Abstract
Abstract: A glucose oxidase-based biosensor was developed for the determination of α-amylase activity. The determination method is based on monitoring the decrease in dissolved oxygen concentration related to the starch concentration, for which starch gives a reaction with α-amylase. Optimization parameters, including glucose oxidase amount, gelatin amount, and glutaraldehyde percentage for cross-linking, were investigated. The effects of pH, buffer system, and temperature on the biosensor system were also investigated. The biosensor had a linear relation to α-amylase activity and good measurement correlation between 0.66 and 9.83 U/ml. In sample analysis studies, α-amylase activity in baker's yeast was determined by the biosensor.
INTRODUCTION
With the discovery of the first starch degrading enzyme by Kirchhoff in 1811, the history of amylases began. Various digestive amylases and malt amylases were reported after that revelation [Citation1]. α- and β-amylases are the two types of starch digestive enzymes. They were first classified by Ohlsson in 1930 according to their reaction products. α-amylases (1,4-α-d-glucan-glucanhydrolase, EC. 3.2.1.1) are extensively distributed extracellular secretary enzymes and they are ubiquitous proteins that play an important role in the carbohydrate metabolism of microorganisms, animals, and plants [Citation2]. α-amylase catalyses the random cleavage of α-1,4 glycosidic linkages in starch and produces glucose, dextrins, and limit dextrins [Citation3,Citation4,Citation5]. Many genes involved in the synthesis of extracellular α-amylase have been identified in Bacillus suptilis. The structural gene for the α-amylase enzyme is amyE [Citation6]. X-ray studies have shown that all α-amylases consist of three domains. These domains are called A, B, and C [Citation7]. Between domains A and B, α-amylases have at least one calcium ion, which is essential for the stability and activating the enzyme [Citation7, Citation8]. The amylase family enzymes have potential application in several industrial processes because of their high thermo-stability. They are used especially in food, textiles, and paper industries [Citation9]. α-amylases are used in a routine manner in bread making for flour standardization and as anti-staling agents [Citation10].
There are many detection methods for detecting α-amylase activity such as competitive substrate, and product inhibition-based α-amylase activity analysis methods [Citation11, Citation12, Citation13], decrease in starch–iodine color intensity [Citation14, Citation15], dinitrosalicylic acid method [Citation16], sensitive agar diffusion method [Citation17], degradation of color-complexed substrate [Citation18, Citation19], and decrease in viscosity of the starch suspension [Citation20]. These methods have exhibited successful α-amylase activity determination; however, some of them may not form into a corporation of routine analysis and may not have as low a detection limit as a biosensor has. As a result, development of a biosensor for the activity determination of α-amylase is very important.
In this study, a different method for α-amylase activity determination is presented. Activity determination was carried out by monitoring the oxygen consumption level. The rate of consumption of oxygen was proportional to the level of glucose produced from the hydrolysis of starch by α-amylase, and was directly related to the activity of the enzyme.
EXPERIMENTAL
Materials and Methods
Glucose oxidase (50 U/mg, of Aspergillus niger origin), potassium dihydrogene phosphate (KH2PO4), citric acid (C6H8O7), acetic acid (CH3CO2H), sodium hydroxide (NaOH), sodium chloride (NaCl), bovine skin gelatin, sodium dodecyl sulfate (CH3(CH2)11OSO3Na), glycine and glutaraldehyde (25%) were purchased from Sigma (St. Louis, USA). α-amylase (65.55 U/mg, of Bacillus suptilis origin) was purchased from NOVO INDUSTRI AS (Copenhagen, Denmark) and starch (from potatoes) was purchased from Fluka (Switzerland). All reagents used were of analytical grades. All solutions were prepared with double distilled water just before their use.
Apparatus
YSI 57 A model oxygen meter, YSI 5700 series Clark-type oxygen electrode (YSI Co. Inc., Yellow Springs, OH, USA) and highly sensitive Teflon membrane for oxygen was used. A water bath was used for preparation of bioactive material (Nüve, BM 302, UK). All the measurements were carried out at constant temperature using a thermostat (Haake JF, Germany). Magnetic stirrers (IKA-Combimag, RCO) and pH meters with electrode (WTW, pH538, Germany) were used for preparing buffer solutions. The temperature was maintained constant in the reaction cell by circulating water at appropriate temperatures around the cell compartment during the experiment.
