Abstract
In this investigation, a new design based on a PANDA ring resonator as an optical trapping tool for tangle protein, molecular motor storage, and delivery is proposed. The optical vortices are generated and the trapping mechanism is controlled in the same way as the conventional optical tweezers. The trapping force is produced by a combination of the gradient field and scattering photons. The required molecular volume is trapped and moved dynamically within the molecular network. The tangle protein and molecular motor can be transported and delivered to the required destinations for Alzheimer's diagnosis by molecular buffer and bus network.
Introduction
Alzheimer's disease (AD) is the most common form of dementia. This neurodegenerative disease is a growing problem in the aging population. It is predicted that AD victims will be tripled by 2050 and healthcare cost will grow beyond one trillion USD. There is a need to slow down the onset of AD. The loss of neural cells associated with AD is believed to be due to the accumulation of beta amyloidal plaques (Selkoe Citation2001, Cumming 2004). Beta amyloid and tau
protein waste deposits in the brain are characteristic features of AD. The toxic effect of tau protein is eliminated when the tau gene is switched off. In AD, the access phosphate group causes the tau protein to malfunction, forming clumps known as neurofibrilary tangles. These result in the neuron transport breakdown leading to the death of the neuron and sub-napses (Astrid et al. Citation2011). Tangles inside neurons (Mayeux 2010) and genes (Selkoe Citation2001) are caused by the abnormal axons in the neuronal networks. A study of axonal transport began with the observation of nerve cell bodies (Morgan Citation2004), where the axons consist of many microtubules. Each microtubule is structured as a hollow cylindrical tube with an external diameter of 25 nm and a wall thickness of 4 nm. The cross-section of the microtubule consists of 13 proto-filaments with the fastest axonal transportation at a rate of 5 μm per second (Karp Citation2010). The main function of the microtubule is to deliver cellular components to the action site. The two major components of the transport machinery are the “engines” and molecular motors.
Optical technologies are improving biological and biochemical screening and detection systems. The optical trapping technique has been used to control kinesin movement on a microtubule surface (Schnitzer and Block Citation1997, Block et al. Citation2003, Block Citation2007). Erickson laboratory researchers (Yang and Erickson Citation2010, Chung et al. Citation2009, Krishnan et al. Citation2009, Chung and Erickson Citation2009, Schmidt et al. Citation2007) have reported on the study of micro-nanofluidics and combination of optics, based on advancing flows, delivery, and leads to implantable devices for living organs (Segev et al. Citation2011, Bugge and Palmers Citation2010, Chen et al. Citation2011). New advance optical strategies using light to drive and halt neuronal activities with molecular specificity have been investigated. The optical methods that have been developed to date encompass a broad array of strategies, including photorelease of caged neurotransmitters, engineered light-gated receptors, naturally light-sensitive ion channels pumps (Szobota and Isacoff Citation2010) and artificial neural networks (Suwanpayak et al. Citation2011b). Recently, the use of an optical trapping tool to transport microscopic volume within an add/drop multiplexer has been reported (Piyatamrong et al. Citation2010, Cai and Poon Citation2010). The transporter used is known as an optical tweezer, which acts as a powerful tool for manipulation of micrometer-sized particles. To date, the useful static tweezers are well recognized and realized. Moreover, the use of dynamic tweezers is now also realized in practical work (Ashkin et al. Citation1987, Egashira et al. Citation1998, Kachnyski et al. 2003). Schulz et al. (Citation2007) have shown the possibility of transferring trapped atoms between two optical potentials. In principle, an optical tweezer uses the forces exerted by intensity gradients in the strongly focused beams of light to trap and move the microscopic volumes. In application, the field intensity can be adjusted and tuned to the desired gradient field. The scattering force then generates the suitable trapping force. Hence, the appropriate force can be configured as the transmitter/receiver for performing a long-distance microscopic transportation.
In this paper, the dynamic optical tweezers/vortices are generated using the optical pulses propagating within anadd/drop optical multiplexer incorporating two nanoring resonators (PANDA ring resonator) (Tasakorn et al. Citation2010). By using the proposed system, the tangle protein and molecular motor can be trapped and transported/received into the optical waveguide by optical tweezers. By utilizing the reasonable optical pulse input power, the dynamic tweezers can be controlled and stored within the system before reaching the desired destinations via the molecular buffer and bus network. In this application, the neuron network can be connected by the link protein for AD and recovery.
Principle and method
In theory, the trapping forces are exerted by the gradient fields in the strongly focused beams to trap and move the microscopic volumes of matters. The optical forces are customarily defined by the relationship (Ou-Yand and Wei Citation2010).
