Abstract
Radiosurgery involves the precise delivery of sharply collimated high-energy beams of radiation to a distinct target volume along selected trajectories. Historically, accurate targeting required the application of a stereotactic frame, thus limiting the use of this procedure to single treatments of selected intracranial lesions. However, the scope of radiosurgery has undergone a remarkable broadening since the introduction of image-guided robotic radiosurgery. Recent developments in real-time image guidance provide an effective frameless alternative to conventional radiosurgery and allow both the treatment of lesions outside the skull and the possibility of performing hypofractionation. As a consequence, targets in the spine, chest and abdomen can now also be radiosurgically ablated with submillimetric precision. Meanwhile, the combination of image guidance, robotic beam delivery, and non-isocentric inverse planning can greatly enhance the conformality and homogeneity of radiosurgery. The aim of this article is to describe the technological basis of image-guided radiosurgery and provide a perspective on future developments. The current clinical usage of robotic radiosurgery will be reviewed with an emphasis on those applications that may represent a major shift in the therapeutic paradigm.
Introduction
Radiosurgery is a very precise focal radiation treatment for well-defined tumors and selected non-neoplastic disorders. By very accurately aiming sharply collimated beams of radiation from multiple directions, a biologically highly effective dose of radiation can be restricted to the target volume while minimizing toxicity within surrounding tissue. This simple concept was conceived and first implemented by Lars Leksell, a Swedish neurosurgeon, whose initial interest was largely the treatment of functional disorders of the central nervous system (CNS) such as trigeminal neuralgia; he also coined the term radiosurgery to describe his technique Citation[1].
Leksell spent the earliest part of his career developing an arc-based stereotactic frame, primarily for use in functional neurosurgery. Attesting to its elegant design, this instrument is still widely used. In seeking to make functional neurosurgery even less invasive, Leksell conceived the notion of coupling his stereotactic frame to an extremely high “destructive” dose of radiation, thereby allowing this device to be used as an ablative surgical tool. After years of effort, Leksell and his team produced a clinically reliable stereotactic radiosurgical device, the GammaKnife.
Starting in 1968, Leksell began treating patients with the first prototype, an instrument that used 179 convergent cobalt-60 radiation sources as its “cutting” tool. Subsequently, the number of sources was increased to 201, as in current models.
Despite its conceptual origins as a tool for functional neurosurgery, the first GammaKnife treatment was delivered to a 20-year-old patient harboring a solid craniopharingioma. This patient enjoyed an excellent outcome with a follow-up of over 27 years Citation[2]. While the modern GammaKnife is a superb and widely available tool for treating brain lesions Citation[3], its application is limited to intracranial targets. This limitation stems from the requirement that a lesion being radiosurgically ablated must be rigidly and precisely fixed inside the GammaKnife at the point where the beams cross. Leksell's stereotactic frame continues to provide the mechanism by which the head is fixed and the target localized. However, attachment of the frame to a patient's head is fairly time-consuming and obviously painful. Furthermore, by design, all the Co60 sources of the GammaKnife are arranged in a near-hemispheric array, with the beam axes crossing at a common point in space known as the isocenter. Because beam collimation is circular, the high dose volume at the point of intersection is a sphere, which by definition has come to describe the volume contained within the 50% isodose. Importantly, the dose gradient produced by the GammaKnife is sufficiently steep that only a few millimeters of displacement result in a significant decrease in the delivered dose, provided that the treatment volume is not too large (e.g., over 10 cc). Conformal GammaKnife treatment of non-spherical lesions (which constitute the vast majority of tumors) is achieved through the use of multiple isocenters. This process requires a treatment planning technique called sphere packing (described in detail later). The technique for sphere packing entails shifting a patient's head position along consecutive isocenters. This can be done either manually or robotically as with the GammaKnife Model C. Selected beam trajectories can also be blocked to further improve conformality and sparing of normal tissue.
Gantry-based LINAC radiosurgery systems use a single beam of radiation that rotates around a fixed point as a high-energy (most often 4–6 Mv) radiation effector Citation[4]. However, LINAC systems are based on standard equipment developed for conventional radiation therapy and have limited mechanical flexibility. Similar to the GammaKnife, LINAC radiosurgical systems are resticted to isocentric treatments. Nevertheless, in most modern LINAC systems beam shaping, by means of computer-controlled multi-leaf collimation, permits added conformality in non-spherical targets. When this process is additionally combined with algorithms for dose optimization, it is termed Intensity Modulated Radiation Therapy (IMRT). The first practical IMRT system was developed by a neurosurgeon, Mark Carol, in the 1990s. Regardless of the method by which the radiation field is shaped, all these new treatment modalities require the application of a stereotactic frame for precise treatment delivery, thus largely precluding the ability to ablate extracranial lesions. In the absence of a system for precision targeting outside the head, radiation is generally administered to extracranial lesions using conventional daily fractionation. This method of treatment protects the normal tissue that is irradiated, but only at the cost of considerable time and often significantly reduced efficacy relative to the more aggressive radiosurgical approach.
Extending radiosurgery to targets in the chest and abdomen has been an area of active research in recent years. Because tumors in the chest and abdomen move during respiration, treatment with radiosurgery is particularly challenging. Conventional systems cannot directly track motion. Gating and breath-holding are two newer techniques for addressing the problem of respiratory motion with conventional radiotherapy systems Citation[5], Citation[6]. In the case of gating, the therapeutic beam is switched off whenever the tumor is outside a predefined motion window Citation[7]. Similarly, with breath-holding, the patient is asked to hold his/her breath for a short period of time, during which the beam is activated Citation[8–10].
Image-guided robotic radiosurgery is now emerging as an important tool for managing many extracranial lesions. Real-time image guidance provides a uniquely flexible method for detecting target motion, while dynamically compensating for this movement by means of the robotic arm. The most advanced techological expression of image-guided robotic radiosurgery is the CyberKnife (Accuray Inc., Sunnyvale, CA). In the course of this paper, the authors will discuss the technological underpinnings of and their clinical experience with this device.