Methods
Preparation of the Electrode. First of all, the Clark electrode was filled inside with KCl solution without containing a bubble. Then, the surface of the Clark electrode was covered with a standard Teflon membrane using an o-ring. 0.5% sodiumdodecylsulphate in phosphate buffer (50 mM, pH 7.5) was pipetted on an oxygen-specific Teflon membrane surface to reduce the tension on the membrane surface, so that gelatin was spread over the surface easily.
Preparation of the Biosensor. Five milligrams of glucose oxidase and five milligrams of gelatin were dissolved in 240 μl, pH 7.5, 50 mM phosphate buffer. The mixture of glucose oxidase, gelatin, and sodium phosphate buffer was dispersed over the dissolved oxygen probe membrane surface and was allowed to dry at 4°C for 30 minutes. After that process, in order to cross-link the enzyme and gelatin with glutaraldehyde solution the bioactive-carrying electrode surface was immersed into 2.5 % (v/v) glutaraldehyde solution for 5 minutes. Thus, chemical covalent bonds (Schiff bases) were formed between the enzyme, gelatin, and glutaraldehyde molecules to provide an immobilization on the surface of the dissolved oxygen probe. Finally, excess of glutaraldehyde was eliminated by double distilled water.
Measurement Procedure. The principle of the reaction is based on the determination of oxygen consumption by the activity of glucose oxidase that is immobilized on the electrode surface. There is an intermediate surface between the bioactive layer and the Teflon membrane of the dissolved oxygen probe. In the reaction, α-amylase hydrolyzes the starch molecules, which present in the phosphate buffer (50 mM, pH 6.5). When α-amylase hydrolyzes starch molecules, dissolved oxygen concentration in the intermediate surface decreases relative to the substrate concentration added into the phosphate buffer medium. The principle of the reaction is depicted in .
50 ml of the phosphate buffer (50 mM, pH 6.5), including starch molecules, were transferred into the reaction cell. Glucose oxidase-based biosensor was placed into the thermostatic reaction cell and the magnetic stirrer was fixed at a constant speed (500 circle/minute). All of the measurements were performed at the same speed. The mixture was incubated for five minutes at the “red-line position” of the oxygenmeter for polarization of the glucose oxidase-based electrode. Dissolved oxygen diffused through the highly sensitive Teflon membrane. That diffusion resulted in the stabilization of the dissolved oxygen between the working buffer and dissolved oxygen probe. Then, the dissolved oxygen concentration was recorded. At this time, α-amylase enzyme solution was injected into the reaction cell; hence it decreased the dissolved oxygen concentration by hydrolyzing starch molecules. In the experiments, different volumes of α-amylase standart solution between 50 to 1500 μl were added into the reaction chamber. After a few minutes, dissolved oxygen concentration reached to a constant level and was recorded again. Measurements were carried out by computing the differences between the dissolved oxygen concentrations (ΔDO), which was recorded before and after adding α-amylase enzyme solutions. In conclusion, the developed biosensor was based on the determination of these changes in the dissolved oxygen concentrations related to the α-amylase activity. These differences were directly proportional to α-amylase activity in a certain amount. After completing the measurements, the glucose oxidase-based biosensor was washed with distilled water and stored at 4°C.
RESULTS AND DISCUSSION
Optimization of the Biosensor
In this part of the study, optimum working conditions were investigated to obtain the maximum performance from the glucose oxidase biosensor, which was developed for the determination of α-amylase activity. Therefore different amounts of glucose oxidase, gelatin, and glutaraldehyde were used for preparation of the bioactive layer material.
Optimization of Glucose Oxidase Amount
The biosensor was prepared by fixing the amounts of gelatin and glutaraldehyde at the varying amounts of glucose oxidase for the purpose of monitoring the effect of the glucose oxidase activity on the GOD-O2 biosensor. Four different biosensors containing 1.2 mg, 2.5 mg, 5.0 mg, and 10.0 milligrams of glucose oxidase were constructed in this study. Glucose oxidase amounts, linear ranges, and R2 values of the different biosensors are given in . As can be seen in , the slopes of the biosensors prepared with different glucose oxidase amounts were very close to each other. The biosensor prepared with 5 mg glucose oxidase had the widest linear range for α-amylase activity obtained by this biosensor. Therefore, 5.0 mg glucose oxidase was detected as the optimum amount.
