Abstract
Radial diffusivity profiles of atomized (MC, d = 1800 ± 200 µm) and inkjet-printed (MI, d = 40 ± 5 µm) alginate-based artificial cells have been generated using 2D Fluorescence Microscopy. The passive outward diffusion of FITC-Dextrans from MIs (0.5% LV alginate/15% CaCl2 coated with 0.5% Chitosan) and MCs (1.5% MV alginate/1.5% CaCl2) was measured and quantified using a Fickian model. As an expected outcome of miniaturization, the ratios of the outer layer diffusivities defined as DMIout/DMCout were 4.25 and 5.07 respectively for the 4 and 70 kDa markers, indicative of the enhanced diffusive potential of the miniaturized capsules.
Introduction
Determining the permeability of biocompatible hydrogels is crucial in biomedical research for the design and development of drug delivery systems, scaffolds in tissue engineering and implants in regenerative medicine (Chang Citation2007, Li Citation2009, Yu et al. Citation2011, Ciofani et al. Citation2008, Chaudhary et al. Citation2010). Several established methods are currently used to measure pore sizes of cross-linked hydrogels namely laser interferometry, chromatographic breakthrough curves, fluorescence recovery after photobleaching (FRAP), and spectrophotometry (Westrin et al. Citation1994, Liu et al. Citation2009, Lee et al. Citation2011). Diffusion of healing nutrients and drugs across the membrane is inversely proportional to the spherical-shaped artificial cell radius and proportional to the surface to volume ratio (S/V) (Fournier Citation2011). Fick’s second law in spherical coordinates describing the transient solute radial diffusion as a function of diffusivity (D) across the membrane is given by Eq. (1). The Renkin-Curry equation used to estimate free solution diffusivity is given by Eq. (2) and the Stokes’ radius (a) calculation for the molecular weight cutoff (MWCO) is given by Eq. (3).
In this article, passive outward diffusion studies will be conducted on atomized macrocapsules (MC)s and miniaturized inkjet capsules (MI)s. Existing diffusion models require cumbersome numerical solutions (Kwok et al. Citation1991). In order to circumvent this shortcoming, an analytical diffusion model (Asi et al. Citation2012, Simpliciano and Asi Citation2012) based on Fickian diffusion will be used for diffusivity determination of known fluorescent MW markers (FITC-Dextrans) across both types of artificial cell membranes.
The use of fluorescence microscopy previously used to track diffusion qualitatively across MCs (Prakash and Martoni Citation2006, Mobed-Miremadi Citation2012) will be extended to modeling diffusivity across MCs and MIs. Using this approach, researchers in the fields of regenerative medicine and tissue engineering can model fluorescent micromeasurements related to intramembrane fluxes with ease and confidence, thus cluster trends by artificial cell size.
Materials and methods
Materials
All chemicals used to make the capsules were purchased from Sigma Aldrich: low molecular weight sodium-alginate (LV) (A0682, 12–80 kDa), medium molecular weight alginate (A2033, µ > 2 000 cP), low molecular weight chitosan (44,886-9, 75% deacetylated, 3.8–6 kDa), and fluorescein isothiocyanate dextran markers abbreviated as FITC- Dextran markers (46947, FD70S, FD4). The piezo jetting device serial number purchased from Microfab Technologies was MJ-B10–49-02.
Methods
The experiments were designed to meet a single original research objective, specifically to compare the diffusivities of alginate-based MCs and MIs in order to assess the effect of increase of (S/V) as a result of miniaturization in terms of diffusive potential.
Artificial cell fabrication and size measurement
Atomization
Macrocapsules were fabricated using the methodology in reference (Prakash and Chang Citation1995). A 1.5% medium viscosity sodium-alginate solution (µ = 500 cP, γ = 45 dyn.cm‐1) was jetted into a 1.5% CaCl2 bath. The air (FA) and liquid (FL) flow rates were adjusted to 1.5 L/min and 0.5 ml/min, respectively. The atomizer needle assembly is a concentric 24 G needle surrounded by a 16 G needle, through which the sodium alginate and air flows. The calcified sodium-alginate beads were then washed with 0.9% NaCl twice.
