Abstract
In the present study, the stabilizing effect of dextrans as additives on the denaturation and inactivation of glucose oxidase (GOD) was investigated. Three different molecular weighted dextrans (Mw 17.5, 75, 188 kD) were used with different concentrations. Dramatically increased enzyme activities were measured after one hour of incubation of enzyme with additives between 25–40°C in water bath. Highest activity value was measured with 75 kDa molecular weighted dextran (in concentration 30% w/v) at pH 5. Dextran as an additive supplied a long shelf-life to the enzyme at 4°C. In the presence of the 75 kDa dextran, the enzyme was more stable and its activity was increased 2.7-fold at 30°C. In addition, dextran protected GOD against inactivation by a n-heptane/aqueous buffer-stirred system.
Introduction
Glucose oxidase (GOD) is involved in a wide variety of applications in the food and fermentation industry, and as an analytical tool in biosensors for medical applications and environmental monitoring (Gouda et al. 1997). GOD (β-D-glucose: oxygen-oxidoreductase, EC 1.1.3.4) from Aspergillus niger is a flavoprotein that catalyzes the oxidation of β-D-glucose to D-glucono- δ -lactone and hydrogen peroxide, using molecular oxygen as the electron acceptor. This enzyme is a dimer of two identical subunits with a molecular weight of 160,000. The dimer contains two disulfide bonds, two free sulfhydryl groups (Tsuge et al. Citation1975), and two FAD molecules tightly bound to the enzyme (Swoboda Citation1969). The dimer has a high degree of localization of negative charges on the enzyme surface and dimer interface (Ahmad et al. Citation2001). The flavin cofactors are responsible for the oxidation-reduction properties of the enzyme (Jones et al. Citation1982, Gouda et al. Citation2003, Gulla et al. Citation2004).
GOD obtained from natural sources is not stable for the long term under the conditions used in such applications. The stabilization of GOD against destructive factors improves its characteristics and broadens its applicability (Atikatoglu et al. Citation2010).
Several methods have been applied to enhance the operational stability of enzymes, such as chemical modification, the use of stabilizing additives, immobilization, and genetic engineering. Use of stabilizing additives increases the resistance of enzyme molecules against the denaturating effect of working conditions and also induces long shelf-life. These additives exert stabilizing effects by inducing hydration of proteins (O'Fagain Citation2003, Janecek Citation1993, Hassani et al. Citation2006).
Thermal inactivation of enzymes occur mainly because of thiol group oxidation, denaturation, and aggregation (Gouda et al. Citation2003, Gulla et al. Citation2004, Ye and Combes Citation1989). Denaturation and aggregation of enzymes during thermal inactivation can be minimized by using additives like salts, polyols, polyelectrolytes, and by immobilization through tertiary structure (Gouda et al Citation2003, Gulla et al. Citation2004). The effectiveness of stabilization by incorporation of additives would depend on the nature of enzymes, their hydrophobic/hydrophilic character, and the degree of interaction with the additives (Gouda et al. Citation2003).
Studies reported that aggregation is the main cause for inactivation of glucose oxidase (GOD), which can be prevented by modifying the microenvironment of the enzyme molecule. Thermal denaturation of GOD is mainly due to the destabilization of hydrophobic interactions and breakage of hydrogen bonds, van der Waals forces, and ionic interactions which lead to conformational changes in the tertiary structure of the enzyme and render it to an inactive form (Tsuge et al. Citation1975).
Previously, we have studied the enhancement of thermal resistance of GOD by covalent conjugation with aldehyde derivative of dextran (Atikatoglu et al. Citation2010). In this current work, we investigated the protective effect of dextrans on GOD denaturation and inactivation. Thus, three different, molecular-weighted dextrans were used as protective additives with different concentrations. Enzyme activities were determined after one hour of incubation and with no incubation in different buffer systems and at working temperatures.
Methods
Reagents
Glucose oxidase from Aspergillus niger (Mw = 186 kDa) (FL.49180), Sephadex G-50 column material (FL.84946), and o-dianisidine (FL.33430) were purchased from Fluka. D-(+)-glucose (G7528) and dextrans from Leuconostoc mesenteroides with different molecular weights (MWMALLS = 17.5, 75, 188 kDa; SI.D4626, SI.D3759 and SI.D4876, respectively) were obtained from Sigma Chemical Co. (St. Louis, MO). All other chemicals used were at analytical grade.