Table 1. The effects of glucose oxidase amount, gelatin amount, and glutaraldehyde percentage on the biosensor response
Optimization of Gelatin Amount
Three biosensors were prepared in order to determine the effect of gelatin on biosensor response. 2.5 mg, 5.0 mg, and 7.5 mg gelatin were immobilized on the electrode surface, respectively. Optimum gelatin amount was determined as 5.0 mg by obtaining α-amylase standard curves. The relation between gelatin amount and biosensor response is shown in . 7.5 mg gelatin caused a decrease in current response of the biosensor because the amount of gelatin was too thick for the substrate to pass through the gelatin layer and to interact with glucose oxidase. Because of the insufficient tightness of the enzyme immobilization, 2.5 mg gelatin also decreased the biosensor response. Maximum biosensor response was obtained using 5.0 mg of gelatin. Consequently, 5.0 mg of gelatin was chosen as the optimum gelatin amount.
Optimization of Glutaraldehyde Percentage
Biosensors were treated with 1.25 %, 2.5 %, and 5.0 % glutaraldehyde solution prepared in phosphate buffer (50 mM, pH 7.0) for the immobilization. After the cross-linking of glutaraldehyde molecules, experiments were carried out to obtain standard curves for α-amylase activity by using the prepared biosensors. Experiments showed that the best response of biosensor was observed at 2.5% glutaraldehyde percentage. The relationship between the biosensor response and glutaraldehyde percentage is indicated in . Optimum glutaraldehyde percentage was determined as 2.5%. The biosensors that were prepared with 1.25% glutaraldehyde were inadequate for immobilization of the enzyme on the electrode surface. Nevertheless, preparing the electrode with 5.0% glutaraldehyde decreased the response of the biosensor because it led to difficulties in reaching to the active site of the enzyme.
In consequence of these three optimization studies, the final biosensor was composed as follows: The amounts of glucose oxidase and gelatin were kept constant at 5 mg and glutaraldehyde percentage was 2.5%. This procedure was selected to be used for preparation of the biosensor in all of the further measurements.
Optimization of pH
The effect of pH on the biosensor response was investigated. Thus, three different buffer systems were used to determine optimum biosensor response. Optimum pH of the enzyme-based biosensor for α-amylase activity is shown in .
Figure 1. The effect of pH on the biosensor response. Working conditions: citrate buffer (♦)(pH 4.5, 5.0, 5.5, 6.0, 6.5, 50 mM); phosphate buffer (■) (pH 6.5, 7.0, 7.5, 8.0, 50 mM) and glycine buffer (▲) (pH 8.5, 9.0, 9.5, 10.0, 10.5, 50 mM), T = 35°C; 10 mg/ml starch solution and 5.244 U/ml standard solution of α-amylase were used.
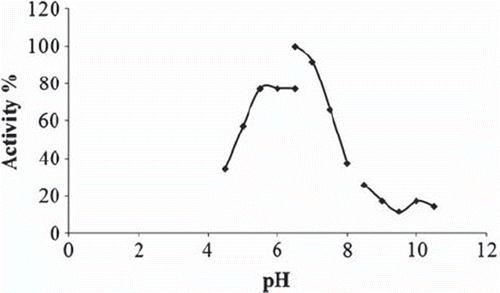
The results demonstrated that the best biosensor signal was obtained at phosphate buffer pH 6.5.
Determination of Appropriate Buffer System
In this part of the study, effects of three different buffer systems on the enzyme-based biosensor response were examined. To this end, citrate, phosphate, and glycine buffer systems were used at pH 6.5. The buffer system from which the optimum biosensor response was obtained was determined by creating standard curves of each buffer system for α-amylase activity. shows the effects of the buffer systems on the biosensor response.
Figure 2. The effect of buffer system on the biosensor response.Working conditions: T = 35°C; 10 mg/ml starch solution and 5.244 U/ml standard solution of α-amylase were used.
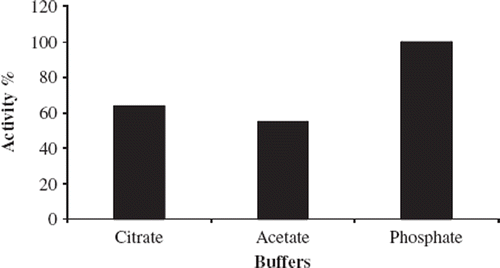
Low-level oxygen corruption was monitored in citrate and acetate buffers in comparison to the phosphate buffer. In the phosphate buffer system, maximum enzymatic activity was determined.