Inkjet bio-printing
Microcapsules were fabricated using Microfab’s Jetlab System using the methodology in reference (Mobed- Miremadi et al. Citation2011). The apparatus consists of a CCD camera (30 fps), a control unit, a print-head, a triggering unit, a fluid delivery unit, and a PC equipped with proprietary software (MicroFab JetServer) to tune the bio-ink formulation to the jetting parameters. The print head has an aperture of 60 μm and is rated for μ = 40 cP and γ = 72 dyn.cm‐1. After inputting the jetting variable settings, droplet generation begins with the triggering box sending electrical signals to the inkjet control unit and to the CCD camera control PC, simultaneously. The inkjet engine fires the 0.5% low viscosity sodium alginate solution (µ = 5 cP, γ = 43 dyn.cm21) through the print head into a 15% (w/v) CaCl2 solution. The parameters for jetting are listed in . After jetting, capsules were allowed to cross-link in the 15% CaCl2 solution for 30 min. Then 1% (w/v) chitosan was added into the 15% CaCl2 solution to make the final chitosan concentration 0.5% (w/v). Capsules were coated for an additional 30 min. This physical adsorption step carried out at room temperature using stirring is necessary to confer mechanical strength to the suspension during the concentration and centrifugation steps (Simpliciano and Asi Citation2012). Next, the capsules were centrifuged at 8 000 g for 5 min and washed with a 0.9% (w/v) NaCl solution 3 times.
Table I. Operating parameters for inkjet printing.
Size measurement
Capsule sizes and membrane thicknesses were measured using a Nikon transmission microscope/camera (Nikon EclipseTi-S) equipped with an Andor Technology Interline CCD camera.
Fluorescence measurements
Calibration solution and sample preparation
Calibration stock solutions ranging from 0.1 to 15.1 mg/ml for each FITC-Dextran MW standard (4, 70, and 500 kDa or stokes radii of 1.4, 5.8, and 14.5 nm) dissolved in 0.9% (w/v) NaCl were prepared. In dilute solutions, there is a linear relationship between intensity and the concentration of the fluorescent marker under observation (Guilbault Citation1990). Hence calibration and diffusion measurements were carried out over this range.
Subsequently, 10 μl of MI or MC solution was then incubated in 1 ml of FITC solutions for 24 h prior to imaging. The solution was centrifuged at 8 000 g for 5 min and washed once with a 0.9% (w/v) NaCl solution.
Fluorescence imaging
A 4 μl sample of sample was pipetted onto a microscope slide and observed under the fluorescent microscope. Prior to sampling the FITC/Acridine Orange filter was chosen from the imaging software (NIS-Elements v.3.2.2) filter selection feature to accommodate the excitation and emission wavelengths of 468 and 520 nm of the FITC molecule. Image acquisition and capture were conducted for a single capsule at a time at three random radial positions as depicted in . Images were captured every 30 s at 12 fps for a 30 min period. The imaging software region of interest (ROI) tool was then used to determine the fluorescent intensity of the FITC samples. At all times the pseudo- coloring feature was used to enhance the contrast between the background and the sample.
Figure 1. Sample image acquisition and analysis using the ROI tool to establish the radial fluorescent intensity profiles for the characterization of the outward diffusion of the FITC-Dextran 70 kDa marker from the microcapsules. Shown in numbered white boxes are sample radii under observation for fluorescent intensity monitoring.
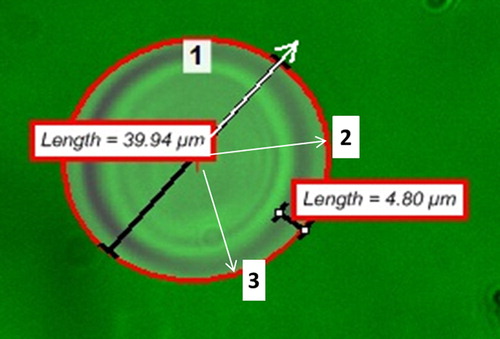
Prior to experimentation, the signal to noise ratio (S/N) was optimized to reduce the extent of photo-bleaching to 2.0% using a 0.27 mg/ml FITC dextran. In addition, the auto-fluorescence of empty MCs and MIs was measured to be negligible.