Image-guided robotic radiosurgery
The basic concept of robotic radiosurgery is simple. A robotic arm moves a small linear accelerator through a series of positions. From each position, beams can be directed towards different areas of the target region, leading to a large number of non-isocentric, non-coplanar beams. Since the beams are non-coplanar and non-isocentric, the possibilities available to the treating surgeon far exceed those offered by conventional systems (). As noted above, both GammaKnife and LINAC treatments are inherently isocentric, and a stereotactic frame is mounted to the head to immobilize the patient. The definition of target volume and organs at risk is then done relative to the frame, which remains attached throughout the treatment. Frames may move over the course of image acquisition, treatment planning and dose delivery, introducing some degree of inaccuracy. In this respect, frame-based treatments are essentially based on a “historical” definition of the position of the lesion with respect to the frame.
Figure 1. a) Isocentric beams from various directions leading to an inhomogenous distribution of beams, i.e., some target areas are not covered (black) and there is a single spot where all beams intersect (light gray). b) Non-isocentric beams from various directions. The beams do not all cross at a single point.
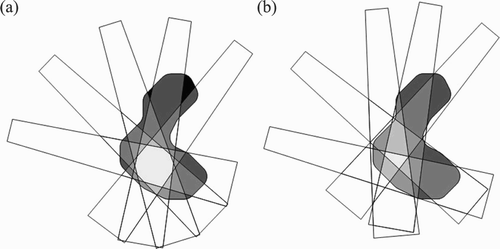
A more flexible and less painful approach is the use of intraoperative imaging to identify the tumor position instead of using a frame to fix the patient. This type of treatment is called image-guided or frameless radiosurgery. The combination of image guidance and robotics offers one significant advantage over conventional approaches: the beam can track patient motion in any direction. Real-time image guidance provides a continuous updating of the lesion's position with respect to an invariable reference such as the patient's anatomy.
Several sensor systems for tracking patient motion and respiration have been described in the literature Citation[11–15]. The basic sensors used in this context fall into two categories: external imaging and internal imaging. External sensors record the external position of the patient, often together with a respiratory state. Examples of such sensors are infrared tracking cameras, optical cameras and laser rangefinders. Typical disadvantages of external imaging include the fact that the target may not always move in the direction indicated by the external sensor. For instance, respiration may move a tumor close to the diaphragm in the inferior-superior direction while the abdominal skin surface moves in the anterior-posterior direction.
The second type of sensors are internal sensors. Examples are X-ray imaging and ultrasound. The images obtained from such internal sensors must be segmented to obtain the tumor position. This is difficult given the real-time and speed requirements of the application. In the context of robotic radiosurgery, a new tracking method has emerged which overcomes the limitations of both sensor types Citation[16], Citation[17]. This method is based on the correlation between external and internal motion, and will be described in more detail in the next section.
Motion compensation in radiosurgery
Organs move during treatment, especially as a result of respiratory motion Citation[6], Citation[13], Citation[18–21]. Conventional systems require a margin around the tumor (). Unlike other treatment systems, the robotic arm can move the LINAC in any direction. Taking into account the nature of the movements, it becomes feasible to compensate for breathing motion.
Figure 2. Tumor motion may result in partial underdosage. Without motion compensation, a margin is required. This leads to a substantial increase in integral radiation dose.
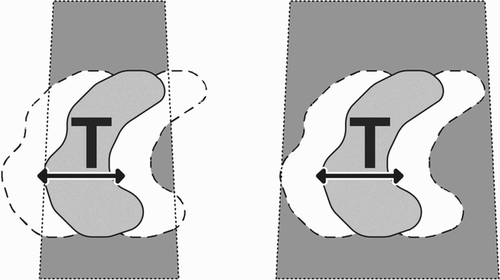
To this end, a sensor system must determine the tumor position with very high speed. For example, a tumor moving with a speed of 1 cm/second will be found 3 mm away from its expected position if the sensor's latency time is 0.3 s. Typical image registration algorithms (especially elastic registration) require multiples of these latency times. Therefore, the method for tracking in robotic radiosurgery combines a fast external real-time sensor with (slow) internal imaging. The real-time sensor can only track points on the patient's skin surface. In correlation with the internal sensor, a very accurate position can be determined. To acquire a real-time signal of the respiration, infrared emitters are attached to a vest on the patient's chest and the infrared tracking system records the motion of these emitters. A stereo X-ray camera system (X-ray cameras with nearly orthogonal visual axes) records the position of gold markers, injected through an 18-gauge biopsy needle into the vicinity of the target area under CT monitoring (). In addition, hardware means for capturing the time points of X-ray image acquisition and infrared sensor acquisition are provided. These hardware means record time stamps, i.e., the time points at which the readings/images were taken, which are needed to synchronize the two sensors. Given the data of gold marker positions and external infrared emitter positions, a correlation model is computed.
Figure 3. Live X-ray to determine the target position, using gold markers (circled in red) and infrared emitters (indicated by blue arrows). [Color version available online.]
![Figure 3. Live X-ray to determine the target position, using gold markers (circled in red) and infrared emitters (indicated by blue arrows). [Color version available online.]](/cms/asset/18e88d79-5434-4e6d-ba28-4770b4306789/icsu_a_188564_f0003_b.jpg)
The external markers can be tracked with optical methods at very high speed. Updated positions can be transmitted to the control computer more than 20 times per second. However, external markers alone cannot adequately reflect internal displacements caused by breathing motion. Thus, the position of the internal gold fiducials is determined at a lower frequency and the correlation model is updated accordingly, e.g., to allow for spontaneous changes in the patient's breathing pattern.
While the external marker positions can be determined frequently, the latency caused by the robot's control system would still leave the robot trailing the actual target position. Due to its cyclic nature, it is possible to predict respiratory motion over a short interval of time () Citation[16], Citation[17], Citation[22], Citation[23]. This prediction can be used to infer the target position at time t0 + latency, thus positioning the LINAC to irradiate the planned region in the target.