Optimization of Temperature
The effect of temperature on the biosensor response can change because of the immobilized enzyme on the electrode surface. Since enzyme activities can be affected by the temperature, it is very important to detect the optimum temperature to obtain the best biosensor response. For this aim, experiments were carried out between 25 and 60°C (). The biosensor worked best at 50 and 55°C. These values are very high as a working temperature and give rise to denaturation of the enzyme, which results in the shortening of biosensor's life. For this reason 40°C was accepted as a working temperature for the further experiments.
Analytical Characteristics of the Biosensor
Linear Range of the Biosensor. In this study, the results obtained for the determination of detection limits for α-amylase activity are given in .
Figure 4. Linear range of the biosensor. Working conditions: Phosphate buffer; pH 6.5, 50 mM; T = 40°C; 10 mg/ml starch solution and α-amylase activity between 0.66-9.83 U/ml.
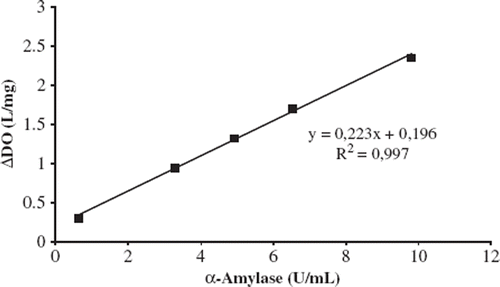
Under the optimum experimental conditions a linear calibration curve was obtained at concentrations between 0.66 and 9.83 U/ml α-amylase with (y = 0.2235x + 0.1969) and R2 = 0.9972. At higher activities than 9.83 U/ml, a diversion from linearity occurred. This is probably because of the limited solubility of oxygen in a water medium, since oxygen acts as a co-substrate for oxygen-consuming enzymes. In consequence, after a particular substrate concentration, oxygen acts as a limiting factor on the reaction.
Repeatability. Repeatability studies were carried out by using the same biosensor 10 times repeatedly. After 10 replicate determinations, repeatability results for 5.244 U/ml α-amylase activities were found, as shown in .
Table 2. Repeatability of the biosensor.
After these experiments, it can be concluded that the biosensor presents a correctly desirable characteristic of repeatability.
Storage Stability. To understand how the performance of the biosensor based on glucose oxidase for α-amylase activity alters with time under the effect of environmental factors, storage stability experiments were performed.
The biosensor was stored at +4°C for two weeks. The measurements were done at the first and seventh day and at the end of the storage period. At the seventh day of the storage period, the response of the biosensor was decreased to 93.4%. After 14 days, no activity was detected. These results revealed that storage stability of the biosensor was not as good as expected.
Sample Analysis. In this section of the study, α-amylase activity of a baker's yeast was detected by using the biosensor. 2.5 grams of baker's yeast was dissolved in 2.5 ml double distilled water. For the disintegration of yeast cells, the mixture was treated with an ultrasonicator. A reference method was used to compare the results [Citation12]. Averages of five measurement results obtained from the trials are shown in . By comparing the results of the experiments, it can be concluded that the biosensor can be applied for activity determination of α-amylase.
Table 3. α-amylase activity determination in baker's yeast using the biosensor and using a spectrophotometric reference method.
CONCLUSION
In this work we demonstrate a novel biosensor based on glucose oxidase for activity determination of α-amylase. We present a different viewpoint of α-amylase activity detection by immobilizing glucose oxidase on Clark electrode to monitor the consumption of the oxygen produced by the hydrolysis of starch. Starch is hydrolyzed by α-amylase in the reaction medium. If we compare this activity determination method with the others, it can be concluded that the biosensor exhibits an alternative one. The biosensor has a rapid response. Activity could be measured with high accuracy and reliability. Ease of construction of the biosensor and simple measurement method are the advantages for the biosensor. In addition, it does not need any expensive equipment, material, or laboratory conditions. Moreover, the biosensor is simple and rapid to use. The quality of industrial products of α-amylase-catalyzed reactions is related to the enzyme activity. For example, this enzyme directly determines the quality of bread in the baking industry because α-amylases degrade starch and produce small dextrins for the yeast to act. In conclusion, biosensor development for amylase activity determination is important.
Declaration of interest: The authors report no conflicts of interest. The authors alone are responsible for the content and writing of the paper.