Here Q is a dimensionless efficiency, nm is the refractive index of the suspending medium, c is the speed of light, and P is the incident laser power, measured at the specimen. Q represents the fraction of power utilized to exert force. For a plane wave that is incident on a perfectly absorbing particle, Q is equal to 1. To achieve stable trapping, the radiation pressure must create a stable, three-dimensional equilibrium. Because biological specimens are usually contained in aqueous medium, the dependence of F on nm can rarely be exploited to achieve higher trapping forces. Increase in the laser power is possible, but only over a limited range due to the possibility of optical damage. Q itself is therefore the key factor of trapping force and depends upon the NA (numerical aperture), laser wavelength, light polarization state, laser mode structure, relative index of refraction, and geometry of the particle.
In the Rayleigh regime, trapping forces decompose naturally into two components. Since the electromagnetic field is uniform across the dielectric, the particles can be treated as induced point dipoles. The scattering force is given by (Svoboda and Block Citation1994)
where
Here σ is the scattering cross-section of a Rayleigh sphere with radius r. S is the time averaged Poynting vector, n is the index of refraction of the particle, m = n/nm is the relative index, and k = 2πnm /λ is the wave number of the light. The scattering force is proportional to the energy flux and points along the direction of propagation of the incident light. The gradient field (Fgrad) is the Lorentz force acting on the dipole induced by the light field. It is given by (Svoboda and Block Citation1994)
where
α is the polarizability of the particle. The gradient force is proportional and parallel to the gradient in energy density (for m > 1). The large gradient force is formed by the large depth of the laser beam. The stable trapping requires that the gradient force in the direction, which is against the direction of incident light (dark soliton valley) and it is greater than the scattering force. By increasing the NA, the focal spot size is decreased and the gradient strength is increased (Suwanpayak et al. Citation2011a). The whole phenomenon occurs within a tiny nanoscale system such as the nanoring resonator.
Optical trapping is one of the most powerful single-molecule technique with vast applications in the study of molecular motors (Ashkin Citation1997) and medicine. Hosokawa et al. (Hosokawa et al. Citation2011) demonstrated the optical trapping of synaptic vesicles in a hippocampal neuron and found that the intracellular synaptic vesicles can be trapped at the focal spot within the laser irradiation time because the vesicles form clusters in a neuron. These clusters can effectively trap at the focal spot because of high polarizability.
Alzheimer's diagnosis
Dynein and kinesin proteins transport cellular chemical cargos in microtubules. In neurons, microtubules are attached to tau proteins. These tau proteins are bound to the microtubules’ surface. The dynein and kinesin protein react differently when expose to tau proteins. The tau protein plays a crucial role in AD. These proteins regulate the transport of nutrients, signaling molecules, and waste proteins in neurons. The tau protein controls dynein and kinesin movement. Neuro degeneration of neurons may be due to mutation in dynein and kinesin resulting in AD. Thus, the transport, movement, and distribution of tau protein along the microtubules are important in the diagnosis of AD (Dixit et al. Citation2008).
In this paper, we propose the use of the optical tweezer for tangle protein trapping and transportation in the neuronal cell (motor protein, tau protein, beta amyloid) causing AD. Amyloid and tau protein are both implicated in memory impairment, mild cognitive impairment (MCI), and early Alzheimer's disease. However, their interaction is still unknown (Shipton et al. Citation2011). In operation, the optical tweezer can trap, transport, and store within the PANDA ring resonator (Piyatamrong et al. Citation2010), incorporating a wavelength router in the same drug delivery network system (Suwanpayak et al. 2011). We use the theory of optical trapping and transportation technique (Svoboda et al. Citation1993, Steven et al. Citation1990, Kolomeisky et al. Citation2007) to trap kinesins and plaques (tangle protein), the kinesin motor molecules are spheres and directly moved to the microtubules where they could be activated by ATP (Ashkin Citation1997).