Treatment planning for robotic radiosurgery
Treatment planning is typically performed on CT image data, but other image modalities (e.g., MRI, fMRI) can be used in addition. In the planning phase, beam directions and beam weights are defined by the surgeon to meet criteria for a suitable distribution of radiation dose. Given the enormous number of possibilities for placing beams (and combinations of beam placements), the goal is to find a beam placement scheme which returns highly conformal distributions for any irregular target shape Citation[24], Citation[25]. Obviously, it would not seem practical to place all beams by hand. Therefore, an inverse planning scheme is needed for the special case of robotic radiosurgery.
Inverse planning specifies a dose distribution goal and attempts to find directions and activation durations for each beam such that the desired distribution can be achieved. First, a large number of non-isocentric, non-coplanar beams is generated, then an optimization method is used to compute beam weights such that dose constraints are fulfilled. We first describe the placement of the beams, then the methods for computing their weight. Due to the large positioning flexibility of a robotic arm, the placement of beams could be done in many ways. In addition, a large number of beams must be placed: Typically, over 100 beam directions are used in one treatment. The beams in conventional radiosurgery are cylindrical with diameters ranging from 5 to 60 mm. The same is true for current robotic radiosurgery, i.e., the beams are cylindrical. One simple method of irradiating a tumor of irregular shape is sphere packing. Placing a set of beams in such a way that all beam axes coincide at a single point P will produce a treated volume of spherical shape. P is then called the isocenter of the treatment. Sphere packing attempts to place several spheres into an (irregular) tumor shape, such that the tumor is fully covered. Overlap between the spheres must be avoided, as this would result in “hot spots”, i.e., undesirable overdosage of subregions within the target (). However, robotic radiosurgery does not use sphere packing.
To illustrate the method for placing beams for robotic radiosurgery, first consider a target volume with the shape shown in . This shape is obtained by sweeping a spherical object along a line segment. A natural extension of isocentric beam placement would thus be to place a series of “isocenter points” along the central line segment of this shape (). Each of these isocenter points is then treated as if it were the center of a spherical target volume. The result is an overlay of a series of spherical volumes. The main difference between this scheme and sphere packing is that now the spheres do overlap, while homogeneity is achieved by the sweeping process. High homogeneity of dose can be achieved by this scheme throughout the entire volume in , simply by giving all beams thus placed the same dose weight. Now the question remains as to how beams for arbitrarily shaped target volumes can be placed automatically.
A straightforward extension of the above line-sweeping placement scheme is illustrated in . Thus, the outer boundary of the target volume is retracted, and a series of isocenter points are placed on the retracted surface. Sweeping is then applied to this retracted surface. Experimentally, it transpires that even dose weights for all beams (as in ) thus placed will not always give the best results. Therefore, we extend the above sweeping scheme by an optimization step: A set of inequalities representing beam weights as well as upper and lower dose thresholds is solved by linear programming to obtain the optimized weights for each beam. It has been found experimentally that the above scheme for placing beams works best with the following modification: Instead of placing isocenters onto a retracted surface, the isocenters are best placed directly on the surface itself. This modification has the advantage that the remaining variable, namely the degree of retraction, has now been eliminated.
Overall, the inverse planning method described by Schweikard et al. Citation[26] proceeds as follows. First, the outer boundary of the target is delineated. A series of points is then computed such that each point is on the outer target surface and the points are evenly spaced. A small number (typically 2–4) of beam directions with random orientation in space is then placed through each such isocenter point. In a second step, the weights of the beams are computed with linear programming. Thus, linear programming computes durations of activation for each beam by solving a large system of inequalites. Experimentally it was found that a larger number of input beams (i.e., 1000) should be used in the weighting step. The actual number of beams needed to obtain an acceptable local optimum depends on a number of parameters, including the beam diameter and the size of the target volume. However, the value of the objective function stops improving rapidly once a sufficiently large number of beams has been placed. In , the number of monitor units is plotted against the number of candidate beams, with all other constraints fixed. As the beam placement contains a random component, the experiments have been repeated multiple times and the results are shown as boxplots. While the number of monitor units and the variance improve substantially until approximately 1500 candidate beams are reached, a further increase in the number of candidate beams does not yield large improvements.
Figure 8. Experimental results showing the influence of the number of candidate beams on the number of monitor units. All other constraints were fixed and the tests were repeated multiple times for different random beam sets. The boxes give the maximum, upper quantile, mean, lower quantile, and minimum values. Any increase in the number of candidate beams beyond approximately 1500 does not lead to large improvements.
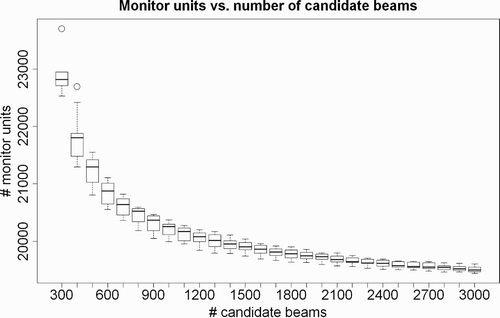
A characteristic feature of linear programming in this application is that the result of the optimization returns with many of the input beams set to zero weights. Typically, the first step of the inverse planning (computation of beam directions) generates approximately 1000 beam directions. The second step (optimization step with linear programming) will automatically select approximately 100–200 beam directions from this set, having non-zero activation time (see for an example). Comparative results obtained with this planning method have shown that this is the method of choice for robotic radiosurgery with cylindrical beams Citation[27].
Figure 9. 3D arrangement of non-isocentric treatment beams computed by inverse planning for robotic radiosurgery.
