were typical elastic-plastic materials. Each fiber shows a low strain modulus (E1) and high strain modulus (E2) [Citation7]. The mechanical properties of the collagen fibers are summarized in . The UTS (ultimate tensile strength) and E1 of the glutaraldehyde-crosslinked fibers were significantly higher than those without glutaraldehyde treatment (P < 0.05). Thus the mechanical strength of fibers can be effectively enhanced by increasing the crosslinking of collagen. As compared with rat tail tendons (RTT) [Citation7], the glutaraldehyde-treated collagen fibers have lower UTS, but higher E1 and elongation at failure (P < 0.05).
Figure 3. Stress-strain curves of collagen fibers with and without glutaraldehyde-treatment. Each thread is typical elastic-plastic material and has a low strain modulus (E1) and high strain modulus (E2) in stress-strain curve.
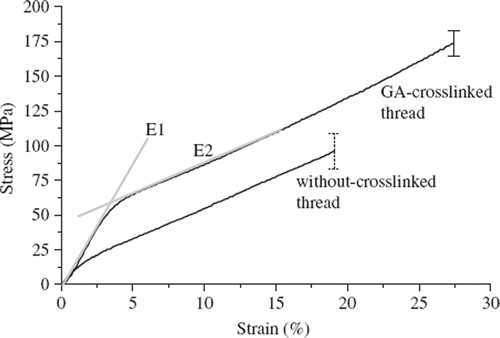
Table 1. Mechanical properties of the collagen fibers before and after glutaraldehyde treatment
The elongation of the collagen fibers at fracture increased significantly after glutaraldehyde-crosslinking, mean strains at fracture of 19.1% without glutaraldehyde-treatment, and 27.9% for the glutaraldehyde-crosslinked fibers. As estimated from equation (1), the collagen fiber has shrunk by 6.88 ± 0.35% during crosslinking, probably due to contraction of collagen fibrils by inter- and intra-molecular crosslinks. It is likely that inter-molecular crosslinks may bring the adjacent collagen fibrils closer, while intra-molecular crosslinks may contract the collagen fibrils. However, these results may only apply for the fiber being crosslinked without constraint in a crosslinking bath.
Accompanying the increase of crosslinking, the average swelling index of the collagen fibers decreased from 38.63 ± 1.96 to 23.96 ± 2.36 after glutaraldehyde treatment. Because of the crosslinks, the collagen fibers have a more compact structure and thus the extent of swelling decreases.
Cells Cultured on Collagen Fibers
The application of collagen fibers is demonstrated here by culturing L929 fibroblast cells using the fibers as substrate. The viability of the cultured cells, as shown by MTT assay that measures the activities of mitochondria dehydrogenase of living cells, increased significantly after 7 days of cultivation (). Improved cell proliferation on the collagen fibers may be explained by the presence of the cell adhesion sites such as RGD sequence, a binding motif for cell adhesion via the prominent α1β1 and α1β2 integrin receptors. In addition, a larger area provided by the surface of collagen fibers (), as compared to that of TCPS (tissue culture polystyrene) culture flask, is also advantageous for the fibers to attain a higher cell density.
Figure 4. The cell activities of L929 fibroblasts which were cultivated on the 12-well culture plate (12-TCPS, ▪) and glutaraldehyde-crosslinked fibers (▪) are normalized to the control group. The control group is defined as the absorbance of the MTT assay of the fibroblasts cultivated on the 12-TCPS in the 1st day. The initial cell numbers are 2×104 cells/well. Each data point resulted from 5 samples.

Cell Morphology Observed under Microscopes
The cultured fibroblast cells were examined after staining with PI, which intercalates with double-stranded DNA, thus locating the cell nuclei. The results of PI-stain () showed that fibroblast cells grew and spread well over the surface of the collagen fiber. The morphology of the cells attached to collagen fibers was examined by the fluorescent image of Rhodamine-labeled Phalloidin, which binds specifically to F-actin microfilaments of the adhered cells. As shown in , the Rhodamine-Phalloidin staining revealed the presence of stress fibers of the well spread L929 cells on collagen fiber. Together, these results indicate that the glutaraldehyde-crosslinked collagen fibers serve as an excellent support for cell attachment and growth.
Figure 5. Confocol microscopic images of L929 fibroblast cells grown on the glutaraldehyde-crosslinked collagen fibers (7 days after seeding), staining with (a) PI for nucleus and (b) Rhodamine-Phalloidin for actin filaments. The initial cells’ density was adjusted to 2×104 and seeded on the 10 mg GA-crosslinked collagen fibers.