The proposed Alzheimer's diagnosis system is shown in y using the molecular buffer and bus network, the required trapped volumes can be transported within the network to the destinations. The volumes can be filtered via the add/ drop filter before reaching the desired destinations. The throughput port (Et1) of the add/drop filter is connected to the microtubule. The effective area of the waveguide is 2.01 μm2 (r = 800 nm) and the external diameter of the microtubule is 25 nm (Karp Citation2010). The size of the axon diameter from birth is 1 μm. Through childhood (7 years) it becomes 12 μm and into adulthood 24 μm (Paus and Toro Citation2009). shows the schematic of the waveguide and microtubule position in the axon. The proposed design system is used to trap kinesin (Bechtluft et al. Citation2007) and transporting/storing the tangle protein via the molecular buffer and bus network. The light waveguide is inserted into the axon to trap the kinesin and to trap/store/receive the tangle protein. The ungrouping form of these proteins causes the Alzehimer's disease of aging (Ou-Yand and Wei Citation2010). The optical tweezer can induced mechanical unfolding and refolding of a single protein molecule in the absence and the presence of molecular chaperones (Xia et al. Citation2005). Furthermore, the optical trapping technique can unfold the poly-protein (Ying et al. Citation2010) with noninvasive neuronal of the adult brain (Choi et al. Citation2004).
Figure 1. Schematic diagram of an Alzheimer's diagnosis system using a molecular buffer and bus network. The coupling coefficients are given as κ0 = 0.5, κ1 = 0.35, κ2 = 0.1, and κ3 = 0.35, respectively. The ring radii are Rad = 1.5 and 1 µm, RR = 0.7 µm and 0.7 µm, and RL = 0.7 and 0.7 µm, respectively.
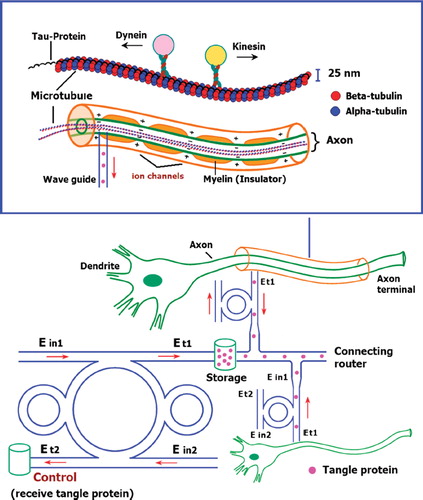
In simulation, a bright soliton of center wavelength at 1.50 µm, peak power 2W, and a pulse 35 fs is input into the system via the input port, with the coupling coefficients given as κ0 = 0.5, κ1 = 0.35, κ2 = 0.1, and κ3 = 0.35, respectively. The ring radii are Rad = 1.5 and 1 µm, RR = 0.7 µm and 0.7 µm, and RL = 0.7 and 0.7 µm, respectively. To date, the evidence of a practical device with the radius of 0.8 µm (approx.) has been reported by Prabhu et al. (Citation2010); Aeff is 2.01 μm2 (r = 800 nm). In this case, the dynamic tweezers (gradient fields) can be in the form of bright solitons, Gaussian pulses and dark solitons, which can be used to trap the required tangle protein, causing the Alzheimer's disease. In four different center wavelengths of tweezers are generated; the dynamical movements are (a) |E1|2, (b) |E2|2, (c) |E3|2, (d) |E4|2, (e) through port, and (f) drop port signals. In this case all microscopic volumes are received by the drop port. In practice, the ring resonator radii can be easily controlled as compared to coupling constants. The important aspect of this result is that the tunable tweezers can be obtained by tuning (controlling) the add (control) port input signal, thus allowing the required number of single protein (tau-protein/ beta myeloid, plaque) to be obtained and seen at the drop/through ports. Otherwise, they propagate within a PANDA ring before collapsing/decaying into the waveguide.
Figure 3. Result of the dynamic tweezers within the buffer with different: (a) wavelengths; (b) the optical potential well of throughput and norm of drop port; and (c) coupling constants, where Rad = 1.5 µm, RR = RL = 700 nm.
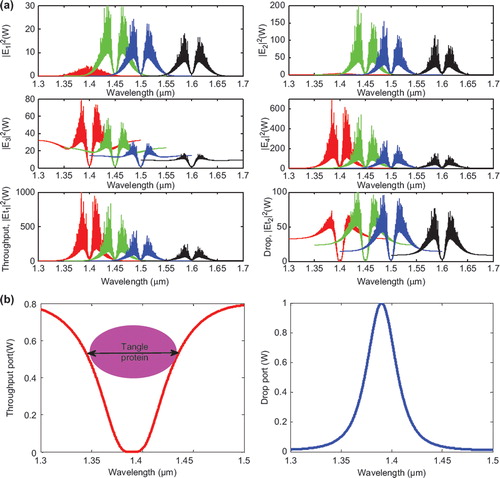
The optical tweezers are generated within the PANDA ring as shown in . The bright soliton is used as the control port signal to obtain the tunable results. The output optical tweezers of the through and drop ports with different coupling constants are shown in . (a) shows the different wavelength, (b) represents the potential well of throughput port and norm of drop port, and (c) represents the coupling constant. In application, the trapped microscopic volumes (molecules) can be transported into the wavelength router via the through port with different coupling coefficients and wavelength (). The advantage of the proposed system is that the transmitter and receiver can be fabricated on-chip and alternatively operated by a single device.