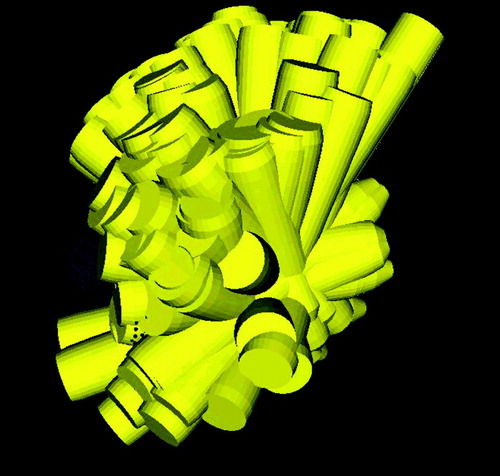
Recently, planning has been extended to the case of moving targets Citation[28], Citation[29]. However, while the target may move during treatment, critical structures in the vicinity may not move with the same speed or in the same direction. Relative motion of organs with respect to one another requires highly accurate planning. The temporal changes over the entire respiration cycle are represented in a motion model (obtained from either 4D CT or by deforming an inhalation image stack into an exhalation image stack). This motion model is used in 4D planning. Reference 30 shows that the linear programming approach extends to 4D inverse planning.
Clinical applications of image-guided robotic radiosurgery
The combination of real-time image guidance and robotic treatment delivery offers several distinct advantages over conventional frame-based radiosurgery. This design substantially augments the number of possible (beam) trajectories, and in some cases increases the volume of space over which they are distributed. As a consequence, the CyberKnife concept often provides enhanced dose conformality and homogeneity while simultaneously sparing normal tissue from needless irradiation. In addition, targeting based on X-ray image correlation, which in terms of accuracy has been demonstrated to be comparable to intracranial frame-based localization, enables extracranial and hypofractionated radiosurgery for the first time Citation[31], Citation[32].
By removing the inferiorly hanging stereotactic frame, image guidance opens up additional beam trajectories that can be advantageous for treating skull base lesions located on the floor of the anterior, middle and posterior cranial fossae. Furthermore, even more inferiorly placed tumors, such as those involving the foramen magnum and below, are readily accessible by radiosurgery for the first time. Because these beam trajectories can be designed to pass preferentially through the splanchnocranium, robotic radiosurgery lessens the need for brain irradiation in comparison to more conventional radiosurgical procedures. Enhanced brain tissue sparing may particularly benefit those patients affected by metachronous lesions requiring multiple radiosurgical treatments within a timeframe of several years (e.g., recurrent brain metastases).
The numerous technical attributes of robotic radiosurgery make it an attractive platform for investigating a range of new clinical applications. Clinical studies over the past few years have emphasized the hypofractionated treatment of larger intracranial tumors and those situated immediately adjacent to radiation–sensitive critical structures such as the optic nerves and the CN VII and VIII complex. Increasingly, however, robotic radiosurgery appears to be an extremely powerful tool for ablating a range of extracranial lesions located in the chest and abdomen. Although more than 2000 patients have been treated by robotic radiosurgery at Stanford University, the first clinical site using this technology, and more than 15,000 patients worldwide, the number of published clinical outcome studies to date is still somewhat limited. Nevertheless, the following will describe the clinical innovations and improvements introduced by robotic radiosurgery in the treatment of intracranial, spinal and other extracranial lesions.
Intracranial lesions
A number of different conventional frame-based devices are available to perform intracranial radiosurgery. However, because it is not practical to keep a stereotactic frame on a patient's head for more than a few hours, radiosurgery is almost invariably delivered in a single shot. In rare instances, hypofractionation is feasible with frames but requires a relatively short interval between fractions (e.g., 6 hours Citation[33]). The extent of normal tissue repair permitted by such a minimal interfraction hiatus may be less than ideal. In contrast, image guidance readily permits any length of time between radiosurgical sessions, and since there is some radiobiological advantage associated with intervals of up to and possibly even beyond 24 hours, most robotic radiosurgery has been administered with daily fractionation. By combining this approach with anywhere from 1 to 5 sessions, a very broad range of lesions can be successfully and safely ablated with radiosurgery, many of which were previously untreatable.
Peri-optic tumors
Hypofractionation is especially useful for treating lesions of the optic pathways or lesions involving other cranial nerves. Staged or multi-session radiosurgery to treat tumors of the optic pathways has proven to be a safe and effective option with an excellent degree of visual preservation and growth control. This treatment modality was intially described by Mehta et al. in 13 patients with tumors adjacent to the anterior visual pathways Citation[34]. Doses of 20–25 Gy were administered in 2–5 fractions separated by 24 hours. No evidence of tumor progression or visual deterioration was reported and vision improved in 4 patients. High rates of tumor control and preservation of visual function following hypofractionated robotic radiosurgery have also been described by the Stanford group Citation[35]. In this retrospective study, 20 patients with meningiomas and 14 patients with pituitary adenomas within 2 mm of the optic apparatus were treated with CyberKnife radiosurgery. Radiosurgery was delivered in 2–5 sessions to a cumulative mean marginal dose of 20 Gy. Overall growth control was achieved in 94% of patients and pre-surgical vision preservation in 91%. Only two cases of tumor progression were found, both associated with visual deterioration. Overall, vision was unchanged in 20 patients, improved in 10, and worse in 3. Both these series are remarkable for the excellent degrees of visual function preservation and growth control in a particularly challenging group of patients (who in many cases had undergone previous conventional radiotherapy). One of the authors (PR) has also treated 3 patients with optic nerve meningiomas inducing loss of visual acuity and restriction of visual fields. Hypofractionated image-guided robotic radiosurgery was used to deliver up to 20 Gy prescribed to the 80% isodose and delivered in 4 fractions. In all 3 cases, growth control in association with restoration of visual acuity and visual fields was observed (unpublished data).
Vestibular schwannomas
Hypofractioned image-guided robotic radiosurgery has been described for the treatment of vestibular schwannomas. Two groups have reported excellent rates of hearing preservation and tumor control Citation[36], Citation[37] following CyberKnife radiosurgery. Since 1999, the CyberKnife at Stanford University has been used to treat over 400 patients with vestibular schwannomas, delivering 18–21 Gy in 3 sessions separated by 24 hours. To date, only one patient within this cohort has shown evidence of tumor progression. No treatment-related trigeminal or facial nerve dysfunction was observed. No cases of total hearing loss were reported after the treatment, while 74% of patients with either Gardner-Robertson grade I or II hearing kept this level of function following treatment (mean follow-up was 36 months) Citation[36].