Purification of GOD
Protein content of the commercial GOD was determined as 90 % (w/w). HPLC (Viscotek GPCmax VE2001 GPC Solvent/sample module) chromatograms of the commercial GOD also confirmed the presence of impurities. Therefore, purchased GOD was purified by Gel Filtration Chromatography using Sephadex G-50, according to the previously described method (Atkatoglu et al. 2010).
Determination of enzyme activity
In order to adjust the temperatures, test tubes containing 780 μl (0.01 M) phosphate buffer were incubated in stirred water baths at working temperatures before the reaction initiation. Subsequently, 50 μl glucose (%25 w/v), 25 μl o-dianisidin (10 mM), 15 μl HRP (0.005 mg/ml), and finally as the initiator of this reaction 30 μl GOD solution (0.0025 mg/ml) were added, respectively, to the buffer-containing test tubes. After 10 minutes of incubation, 100 μl H2SO4 (2 M) was added to the reaction media to stop the reaction and absorbance values at 400 nm were recorded (Atikatoglu et al. Citation2010, Park et al. Citation2000, Dai et al. Citation2002). One enzymatic unit causes the oxidation of 1 μmol of o-dianisidine per minute (εo-dianisidine: 17 500 M−1cm−1 at 400 nm) (Atikatoglu et al. Citation2010).
Thermal stability of GOD was evaluated with the activities determined according to the procedure described above after 60 min of incubation at different working temperatures in the absence and presence of different molecular weighted dextrans (Mw 188 kDa, 75 kDa, and 17.5 kDa) as additives. Meanwhile, the stability of enzyme against pH factor was also determined by repeating the procedure given above for each pH value in the absence and presence of 75 kDa dextran. Enzyme/additive preparations, which were in buffer solutions at desired pH values, were incubated at 30 °C for 1 h. Furthermore, shelf-life of native and dextran-treated GOD were evaluated at 4 and 30 °C. All values are expressed as the mean ± standard deviation (SD).
Determination of thermal stability
Dextran-containing buffers were prepared by adding various amounts (10, 20, and 30% w/v) of different molar weighted dextrans (17.5 kDa, 75 kDa, 188, 500 kDa) to phosphate (for pH 6, 7, and 8), acetate (for pH 4 and 5) or carbonate (for pH 9) buffer. Purified commercial GOD was added to the dextran-containing buffer. Reaction mixtures were incubated for an hour in water baths at different temperatures (25, 30 40, 50, 60, 70, 80, 90 °C) and enzyme activity was assayed.
Enzyme inactivation assays in stirred n-heptane/aqueous buffer systems
50 ml of 0.05 M sodium acetate buffer-containing native GOD or 75 kDa dextran (30 % w/v) treated GOD and 10 ml of n-heptane were mechanically stirred at 1,200 rpm. In order to prevent enzyme inactivation by the organic solvent molecules, which were dissolved in the aqueous phase, the experiment was carried out at 25 °C and pH 5.
Samples of the aqueous phase were withdrawn at different times, and the residual activity was measured as previously described. All measurements represent averages of at least three experiments. In all cases, the experimental error was not higher than 5%.
Determination of storage stabilities of native and dextran-treated GOD
Sample preparations containing 0.0025 mg/ml native GOD or GOD treated with water solution of 75 kDa (30% w/v) dextran were incubated in an acetate buffer (pH 5.0) at 4°C. Enzyme activity was assayed at proper time intervals in order to determine the remaining activity of the enzyme. All the experiments were carried out in triplicate and the results represent mean values with less than 2% of error.
Scanning Probe Microscope (SPM) analysis
Surface morphologies of native and dextran-treated GOD were observed with scanning probe microscope SPM 9600 (Shimadzu, Japan) in dynamic mode using a Silicon cantilever. The force constant and resonance frequency of the cantilever used were 42 N/m and 320 kHz, respectively. Scanning rate and resolution were set to 1 Hz and 256 × 256 pixels, respectively. Samples were prepared by depositing a drop of purified commercial GOD or dextran-treated GOD on a fre?hly cleaved mica surface. The sample surface was rinsed with ultra pure water and dried at room temperature after 5 minutes of incubation. Each SPM image was obtained using an area of 0.5 μm × 0.5 μm.