Conclusion
We have demonstrated that the tangle protein and molecular motor can be trapped and transported/received into the optical waveguide by optical tweezers (Hosokawa et al. Citation2011, Mthunzi et al. Citation2010). By utilizing the specific optical pulse input power, the dynamic tweezers can be generated to transport, store, and deliver the molecule volumes to their final destination via the molecular buffer and bus network. The tweezer amplification is also available by using the nanoring resonators and modulated signals via the control port. In conclusion, the use of the molecular buffer and bus network for long-distance protein trapping and transportation can be realized using the proposed system. The trapped proteins and tangles can then be transported via the wavelength router and bus network to the required (connecting) targets. In the near future, AD diagnosis using micro-ring resonators can be extended into quantum dot lasers to be employed as high-precision scalpels with which tangle proteins and molecular structures may be parted in control manners. In addition certain cell organelles, either intra-cellular or extra-cellular, may be activated using the proposed system, which would open up unforeseen opportunities in the molecular surgery of AD.
Acknowledgements
We would like to thank the Institute of Advanced Photonics Science, Nanotechnology Research Alliance, Universiti Teknologi Malaysia (UTM), and King Mongkut's Institute of Technology (KMITL), Thailand, for providing the research facilities. This research work has been supported by UTM's Tier 1 Research Grant, MOHE SLAI Fellowship, MyBrain15 Fellowship, and the Ministry of Higher Education (MOHE) research grant.
Declaration of interest
The authors report no conflicts of interest. The authors alone are repsonsible for the content and writing of the paper
References
- Ashkin A, Dziedzic JM, Yamane T. 1987. Optical trapping and manipulation of single cells using infrared laser beams. Nature 330:769–771.
- Ashkin A. 1997. Optical trapping and manipulation of neutral particles using lasers. Proc Natl Acad Sci 94:4853 4858.
- Astrid S, Van der Jeugd A, Fang Z, Tariq A, Alschun D, Petrova O, Drexler D, Zhou L, Rune G, Mandelkow E, D'Hooge R, Alzheimer C, Mandelkow EM. 2011. Tau-induced defects in synaptics plasticity, learning and memory are reversible in trans-genic moce after switching off the tau mutant. Journal of Neuroscience 31(7): 2511–2525.
- Bechtluft P, Van Leeuwen RGH, Tyreman M, . 2007. Direct observation of chaperone-Induced changes in a protein folding pathway. Science 318:1458–1461.
- Block SM, Asbury CL, Shaevitz JW, . 2003. Probing the kinesin reaction cycle with a 2D optical force clamp. PNAS 100(5):2351–2356.
- Block SM. 2007. Kinesin motor mechanics: Binding, stepping, tracking, gating, and limping. Biophys 92:2986–2995.
- Bugge M, Palmers G. 2010. Implantable device for utiliztion of the hydraulic energy of the heart. US RE41,394 E. 2010 June 22.
- Cai H, Poon A. 2010. Optical manipulation and transport of microparticle on silicon nitride microring resonator -based add-drop devices. Opt Lett 35:2855–2857.
- Chen SY, Hu SH, Liu DM, . 2011. Drug delivery nanodevice, its preparation method and use. US 2011/0014296 A1. 2011 Jan 20.
- Choi JH, Wolf M, Toronov V, . 2004. Noninvasive determination of the optical properties of adult brain: Near-infrared spectroscopy approach. J Biomed Opt 9(1):221–229.
- Chung AJ, Erickson D. 2009. Engineering insect flight metabolics using immature stage implanted microfluidics. Lab Chip 9:669–676.
- Chung AJ, Huh YS, Erickson D. 2009. A robust electrochemically driven microwell drug delivery system for controlled vasopressin release. Biomed Microdevices 11:861–867.
- Cummings JL. 2004. Alzheimer's disease. N Engl J Med 351:56–67.
- Dixit R, Ross JL, Goldman YE, . 2008. Differential regulation of dynein and kinesin motor proteins by tau. Science 319(5866):1086–1089.
- Egashira K, Terasaki A, Kondow T. 1998. Photon-trap spectroscopy applied to molecules adsorbed on a solid surface: Probing with a standing wave versus a propagating wave. App Opt 80:5113–5115.