Modeling diffusivity
The solution to Eq. (1) solved analytically using Maple 15.0 is given by Eq. (4a) (Asi et al. Citation2012, Simpliciano and Asi Citation2012). The initial and boundary conditions are given by Eqs. (4b)–(4d). Residual sum of squares (RSS) minimization was coded in MATLAB 2010a to generate the radial profile of diffusivity.
The term C0 correspond to the initial concentration of FITC-Dextran solution into which the capsules where incubated. The equilibrium concentration is denoted as Ceq and rout is the radius of the spherical capsule. As stated above in the calibration section, concentration (C) and fluorescent intensity (I) are correlated by a linear relationship in dilute solutions.
Three types of diffusivities were calculated based on the delineation presented in . Average core diffusivity (Dcore) were calculated based on the distance from the core to the inner membrane. Average membrane (Dm) and outer layer (Dout) diffusivities were calculated based on the entire membrane thickness and the last quartile of the membrane thickness, respectively.
Three capsules were examined for each artificial cell fabrication method on which the average above-mentioned diffusivities are based.
Results
A sample mean fluorescent intensity profile as function of time representing the outward diffusion of the 4 kDa marker from the MIs and corresponding realtime snapshots are presented in and , respectively. Sample outward diffusivity plots as a function of radial distance to the capsule core are presented in Figures 5a and 5b for the 4 kDa marker. For the 500 kDa molecule, intensity values inside the MIs and MCs were very close to background intensity values reflected in the low S/N (< 1.5) ratio, hence no diffusivities were calculated.
Figure 3. Mean fluorescent intensity profile as a function of time for the outward diffusion of the FITC-Dextran 4 kDa marker from microcapsules.

Figure 4. Snapshots of outward diffusion of the FITC 4 kDa marker from microcapsules captured at (a) 5 s, (b) 28 s, (c) 57 s, (d) 92 s, (e) 120 s, and (f) 180 s. Shown on each image is the pseudo-color intensity scale.
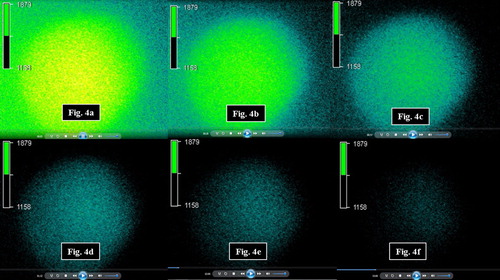
Average membrane (Dm), Renkin Curry Diffusivity (Dfree), core diffusivity (Dcore), and outer layer diffusivities (Dout) per capsule type/MW marker are presented in . A set of observations applicable to both capsule types are the following: (a) A sharp decrease in D values is observed at the membrane/solution interface, and the diffusivity is constant near the core reinforcing the validity of the second boundary condition (dC/dr = 0 at r = 0), (b) Dout in the outermost membrane layer is higher for the MIs while the Dcore and Dm are higher for MCs, (c) Dout and Dm are inversely proportional to the MW of the marker, and (d) The membrane is impermeable to the 500 kDa marker.
Table II. Comparative summary of diffusivity calculations for microcapsules and macrocapsules.
Discussion
The above quantitative findings are in agreement with the first qualitative comparison of diffusivity measurements between atomized and inkjet bio-printed capsules conducted using the 4 kDa marker specifically with regard to the enhanced diffusive properties of the miniaturized artificial cells and the lack of permeability of both capsule types to the 500 kDa marker (Mobed-Miremadi Citation2012).