Results and discussion
The denaturation temperature indicates the destruction of secondary structure, but the destruction of secondary structure is not the only reason for enzyme deactivation. Destroyed hydrate layer, enzyme aggregation, and some other unfavorable factors could also lead to a notable decrease and even a total loss of enzyme activity, even when temperature was much lower than its denaturation temperature (Noel and Combes Citation2003).
The effect of different molecular weighted dextrans as additives on the thermal stability of GOD was studied (). The enzyme/additive preparations were incubated in 50 mM acetate buffer pH 5.0, for 1 h at corresponding temperatures, and the activity was assayed. It can be seen from these activity results () that 75 kDa dextran-treated GOD showed highest activity at all temperatures (25–80 °C). When we compare these activity results with our previous studies (Atikatoglu et al. Citation2010, Altikatoglu and Basaran Citation2011), it is obvious that the molecular weight of the dextran used plays a critical role in the thermal stabilization of the enzyme molecules.
Figure 1. The effect of different molecular-weighted dextrans as additives on the thermal stability of GOD.
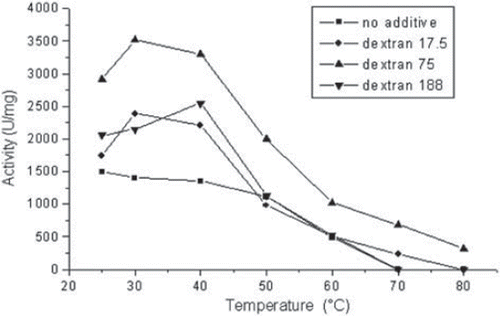
It can be seen from . that the activity of dextran-treated GOD increased with the increasing dextran concentration. When dextran concentration of the 75 kDa dextran-treated GOD was 30% (w/v), the activity values were increased at all temperatures (ca. 2-fold higher activity than pure enzyme). But when dextran of dextran-treated GOD was lower, the activity increase could be seen only between temperatures of 25–40 °C in comparison with the native enzyme. Furthermore, at 70 °C, where native enzyme lost its activity, dextran-treated GOD (20, 30% w/v) showed activity. Polyols have been successfully used as additives for improving the thermal stability of enzymes in aqueous and non-aqueous media. The novel use of polyol-containing polymers as thermoprotectant additives for enzymes was described previously (Iyer and Ananthanarayan Citation2008, Ladero et al. Citation2006, Sadana and Henley Citation1987).
Enzymes will generally undergo reversible or irreversible conformational changes under extreme pH conditions. The conformational changes of GOD under different pH conditions with or without dextran as additive (75 kDa dextran in concentrations 10, 20, and 30% w/v) were studied (). The conformation stability in α-helixes and β-sheets of enzyme mainly depends on the hydrogen bonds formed between the hydrogen atoms and the oxygen atoms on neighboring amino acid residues (Federici et al. Citation2009). These hydrogen bonds as well as the net charge of the enzyme surface would be greatly influenced by pH variation (Boscolo et al. Citation2009, Li et al. Citation2010). As shown in , the maximum in the pH profile of enzyme activity - pH 4.0 shifted toward pH - 5.0. The highest activity values were obtained in the presence of 30% (w/v) 75 kDa dextran at all pHs. The use of additives at different pHs probably modifies the charge of protein surface and consequently changes the isoelectric constant of the surroundings (Altikatoglu and Basaran Citation2011, Costa et al. Citation2002). It could be deduced from the above results that electrostatic interactions played an important role in maintaining pH stability of GOD.
Figure 3. pH profile of GOD activity in the presence of additives (in the presence of 10, 20, and 30% (w/v) 75 kDa dextran). Experimental conditions were 30 °C and incubation for 1 h. Each data point represents the average value of three independent experiments with error bars indicated.
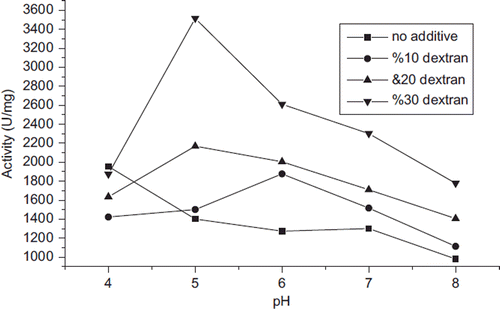
These results are in agreement with the SPM images (). shows the SPM images of the surface morphologies of the GOD in the presence and absence of additive (75 kDa dextran). These SPM images clearly show the variations in the morphology of the GOD before and after dextran addition. Although all specimens prepared in the same conditions, the differences observed in SPM images indicate? the interaction between GOD and dextran, hence enzyme microenvironment modification by additives was revealed.