- Hosokawa C, Kudoh SN, Kiyohara A, . 2011. Optical trapping of synaptic vesicles in neurons. Appl Phys Lett 98:163705.
- Karp G. 2010. Cell and molecular biology, 6th ed. New York: John Wiley & Sons, Inc.
- Kachynski AV, Kuzmin AN, Pudavar HE, . 2003. Measurement of optical trapping forces by use of the two-photon-excited fluorescence of microspheres. Opt Lett 28:2288–2290.
- Kolomeisky AB, Michael E. Fisher ME. 2007. Molecular motors: A theorist's perspective. Annu Rev Phys Chem 58:675–95.
- Krishnan M, Tolley M, Lipson H, . 2009. Hydrodynamically tunable affinities for fluidic assembly. Langmuir. 25:3769–3744.
- Mayeux R, Early MD. 2010. Alzheimer's disease. N Engl J Med 23: 2194–2201.
- Morgan JE. 2004. Circulation and axonal transport in the optic nerve. Eye 18:1089–1095.
- Mthunzi P, Lee WM, Riches AC, . 2010. Intracellular dielectric tagging for improved optical manipulation of mammalian cells. IEEE J Quantum Electronics 16(3):608–618.
- Ou-Yand HD, Wei MT. 2010. Complex fluids: Probing mechanical properties of biological system with optical tweezers. Annu Rev Phys Chem 61:421–440.
- Paus T, Toro R. 2009. Could sex differences in white matter be explained by g ratio? Neuro 3(14):1–7.
- Piyatamrong B, Kulsirirat K, Mitatha S, . 2010. Dynamic potential well generation and control using double resonators incorporating in an add/drop filter. Mod Phys Lett B 24:3071–3082.
- Prabhu AM, Tsay A, Han Z, Van V. 2010. Extreme miniaturization of silicon add-drop microring filters for VLSI photonics applications. J IEEE Photon 2(3):436–444.
- Schmidt BS, Yang AHJ, Erickson D, Lipson M. (2007). Optofluidic trapping and transport on solid core waveguides within a microfluidic device. Optics Expres.1(22):14322–14334.
- Schnitzer MJ, Block SM. 1997. Kinesin hydrolyses one ATP per 8-nm step. Nature 388:386–390.
- Schulz M, Crepaz H, Schmidt-Kaler F, . 2007. Transfer of trapped atoms between two optical tweezer potentials. J of Mod Opt 54: 1619–1626.
- Segev M, Christodoulides DN, Rotschild C. 2011. Method and system for manipulating fluid medium. US 2011/0023973 A. 2011 Feb 3.
- Selkoe DJ. 2001. Alzheimer's disease genes, proteins and therapy. Physiological Reviews 81(2):741–766.
- Shipton OA, Leitz JR, Dworzak J, Vargas-Caballero M. 2011. Tau protein is required for amyloid induced impairment of hippocampal long-term potentiation. The Journal of Neuroscience 31(5):1688–1692.
- Steven MB, Goldstein L, Schnapp BJ. 1990. Head movement by single kinesin molecules studied with optical tweezers. Nature 348: 348–352.
- Suwanpayak N, Jalil MA, Aziz MS, . 2011a. Molecular buffer using a PANDA ring resonator for drug delivery use. Int J Nanomed 6: 575–580.
- Suwanpayak N, Jalil MA, Teeka T, . 2011b. Optical vortices generated by a PANDA ring resonator for drug trapping and delivery applications. Bio Med Opt Express. 2(1):159–168.
- Svoboda K, Block SM. 1994. Biological applications of optical forces. Annu Rev Biophys Biomol Struct 23:247–283.
- Svoboda K, Schmidt CF, Schnapp BJ, Block SM. 1993. Direct observation of kinesin stepping by optical trapping interferometry. Nature 365:721–727.
- Szobota S, Isacoff EY. 2010. Optical control of neuronal activity. Annu Rev Biophy 39:329–348.
- Tasakorn M, Teeka C, Jomtarak R, . 2010. Multitweezers generation control within a nanoring resonator system. Opt Eng 49:075002.
- Xia X, Hu Z, Marquez M. 2005. Physical bonded nanoparticle network: A novel drug delivery system. J Controlled Release 103:21–30.
- Yang AJH, Erickson D. 2010. Optofluidic ring resonator switch for optical particle transport. Lab Chip 10:769–774.
- Ying J, Ling Y, Westfield LA, . 2010. Unfolding the A2 domain of von Willebrand factor with the optical trap. Biophys J 98(8): 1685–1693.