Trigeminal neuralgia
The unique technical attributes of robotic radiosurgery make it an important new tool for managing trigeminal neuralgia (TN). In comparison to GammaKnife radiosurgical rhizotomy, a longer segment of nerve can be irradiated non-isocentrically with robotic radiosurgery, which results in a conformal yet relatively homogeneous dose distribution. A rapid onset of pain relief was first reported after CyberKnife rhizotomy among patients with idiopathic TN by Romanelli et al. Citation[38]. A more recent multi-institutional study confirmed these results in a larger cohort of 41 patients Citation[39]. In this later study, a prescribed dose of 60–70 Gy was delivered to the retrogasserian region of the trigeminal nerve during a single session. The median latency with which pain relief was observed was only 7 days. Initial pain control was ranked as excellent in 36 patients (87.8%). Only three patients (7.3%) reported no pain relief, while two other patients experienced a moderate reduction of pain. Long-lasting pain relief was found in 32 (78%) of 41 patients. A significant number of patients developed numbness after the treatment (51.2%), but this incidence is much less than with traditional destructive procedures. A strict relation between the length of trigeminal nerve treated and onset of numbness was found, which has led to a gradual dose de-escalation.
The published data from CyberKnife rhizotomy, albeit still preliminary, suggests that, compared to other radiosurgery devices, robotic radiosurgery can induce higher rates of initial pain control and hypoesthesia in association with a shorter latency of pain relief. The early onset of pain relief may reflect the improved treatment conformality and dose homogeneity that is possible with the non-isocentric robotic beam targeting and the absence of a rigid frame blocking beam penetration at the level of the skull base. Further experience is now being accumulated with only 6 mm of nerve being irradiated to a marginal dose of 60 Gy in the hope of reducing the rate of hypoesthesia.
Treatment of spinal tumors with robotic radiosurgery
There have been several reports of spinal radiosurgery over the last 10 years. The first of these involved the surgical attachment to the patient of a stereotactic frame specifically designed to immobilize and localize within the spine Citation[40]. More recently, several institutions have reported their experience with intensity-modulated radiation therapy for spinal lesions using a standard linear accelerator and non-invasive external immobilization, a method originally described by Lax and colleagues to treat extracranial lesions Citation[41–47].
Robotic radiosurgery provides an important new tool for treating a broad spectrum of spinal lesions with the same measure of accuracy and conformality that characterizes standard frame-based intracranial radiosurgery. An evaluation of the clinically relevant accuracy for treating spinal lesions has been reported by Yu et al. Citation[32]. These measurements were performed at three different CyberKnife facilities using head and torso phantoms loaded with packs of radiochromic film. The displacement of the dose contours from the treatment planning was reported to be submillimetric. The average treatment delivery precision was found to be 0.3 ± 0.1 mm. Fiducial tracking error was less than 0.3 mm for radial translations up to 14 mm and less than 0.7 mm for rotations up to 4.5° Citation[32].
In the first clinical report of spinal radiosurgery with robotic methods, Ryu et al. demonstrated the safety and short-term efficacy of this treatment for a variety of neoplastic and vascular lesions Citation[48]. Surgical implantation of fiducials to track the spinal lesion was performed with no accidents or adverse side-effects related to the implantation of fiducials. Hypofractionation using 2–5 fractions was typically employed to minimize the risk of radiation injury to the spinal cord. More recently, the safety and efficacy of single-fraction spinal robotic radiosurgery was also reported by Gerszten et al. Citation[49]. A cohort of 125 patients with spinal lesions were treated in a single session, using a mean tumor dose of 14 Gy as prescribed to the 80% isodose. Most of these lesions were metastatic (108 cases), and 59% of them had been treated previously with conventional irradiation. No acute radiation toxicity or new neurological deficits occurred during the median 18-month follow-up. Because the spinal cord is fragile and poorly vascularized, it has a unique proclivity to radiation injury, and because many of these patients had undergone previous conventional irradiation, the findings in the study of Gerszten et al. are particularly impressive. This clinical safety profile reinforces the notion that robotic radiosurgery indeed provides submillimetric precision.
Gerszten et al. also demonstrated improved pain scores and quality of life in patients with pain stemming from vertebral body metastases: axial and radicular pain improved in 74 of 79 patients. The same group recently showed that treatment of metastatic tumors of the spine can be combined with kyphoplasty to repair pathological compression fractures Citation[50]. This is a new treatment paradigm which, by combining minimally invasive procedures, lessens the risk of surgical complications, lowers cost and improves quality of life. Even more integrated approaches may emerge in the future and change the classic surgical management of spinal and body lesions.
Robotic treatment of extracranial lesions
Treatment of extracranial lesions with precision irradiation from conventional LINACs and non-invasive immobilization has been described by several centers during the last five years Citation[44–47], Citation[51–53]. A review of the stereotactic radiotherapy experience in treating liver malignancies has been recently provided by Fuss and Thomas Citation[54]. Image-guided robotic radiosurgery adds a powerful and versatile tool to the field. The method for tracking target motion described above has now been applied in multiple centers worldwide to ablate lung tumors. As for the treatment of spinal lesions, image guidance requires the implantation of fiducials in the vicinity of the lesion so that real-time tracking can be performed Citation[16], Citation[17]. Gold seeds are preferred since they are inert, readily inserted, and easy to image.
Robotic treatment of pulmonary lesions was studied in 23 patients with biopsy-proven lung tumors (15 primary and 8 metastatic lesions) in a Phase I trial by Whyte et al. Citation[55]. After CT-guided percutaneous fiducial placement, the patients received 15 Gy in a single fraction. The treatment was well-tolerated, despite some complications related to the implantation of fiducials. Radiographic progression was found in only 2 patients in a follow-up period ranging from 1 to 26 months (mean: 7 months). Unfortunately, long-term follow-up has not yet been reported.