The storage stabilities of GOD with and without dextran are compared in . The activity of native GOD decreased by 72% after 10 days of storage and continued to decrease thereafter. On the thirty-eighth day of storage, only 4% of its initial activity remained. Storage stability of GOD could be significantly improved by adding dextran (75 kDa, in 30% w/v concentration), so that the activity was stable during the first 20 days, and 44% of the activity could be retained in 90 days.
Table I. Effect of dextran on the storage stability of GOD at 4°C. Each data point represents the average value of three independent experiments.
Dextran (Mw 75 kDa) treated GOD exerted a stabilizing effect against the n-heptane/aqueous buffer-stirred system. As shown in a and b, dextran-treated GOD preserved 100% of residual activity after 15 and 10 h while the native enzyme was almost fully inactive within the first 4 and 3 h. Interfacial inactivation must be the major driving force for the gradual loss of activity of the unmodified enzyme, while the covering of the enzyme with the polymer proved to be very protective (Betancor et al. Citation2004). By protecting the non-covalent interactions by means of additives, the denaturation of protein can be minimized (Gulla et al. Citation2004). These values are in agreement with the values reported in the case of other enzymes with dextran aldehyde (Betancor et al. Citation2004, Altikatoglu and Celebi Citation2011).
Figure 5. Inactivation course of native (<) and dextran in concentration 30% (w/v) (Mw 75 kDa) treated GOD (<) in presence of liquid-organic interface (a); under strong agitation (1200 rpm) (b). Experimental conditions were 25 °C and pH 5.0. Each data point represents the average value of three independent experiments.
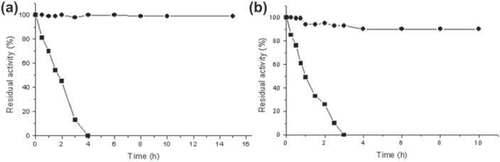
When we compare these results with our previous study (Altikatoglu and Basaran Citation2011), it is obvious that, depending on the enzyme used, on the concentration and on the molecular weight of additive employed, the protective effect of the same polyol may vary considerably.
Conclusions
The presence of polysaccharides and polyols significantly influenced the enzyme activity and stability (Noel and Combes Citation2003, Li et al. Citation2010). The experiments revealed that the additive provided much better long-term storage stability at pH 5.0. The application of additive improved the pH and temperature profile of enzyme activity. The results show that there are interactions between dextrans and the enzyme, leading to an increase or a reduction in the activity. The possibility of stabilizing GOD against thermal, pH, or aqueous-solvent and air-liquid interfaces inactivation in the presence of dextrans would be of great interest for biotechnological applications, as many industrial processes require elevated temperature, pH, or organic solvents.
Acknowledgments
The authors wish to dedicate this study to the beloved professor Huriye Kuzu.
Declaration of interest
The authors report no conflicts of interest. The authors alone are responsible for the content and writing of the paper.
References
- Ahmad A, Akhtar MS, Bhakuni V. 2001. Monovalent cation-induced conformational change in glucose oxidase leading to stabilization of the enzyme. Biochemistry 40:1945–1955.
- Altikatoglu M, Basaran Y. 2011. Additive effect of dextrans on the stability of horseradish peroxidase. Protein J 30:84–90.
- Altikatoglu M, Celebi M. 2011. Enhanced stability and decolorization of Coomassie Brilliant Blue R-250 by dextran aldehyde-modified horseradish peroxidase. Artif Cells, Blood Substitutes, Biotechnol 39:185–190.
- Atikatoglu M, Basaran Y, Arioz C, Ogan A, Kuzu H. 2010. Glucose oxidase-dextran conjugates with enhanced stabilities against temperature and pH. Appl Biochem Biotechnol 160:2187-2197.