Using spectrophotometry, for an alginate concentration of 0.5% (w/v) and uncoated miniaturized capsules, membrane diffusivities (Dm) of 6.64 × 10‐6 m2/s and 1.9 × 10‐7 m2/s have been reported by modeling the transient kinetics of release using an unspecified Fickian solution for methylene blue (MW = 320 Da, a = 0.5 nm) and vitamin B12 (MW = 1355 Da, a = 0.82 nm), respectively (Dohnal and Štěpánek Citation2010). Although, these values are at least 6 orders of magnitude lower than the membrane diffusivities calculated in this article using fluorescence microscopy, because of differences in the cross-linking solution and Stokes’ radii of the solutes no valid comparisons can be drawn. For MCs using spectrophotometry, Dm for albumin (MW = 66.7 kDa, a = 2.98 nm) using the same analytical model given by Eq. (4) was determined to be 2.00 × 10‐16 m2/s (Simpliciano and Asi Citation2012) as compared to a value of 3.26 × 10‐14 m2/s obtained using the fluorescence imaging method and 70 kDa dextran (a = 3.02 nm). With a 163 fold difference in diffusivity values, this comparison should be conducted with caution given that the electrostatic repulsive forces between the negatively charged alginate membrane and albumin at pH = 7.4 absent in the case of uncharged Dextrans.
The ratios of the average outer layer diffusivities where the bulk of diffusion occurs, defined as DMIout/DMCout were 4.25 and 5.07 with calculated DMIout values calculated to be 7.70 × 10‐14 m2/s and 3.02 × 10216 m2/s for the 4 and 70 kDa markers, respectively. This enhancement in diffusive properties is an expected outcome of the miniaturization, however, according to theory, a 45 fold increase corresponding to the increase in (S/V) should have resulted from the scale-down (Fournier Citation2011). An unexpected outcome of the miniaturization was that diffusion through the core of the MCs (1.5% MW alginate/1.5% CaCl2) has been measured to be up to 929 faster as compared to MIs (0.5% LV/15% CaCl2) based on the 4 kDa diffusive profile, thus inferring a lower number of cross-links per surface area for the larger capsules at a higher alginate concentration. In fact, for both 4 kDa and 70 kDa markers, the ratio of (Dcore/Dfree) was respectively calculated to be 0.39 and 0.98, supporting the existence of a semi-hollow core for the macrocapsules. The upper range of alginate concentration for the miniaturized capsules has been limited by the print head’s rheological rating compensated for by a higher CaCl2 concentration to maintain an equivalent cross-linked mesh size to the MCs. For future studies, Atomic Force Microscopy (AFM) will be used for pore size optimization of the chitosan-coated MI membrane and Scanning Electron Microscopy (SEM) will be used for core characterization. With respect to quantifying the measurement accuracy of this imaging method, future efforts will consist of varying capture rate (fps) and artificial cell core fluidity tuned by citrate treatment time.
At last the clear advantages of the use of fluorescence microscopy over spectrophotometry extensively used as a control method to draw comparisons are twofold: (a) single and/or multiple artificial cells can be simultaneously examined using the ROI feature as compared to bulk solution measurements, (b) the MIs can only be tested using spectrophotometry using a concentrated slab/pellet conformation non-representative of their diffusive behavior in solution.
Conclusion
During this research, a reproducible methodology was developed to quantitatively determine the outward diffusion of FITC-Dextran 4, 70, and 500 kDa from alginate- based artificial cells and through the use of 2D fluorescence microscopy combined with Fickian modeling. The determination of diffusivity coefficients through inkjet bio-printed capsules is an original contribution due to the novelty of the reproducible and high throughput inkjet printing technique applied to alginate-based artificial cell miniaturization. As an expected outcome of miniaturization, the ratios of the average outer layer diffusivities defined as DMIout/DMCout were 4.25 and 5.07 for the 4 and 70 kDa MW markers respectively, indicative of the higher diffusive potential of MIs. Future efforts will be focused on studying the effect of capsule miniaturization and MW cutoff in the 20–70 kDa range in order to tune the permeability of the MIs for specific therapeutic windows of solute release and comparative pore size characterization between MCs and MIs using AFM and SEM. These studies will in turn be invaluable in examining cell growth in the miniaturized capsules’ micro-environment.
Acknowledgement
The authors would like to acknowledge the Davidson’s College of Engineering Faculty Development grant “Inkjet Bioprinting” and C-SUPERB Joint Venture Grant “Bio- Printing of Mammalian Cells” for funding this effort.