Treatment of pancreatic adenocarcinomas with CyberKnife radiosurgery was first reported by Romanelli et al. Citation[56]. Twelve patients with pancreatic adenocarcinomas were treated with 15, 20 or 25 Gy delivered in a single fraction. The treatment was well-tolerated with no acute significant toxicity. Decreased values of the pancreatic cancer marker CA 19–9 were found in the majority of the patients following the treatment. All patients with pain prior to treatment experienced an improvement, a phenomenon similar to that experienced by patients with vertebral body metastatic pain following radiosurgery Citation[49].
These preliminary results have been confirmed by further reports from Koong et al. Citation[57], Citation[58]. They found that local control of tumor progression was achieved with a single dose of 25 Gy. This treatment was well-tolerated without serious gastrointestinal toxicity. In a further report, the same group investigated the combination of a 25-Gy radiosurgical boost with 5-fluorouracil (5-FU) chemotherapy and IMRT. Grade 3 toxicity was experienced by 2 patients. Local control was achieved in 15 of 16 patients, but the combined therapy did not affect overall survival (the patients died from distant progression). It is of interest that greater toxicity was reported following the combination of radiosurgical boost with IMRT, with no better survival than with radiosurgery alone. This finding has encouraged the authors to replace IMRT plus radiosurgery with radiosurgery alone in order to achieve local growth control, while more aggressive chemotherapy protocols have been implemented to improve the control of metastatic disease. While these preliminary results are uniformly encouraging, much more evidence is needed to prove that robotic radiosurgery can substantially impact the clinical outcome for a range of extracranial lesions.
Near-future directions
Although tumors that move throughout respiration are now routinely tracked and treated using gold fiducials placed in proximity to the lesion, fiducial implantion is invasive, albeit minimally so, and this procedure can be technically demanding. Recent research suggests that motion compensation without implanted gold markers may be clinically practical Citation[30]. Such technology would considerably simplify treatment protocols. Using 4D imaging and image registration, several CT scans are acquired before treatment using different stages of the breathing cycle for each. A large number of digitally reconstructed radiographs (DRRs) are computed from each CT volume, incorporating the potential for a broad range of small patient movements into all the synthetic images. During treatment, live X-ray images are compared to all DRRs for the different respiratory states. During the process of performing 2D-2D registrations, the best matching DRR is identified and the current state in the breathing cycle, as well as the location of the tumor, can be determined.
With motion compensation, the treatment beams move synchronously to the target, and their effect on the target region will be approximately as planned. However, some tumors may deform or rotate, and tissue surrounding the tumor might exhibit a different motion pattern than the tumor itself. Obtaining information on the relative motion of organs with respect to the treatment beams can be incorporated into the planning process, thus further improving the overall precision of the treatment.
Current robotic systems use cylindrical collimators. Multi-leaf collimators in combination with robotic systems could still further reduce treatment time and improve treatment conformality. As will be explained below, this same objective might also be achieved by dramatically increasing LINAC output and thereby not having to sacrifice the deterioration in beam penumbra that comes with multi-leaf collimation.
Far-future directions
Most often, idle speculation about remote future technology development is just that, and is therefore destined to remain forever within the domain of science fiction. However, sometimes this exercise can be useful in distilling the essence of an important concept. With the latter objective/hope in mind, it is worth musing upon what the ultimate future embodiment of image-guided robotic radiosurgery might look like.
As medical imaging grows ever more able to resolve ever smaller tumors, many of which are invisible to contemporary scanning modalities, perhaps in conjuction with other diagnostic tools such as gene array chips, minimally invasive lesion ablation will be increasingly required. Given its high rate of efficacy, the spatially driven biological specificity of radiation, and the relatively low incidence of complications, radiosurgery is uniquely positioned to fill this need. It is not hard to envision a world where radiosurgery is routinely and perhaps even repeatedly used in many/most cancer patients to treat multiple small or even microscopic lesions. However, to reach such a point of ubiquitous acceptance, especially with metastatic cancer, radiosurgical technology will need to evolve further. The focus of such an ongoing progress must involve hardware and software improvements, in order to obtain greater system efficiency and spatial accuracy while at the same time making the process of treating soft tissue targets ever less invasive. Increased LINAC output, improved image-guidance tools and use of unexplored radiant energy sources may all play a role in the development of an even more advanced form of tumor ablation – microradiosurgery. This will be discussed below.
Increased LINAC output
There are several logical technological developments that have the potential to enhance the efficiency of image-guided radiosurgery. The first of these involves ever greater LINAC output. Over the past decade, the radiation output of the CyberKnife has increased approximately 300%, from 200 to 600 rad/min. Although the engineering needed to increase this further is not trivial, especially given the associated challenges of precisely manipulating the weight of the necessary additional tungsten radiation shielding, it is worth speculating about the potential clinical opportunities that could be enabled with ever greater LINAC output, perhaps even as “hot” as 4-5,000 rad/min. As machine output goes up, the time penalty decreases, thereby creating two clinical opportunites: 1) to further improve radiosurgical conformality for complex-shaped lesions by working with ever finer pencil beams, which secondarily also have less penumbra; and, even more importantly, 2) to treat ever larger numbers of metastases during a single session. The latter situation is based on the growing acceptance within the oncologic community of managing “oligo-metastatic” cancer as a local disease. In other words, in many patients with metastastic cancer, long-term palliation, and occasionally even cure, is possible through aggressive local therapies which primarily involve either surgical resection or local irradiation. By first principles, radiosurgery combines the virtues of both treatments and, when possible, is increasingly the preferred modality for treating a metastasis.
There is no formal definition for determining how many lesions can be present and still classify a patient's cancer as oligo-metastatic: This is in contrast to a cancer being even more widespread and thereby classified as a “systemic” disease, one that requires primarily a chemotherapeutic approach. However, all of this classification is in large part an artificial construct, driven somewhat by the diminishing rate of return (cure or prolonged remission) that can be expected as the number of metastases increases; the greater the number, the harder it is to justify, often for reasons of cost, a primarily localized therapy. Nevertheless, one can readily envision an ever more efficient process of large-scale tumor ablation based on fiducial-less CyberKnife radiosurgery that might also be combined with automated tumor detection from CT, PET and MRI. The idea of treating several dozen lesions throughout the body of selected cancer patients with radiosurgery would be thoroughly practical if the overall procedure were not too long. It is this latter point that emphasizes the importance of working with sufficient LINAC output.