- Betancor L, Fuentes M, Dellamora-Ortiz G, Lopez-Gallego F, Hidalgo A, Alonso-Morales N, Mateo C, Guisan JM, Fernandez-Lafuente R. 2005. Dextran aldehyde coating of glucose oxidase immobilized on magnetic nanoparticles prevents its inactivation by gas bubbles. J Mol Catal B: Enzym 32:97–101.
- Betancor L, Lόpez-Gallego F, Hidalgo A, Alonso-Morales N, Fuentes M, Fernández-Lafuente R, Guisán JM. 2004. Prevention of interfacial inactivation of enzymes by coating the enzyme surface with dextran-aldehyde. J Biotechnol 110:201–207.
- Boscolo B, Leal SS, Salgueiro CA, Ghibaudi EM, Gomes CM. 2009. The prominent conformational plasticity of lactoperoxidase: A chemical and pH stability analysis. Biochim Biophys Acta Proteins Proteomics 1794:1041–1048.
- Costa SA, Tzanov T, Carneiro AF, Paar A, Gubitz GM, Cavaco-Paulo A. 2002. Studies of stabilization of native catalase using additives. Enzyme MicrobTechnol 30:387–391.
- Dai GL, Li JR, Jiang L. 2002. Difference in enzyme activity and conformation of glucose oxidase before and after purification. Colloids Surf B 24:171–176.
- Federici L, Masulli M, Gianni S, Ilio CD, Allocati N. 2009. A conserved hydrogen-bond network stabilizes the structure of Beta class glutathione S-transferases. Biochem Biophys Res Commun 382:525–529.
- Gouda MD, Singh SA, Rao AGA, Thakur MS, Karanth NG. 2003. Thermal inactivation of glucose oxidase-mechanism and stabilization using additives. J Biol Chem 278:24324–24333.
- Gulla KC, Gouda MD, Thakur MS, Karanth NG. 2004. Enhancement of stability of immobilized glucose oxidase by modification of free thiols generated by reducing disulfide bonds and using additives. Biosensors and Bioelectronics 19:621–625.
- Hassani L, Ranjbar B, Khajeh K, Naderi-Manesh H, Naderi-Manesh M, Sadeghi M. 2006. Horseradish peroxidase thermostabilization: The combinatorial effects of the surface modification and the polyols. Enzyme Microb Technol 38:118–125.
- Iyer PV, Ananthanarayan L. 2008. Enzyme stability and stabilization— aqueous and non-aqueous environment. Process Biochem 43:1019–1032.
- Janecek S. 1993. Strategies for obtaining stable enzymes. Process Biochem 28:435–445.
- Jones MN, Manley P, Wilkinson A. 1982. The dissociation of glucose-oxidase by sodium normal-dodecyl sulfate. Biochem J 203:285–291.
- Ladero M, Santos A, Garcıa-Ochoa F. 2006. Kinetic modelling of the thermal inactivation of an industrial β-galactosidase from Kluyveromyces fragilis. Enzyme Microb Technol 38:1–9.
- Li J, Jiang Z, Wua H, Lianga Y, Zhanga Y, Liua J. 2010. Enzyme–polysaccharide interaction and its influence on enzyme activity and stability. Carbohydr Polym 82:160–166.
- Noel M, Combes D. 2003. Rhizomucor miehei lipase: Differential scanning calorimetry and pressure/temperature stability studies in presence of soluble additives. Enzyme Microb Technol 33:299–308.
- O'Fagain C. 2003. Enzyme stabilization-recent experimental progress. Enzyme Microb Technol 33:137–149.
- Park EH, Shin YM, Lim YY, Kwon TH, Kim DH, Yang MS. 2000. Expression of glucose oxidase by using recombinant yeast. J Biotechnol 81:35–44.
- Sadana A, Henley JP. 1987. Single-step unimolecular non-1st-order enzyme deactivation kinetics. Biotechnol Bioeng 30:717–723.
- Swoboda BEP. 1969. The relationship between molecular conformation and the binding of flavin-adenine dinucleotide in glucose oxidase. Biochim Biophys Acta 175:365–379.
- Tsuge H, Natsuakai O, Ohashi K. 1975. Purification, properties and molecular features of glucose oxidase from Aspergillus niger. J Biochem 78:835–843.
- Ye WN, Combes D. 1989. The relationship between the glucose oxidase subunit structure and its thermostability. Biochim Biophys Acta 999:86–93.