REFERENCES
- Gupta, R., Gigras, P., Mohapatra, H., Goswami, V. K., Chauhan, B. (2003). Microbial α-amylases: A biotechnological perspective. Process Biochemistry, 38: 1599–1616.
- Payan, F. (2004). Structural basis for the inhibition of mammalian and insect α-amylases by plant protein inhibitors. Biochimica et Biophysica Acta, 1696: 171–180.
- Strobl, S., Maskos, K., Wiegand, G., Huber, R., Gomis-Rüth, F. X., Glockshuber, R. (1998). A novel strategy for inhibition of α-amylases: Yellow meal worm α-amylase in complex with the Ragi bifunctional inhibitor at 2.5 Å resolution. Structure, 6: 911–921.
- Tripathi, P., Leggio, L. L., Mansfeld, J., Ulbrich-Hofmann, R., Kayastha, A. M. (2007). α-amylase from mung beans (Vigna radiata) – Correlation of biochemical properties and tertiary structure by homology modeling. Phytochemistry, 68: 1623–1631.
- Kumar, R. S. S., Singh, S. A., Rao, A. G. A. (2009). Conformational stability of α-amylase from malted sorghum (Sorghum bicolor): Reversible unfolding by denaturants. Biochimie, 91: 548–557.
- Mielenz, J. R. (1983). Bacillus stearothermophilus contains a plasmid-borne gene for α-amylase. Proc. Natl. Acad. Sci. USA, 80: 5975–5979.
- Nielsen, J. E., Borchert, T. V. (2000). Protein engineering of bacterial α-amylases. Biochimica et Biophysica Acta, 1543: 253–274.
- Kumari, A., Rosenkranz, T., Kayastha, A. M., Fitter, J. (2010). The effect of calcium binding on the unfolding barrier: A kinetic study on homologous α-amylases. Biophysical Chemistry, 151: 54–60.
- Francis, F., Sabu, A., Nampoothiri, K. M., Ramachandran, S., Ghosh, S., Szakacs, G., Pandey, A. (2003). Use of response surface methodology for optimizing process parameters for the production of α-amylase by Aspergillus oryzae. Biochemical Engineering Journal, 15: 107–115.
- Goesaert, H., Slade, L., Levine, H., Delcour, J. A. (2009). Amylases and bread firming – an integrated view. Journal of Cereal Science, 50: 345–352.
- Yamaguchi, M., Wakasugi, J., Sakakima, J. (2008). Competitive and product inhibition-based α-amylase activity analysis method. Clinical Biochemistry, 41: 325–330.
- Priya, S., Kaur, N., Gupta, A. K. (2010). Purification, characterization and inhibition studies of α-amylase of Rhyzopertha dominica. Pesticide Biochemistry and Physiology, 98: 231–237.
- Baks, T., Janssen, A. E. M., Boom, R. M. (2006). The effect of carbohydrates on α-amylase activity measurements. Enzyme and Microbial Technology, 39: 114–119.
- Saxena, R. K., Dutt, K., Agarwal, L., Nayyar, P. (2007). A highly thermostable and alkaline amylase from a Bacillus sp. PN5. Bioresource Technology, 98: 260–265.
- El-Naggar, M. M. A., Farag, M. G. (2010). Physical and biological treatments of polyethylene–rice starch plastic films. Journal of Hazardous Materials, 176: 878–883.
- Miller, G. L. (1959). Use of dinitrosalicylic acid reagent for determination of reducing sugar. Analytical Chemistry, 31: 426–428.
- Farias, D. F., Carvalho, A. F. U., Oliveira, C. C., Sousa, N. M., Rocha-Bezerrra, L. C. B., Ferreira, P. M. P., Lima, G. P. G., Hissa, D. C. (2010). Alternative method for quantification of alfa-amylase activity. Braz. J. Biol. 70: 405–407.
- Wong, D. W. S., Batt, S. B., Robertson, G. H. (2000). Microassay for rapid screening of α-amylase activity. J. Agric. Food Chem., 48: 4540–4543.
- Sheehan, H., McCleary, B. V. (1988). A new procedure for the measurement of fungal and bacterial α-amylase. Biotechnology Techniques, 2: 289–292.
- Perten, H. (1984). A modified falling-number method suitable for measuring both cereal and fungal alpha-amylase activity. Cereal Chem., 61: 108–111.