Fiducial-less targeting
A glaring deficit in the current technique for image-guided radiosurgical ablation is the need for implanted fiducials in or around soft tissue targets; the treatment of most such lesions belies the very “non-invasive” essence of radiosurgery. Although the preliminary research alluded to above holds out the potential of performing some fiducial-less radiosurgery in the lung, it is clear that a more universal solution for soft tissue targeting, one that will also work in the abdomen and pelvis, is highly desirable. Rapid improvements in the flat panel X-ray detectors used with image guidance, both in terms of resolution and sensitivity, when combined with even more complex algorithms, may eventually permit some targets within the abdomen and pelvis to be localized. However, it would seem likely that additional, and perhaps even quite radical, changes to the real-time imaging system of the CyberKnife will be needed to enable universal “whole body” image guidance. One could envision CT-like imaging serving a role in targeting. Regardless, fiducial-less soft tissue radiosurgery will be vital if large-scale tumor ablation for many patients with “oligo-metastatic” cancer (as described above) is to become a reality.
Is there a place for new energy sources?
Radiosurgery is not defined by any specific radiation source. Co60, LINAC-generated X-ray photons and heavy particles (protons, helium or carbon ions) represent the range of radiosurgical beams that are currently in use. We believe that there are only the most modest differences in clinical advantage between these technologies, some of which are extremely expensive. It is interesting to speculate whether or not there might be some even better therapeutic ionizing energy source for radiosurgery that has yet to be investigated clinically. Orthovoltage microbeam radiation may be such an entity. Microbeam irradiation disperses minimally as it transits the body and appears to be associated with a unique form of near-complete repair in normal tissues Citation[59]. It is possible to use microbeams ranging from 27 to 270 µm with doses as high as 2500 Gy causing a biological effect (in essence thorough necrosis) only along the path of penetration Citation[59]. Widespread clinical diffusion of microbeam radiosurgery is severely limited by the fact that a synchrotron source is required. However, if the currently huge expense of generating microbeam radiation could be lessened by 2–3 orders of magnitude, some unique clinical opportunities might develop in conjunction with ultra-precise image guided delivery (the targeting accuracy will need to be above and beyond the highest radiosurgical standards currently available).
Microbeam radiosurgery has the potential to virtually cut tissue like a knife without compromising the biologic integrity of the normal tissues along the beam trajectory. The penetration of microbeams can be modified in relation to their energy. Using low energy ranges (around 120 kv), this ability to create radiosurgical cuts through the tissue may be restricted to the cortex, opening unexpected new ways to treat brain disease. For example, epileptogenic foci in eloquent cortex could be treated with microradiosurgical cortical transections. The interruption of the horizontal pathways of seizure spread could be achieved without damage to the vertical columns of the cortex, thereby avoiding catastrophic neurological complications. Consequently, low-energy microbeams have theoretical promise for ablation of neocortical epileptogenic foci. A much better understanding of the biology underlying microbeam irradiation will be required, along with an entirely new cost-effective technology for generating such X-rays, before microbeams might become a clinically practical treatment option. Despite such huge current barriers, it is worth keeping in mind the substantial theoretical clinical advantages of microbeam radiosurgery.
Microimaging
Is it possible to envision a future where microscopic malignant lesions are detected early by increasingly powerful neuroimaging tools? Radiosurgical microablation of such lesions could then become an important treatment option. Certainly, the ability to micro-scan tissues using high-power MR imaging or microtomography can provide a powerful tool to map clusters of metastatic cells spread throughout the body. There is growing evidence that micro CT, micro MR, micro PET, micro SPECT and optical imaging can provide anatomic and functional localization at a microscopic level. For instance, the spatial resolution of micro CT is currently less than 50 µm and can be used to obtain real-time 3D attenuation maps of anatomic structures in experimental animals Citation[60], Citation[61]. Micro-CT has recently been shown to provide 3D resolution of basic micro-architectural structures, such as lung alveoli, in small animals Citation[62]. Micro CT can also be used to dynamically track the respiratory and cardiac cycles Citation[62], offering the possibility of performing image guidance at a microscopic level. Modern micro PET scanning provides high sensitivity in the analysis of physiologic and pathologic states in association with a volumetric spatial discrimination of approximately 1 mm3 Citation[63], Citation[64]. Molecular probes can be used to further enhance the sensitivity and resolution of the discussed imaging modalities. Even gene expression can now be imaged through the detection of marker proteins (for a review, see reference 65).
Overall, the technological advances made during recent years are impressive, and a detailed discussion would be beyond the scope of this article. However, it appears that within a few years we could clinically use microimaging modalities to study microscopic features of normal and pathological tissues, investigate the presence of dynamic phenomena such as gene expression in malignant cells or apoptosis, and obtain microscopic 3D target reconstruction. It is therefore possible to envision microimage guidance as an attainable goal in the future.
Microradiosurgery and molecular radiosurgery
Aside from the huge side effects, conventional radiation therapy and chemotherapy for metastatic disease are plagued by their limited efficacy. In most cases, once the load of malignant cells exceeds the limits of the immune system of a given individual, an effective fight can no longer be waged and the patient is doomed. How could this outlook be changed by the radioablation of microscopic disease? Microradiosurgery certainly is an attractive option, insofar as disseminated microscopic disease can be effectively mapped and a radiosurgical tool is available that can precisely deliver beams of submillimetric width within a reasonable timeframe. We have discussed above the possibility of imaging microscopic or macromolecular targets and using microbeams to cause extremely selective tissue damage. The combination of these two technologies is a promising way to perform radioablation of microscopic lesions or, even further, to deliver focused radiation to specific macromolecular targets. We believe that this ambitious goal is still far in the future but is also eminently realistic.