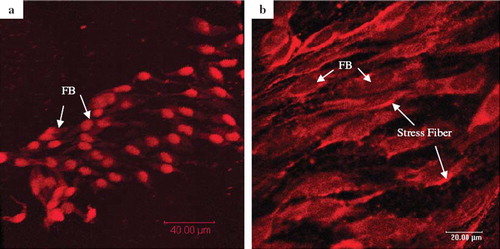
On day 7 after cell seeding, the morphology of L929 fibroblasts located on the surface of the glutaraldehyde-crosslinked collagen fibers show a flattened, spread, and spindle-shaped appearance typical of a cellular monolayer (). The cells have formed a confluent layer that conforms closely to the surface of collagen fiber. The use of glutaraldehyde for cross-linking biological tissue implants has a number of undesirable side-effects reported, including cytotoxicity and induction of calcification [Citation13]. In this study, the collagen fibers were thoroughly washed during preparation and the cells a ppeared to maintain their good abilities in proliferation. The close interaction of cells with collagen fibrils on the glutaraldehyde-crosslinked collagen fibers is obvious. This demonstrates that the newly developed collagen fibers provide an excellent environment for cell growth and have potential application for construction of scaffolds for tissue engineering.
Figure 6. SEM photographs of L929 fibroblasts grown and attached on the glutaraldehyde-crosslinked fibers (7 days after seeding) under 1000× magnification. The cell exhibits a typical spindle-shape on the surface of the fiber. The initial cells’ density is about 2×104 and seeded on the 10 mg glutaraldehyde-crosslinked collagen fibers.
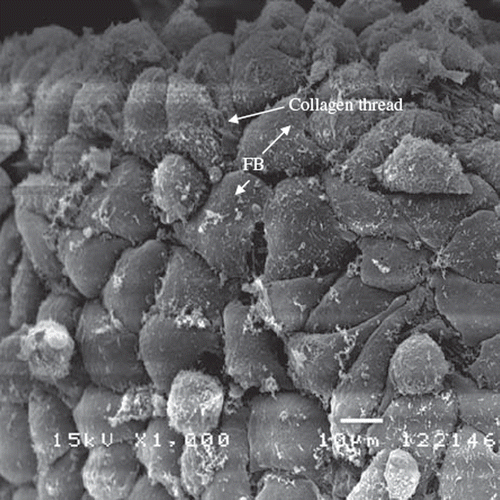
CONCLUSION
We have used a gravity filament method for preparing the reconstituted collagen fibers from triple-helices collagen molecules. The glutaraldehyde-crosslinked collagen fibers have much better mechanical strength than uncross-liked ones and are suitable for cell growth. They can be used as a single fiber or as the starting material to construct 3-D scaffolds for engineering tissue regeneration.
Declaration of interest: The authors report no conflicts of interest. The authors alone are responsible for the content and writing of the paper.
REFERENCES
- Kadler, K.E., Holmes, D.F., Trotter, J., Chapman, J.A. (1996). Collagen fibril formation. Biochem. J. 316:1–11.
- Lee, C.H., Singla, A., Lee, Y. (2001). Biomedical applications of collagen. Int. J. Pharm. 221:1–22.
- Hsu, F.Y., Tsai, S.W., Wang, F.F., Wang, Y.J. (2000). The collagen-containing alginate/poly(L-lysine)/alginate microcapsules. Artif. Cells Blood Substit. Immobil. Biotechnol. 28:147–54.
- Hsu, F.Y., Chueh, S.C., Wang, Y.J. (1999). Microspheres of hydroxyapatite/reconstituted collagen as supports for osteoblast cell growth. Biomaterials 20:1931–6.
- Gentleman, E., Lay, A.N., Dickerson, D.A., Nauma, E.A., Livesay, G.A., Dee, K.C. (2003). Mechanical characterization of collagen fibers and scaffolds for tissue engineering. Biomaterials 24:3805–13.
- Koob, T.J., Hernandez, D.J. (2002). Material properties of polymerized NDGA-collagen composite fibers: development of biologically based tendon constructs. Biomaterials 23:203–12.
- Kato, Y.P., Christiansen, D.L., Hahn, R.A., Shieh, S.J., Goldstein, J.D., Sliver, F.H. (1989). Mechanical properties of collagen fibres. Biomaterials 10:38–42.
- Lynn, A.K., Yannas, I.V., Bonfield, W. (2004). Antigenicity and immunogenicity of collagen. J Biomed. Mater. Res. B, Appl. Biomater. 71:343–54.
- Len, C.H., Wang, F.F., Wang, Y.J. (2003). Osteogenic enrichment of bone-marrow stromal cells with the use of flow chamber and type I collagen-coated surface. J. Biomed. Mater. Res. 66A:38–46.
- Williamson, M.R., Adams, E.F., Coombes, A.G.A. (2006). Gravity spun polycaprolactone fibres for soft tissue engineering: Interaction with fibroblasts and myoblasts in cell culture. Biomaterials 27:1019–26.
- McClain, P.E., Wiley, E.R. (1972). Differential scanning calorimeter studies of the thermal transitions of collagen: Implications on structure and stability. J. Biol. Chem. 247:692–7.
- Lee, J.M., Pereira, C.A., Abdulla, D., Naimark, W.A., Crawford, I. (1995). A multi-sample denaturation temperature tester for collagenous biomaterials. Med. Eng. Phys. 17:115–21.
- McPherson, J.M., Sawamura, S., Armstrong, R. (1986). An examination of the biologic response to injectable, glutaraldehyde cross-linked collagen implants. J. Biomed. Mater. Res. 20:93–107.