Declaration of interest
Authors have no declaration of interests to support.
References
- Asi B, Parasseril J, Tat M, Wong E. 2012. Comparative diffusivity measurements between spectrophotometry and fluorescence microscopy. [online] Available at http://www.engr.sjsu.edu/∼bmes/BMDConf2012/abstract5.html for free access. Accessed on 6/25/2012.
- Chang TMS. 2007. Artificial Cells: Biotechnology, Nanomedicine, Regenerative Medicine, Blood Substitutes, Bioencapsulation, Cell/Stem Cell Therapy. Singapore: World Scientific Publishing Co. Pte. Ltd, London: Imperial College Press, London [online] Available at: www.artcell.mcgill.ca for free access or download. Accessed on 6/9/2012.
- Chaudhary A, McShane MJ, Srivastava R. 2010. Glucose response of dissolved-core alginate microspheres: towards a continuous glucose biosensor. Analyst. 135:2620–2628.
- Ciofani G, Raffa V, Pizzorusso T, Menciassi A, Dario P. 2008. Characterization of an alginate-based drug delivery system for neurological applications. Med Eng Phys. 30:848–855.
- Dohnal J, Štěpánek F. 2010. Inkjet fabrication and characterization of calcium alginate microcapsules. Powder Technol. 200:254–259.
- Fournier RL. 2011. Basic Transport Phenomena in Biomedical Engineering, 3rd ed. Philadelphia: Taylor &Francis.
- Guilbault GG. 1990. Practical Fluorescence (Modern Monographs in Analytical Chemistry). 2nd ed. New York: Marcel Dekker Inc.
- Kwok WY, Kiparissides C, Yuet P, Harris J, Goosen MFA. 1991. Mathematical modeling of protein diffusion in microcapsules. Can J Chem Eng. 69:361–370.
- Lee YH, Chang JJ, Lai WF, Yang MC, Chien CT. 2011. Layered hydrogel of poly(γ-glutamic acid), sodium alginate, and chitosan: Fluorescence observation of structure and cytocompatibility. Colloids Surf B Biointerfaces. 86:409–413.
- Li H. 2009. Smart Hydrogel Modeling. Singapore: Springer.
- Liu J, Zhang Y, Yang T, Ge Y, Zhang S, Chen Z, et al. 2009. Synthesis, characterization, and application of composite alginate microspheres with magnetic and fluorescent functionalities. J Appl Polym Sci. 113:4042–4051.
- Mobed-Miremadi M. 2012. High Throughput Methods for Miniaturization of Implantable Artificial Cells. In: Chang TMS. Ed. Selected Topics in Nanomedicine. Singapore: World Scientific Publishing. In Press.
- Mobed-Miremadi M, Acks E, Polasaward S, Chen D. 2011. High throughput miniaturization of artificial cells. Artif Cells Blood Substit Biotechnol. 39:310–316.
- Prakash S, Chang TM. 1995. Preparation and in vitro analysis of microencapsulated genetically engineered e. coli DH5 cells for urea and ammonia removal. Biotechnol Bioeng. 46:621–626.
- Prakash S, Martoni C. 2006. Towards a new generation of therapeutics. Appl Biochem Biotechnol. 128:1–21.
- Shan Y. 2011. Viability of Microencapsulation of E. Coli for Urea Removal Using Inkjet Bio-Printing, MS Project Report. [online] Available at http://www.engr.sjsu.edu/ges/media/pdf/mse_prj_rpts/fall2011/. Accessed on 6/25/2012.
- Simpliciano C, Asi B. 2012. Pore size determination and validation using AFM and spectrophotometry. [online] Available at http://www.engr.sjsu.edu/∼bmes/BMDConf2012/abstract4.html for free access. Accessed on 6/25/2012.
- Westrin B, Axelsson A, Zacchi G. 1994. Diffusion measurement in gels a review. J Contr Release. 30:189–199.
- Yu W, Song H, Zheng G, Liu X, Zhang Y, Ma X. 2011. Study on membrane characteristics of alginate-chitosan microcapsule with cell growth. J Membr Sci. 377:214–220.