Conclusions
Radiosurgery is experiencing a technological revolution. The recent introduction of image guidance and robotic delivery have broadened the applications of radiosurgery to the entire body. Improvements in neuroimaging and computer technology, plus possible use of new radiation sources, will probably change still further the way we treat tumors, with a shift of the treatment paradigms toward more localized, non-invasive technology such as radiosurgery. Implementation of radiosurgical ablation on a microscopic or even molecular level is a likely consequence of this foreseeable trend over the coming decades.
References
- Leksell L. The stereotaxic method and radiosurgery of the brain. Acta Chir Scand 1951; 102: 316–319
- Ulfarsson E, Lindquist C, Roberts M, Rahn T, Lindquist M, Thoren M, Lippitz B. Gamma knife radiosurgery for craniopharyngiomas: long term results in the first Swedish patients. J Neurosurg 2002; 97(S5)613–622
- Muacevic A, Kreth F W, Horstmann G A, Schmid-Elsaesser R, Wowra B, Steiger H J, Reulen H J. Surgery and radiotherapy compared with gamma knife radiosurgery in the treatment of solitary cerebral metastases of small diameter. J Neurosurg 1999; 91: 35–43
- Winston K R, Lutz W. Linear accelerator as a neurosurgical tool for stereotactic radiosurgery. Neurosurgery 1988; 22: 454–464
- Wong J. Methods to manage respiratory motion in radiation treatment. J R Palta, M T Rockwell. Intensity-Modulated Radiation Therapy. Medical Physics Publishing. 2003; 663–702
- Langen K M, Jones D TL. Organ motion and its management. Int J Radiat Oncol Biol Phys 2001; 50: 265–278
- Berson A M, Emery R, Rodriguez L, Richards G M, Ng T, Sanghavi S, Barsa J. Clinical experience using respiratory gated radiation therapy: comparison of free-breathing and breath-hold techniques. Int J Radiat Oncol Biol Phys 2004; 60: 419–426
- Kimura T, Hirokawa Y, Murakami Y, Tsujimura M, Nakashima T, Ohno Y, Kenjo M, Kaneyasu Y, Wadasaki K, Ito K. Reproducibility of organ position using voluntary breathhold method with spirometer for extracranial stereotactic radiotherapy. Int J Radiat Oncol Biol Phys 2004; 60: 1307–1313
- Onishi H, Kuriyama K, Komiyama T, Tanaka S, Sano N, Aikawa Y, Tateda Y, Araki T, Ikenaga S, Uematsu M. A new irradiation system for lung cancer combining linear accelerator, computed tomography, patient self-breathholding, and patient-directed beam-control without respiratory monitoring devices. Int J Radiat Oncol Biol Phys 2003; 56: 14–20
- Cheung P CF, Sixel K E, Tirona R, Ung Y C. Reproducibility of lung tumor position and reduction of lung mass within the planning target volume using active breathing control (ABC). Int J Radiat Oncol Biol Phys 2003; 57: 1437–1442
- Brewer J, Betke M, Gierga D P, Chen G TY (2004) Realtime 4D tumor tracking and modeling from internal and external fiducials in fluoroscopy. Proceedings of 7th International Conference on Medical Image Computing and Computer-Assisted Intervention (MICCAI 2004), Saint MaloFrance, September, 2004, C Barillot, D R Haynor, P Hellier. Springer, Berlin, 594–601, Part II. Lecture Notes in Computer Science Vol 3217
- Hoisak J DP, Sixel K E, Tirona R, Cheung P CF, Pignol J P. Correlation of lung tumor motion with external surrogate indicators of respiration. Int J Radiat Oncol Biol Phys 2004; 60: 1298–1306
- Tsunashima Y, Sakae T, Shioyama Y, Kagei K, Terunuma T, Nohtomi A, Akine Y. Correlation between the respiratory waveform measured using a respiratory sensor and 3D tumor motion in gated radiotherapy. Int J Radiat Oncol Biol Phys 2004; 60: 951–958
- Gierga D P, Chen G TY, Kung J H, Betke M, Lombardi J, Willett C G. Quantification of respiration-induced abdominal tumor motion and its impact on IMRT dose distributions. Int J Radiat Oncol Biol Phys 2004; 58: 1584–1595
- Dieterich S, Tang J, Rodgers J, Cleary K (2003) Skin respiratory motion tracking for stereotactic radiosurgery using the CyberKnife. Proceedings of the 17th International Congress and Exhibition (CARS 2003), LondonUK, June, 2003, H U Lemke, K Inamura, M W Vannier, A G Farman, K Doi, J HC Reiber. Elsevier, Amsterdam, 130–136, Computer Assisted Radiology and Surgery
- Schweikard A, Glosser G, Bodduluri M, Murphy M J, Adler J R. Robotic motion compensation for respiratory movement during radiosurgery. Comput Aided Surg 2000; 5: 263–277
- Schweikard A, Shiomi H, Adler J R. Respiration tracking in radiosurgery. Med Phys 2004; 31: 2738–2741
- Kaus M R, Netsch T, Kabus S, Pekar V, McNutt T, Fischer B (2004) Estimation of organ motion from 4D CT for 4D radiation therapy planning of lung cancer. Proceedings of 7th International Conference on Medical Image Computing and Computer-Assisted Intervention (MICCAI 2004), Saint MaloFrance, September, 2004, C Barillot, D R Haynor, P Hellier. Springer, Berlin, 1017–1024, Part II. Lecture Notes in Computer Science Vol 3217
- Mageras G S, Pevsner A, Yorke E D, Rosenzweig K E, Ford E C, Hertanto A, Larson S M, Lovelock D M, Erdi Y E, Nehmeh S A, Humm J L, Ling C C. Measurement of lung tumor motion using respiration-correlated CT. Int J Radiation Oncology Biol Phys 2004; 60: 933–941
- Plathow C, Ley S, Fink C, Puderbach M, Hosch W, Schmähl A, Debus J, Kauczor H U. Analysis of intrathoracic tumor mobility during whole breathing cycle by dynamic MRI. Int J Radiat Oncol Biol Phys 2004; 59: 952–959
- Shirato H, Seppenwoolde Y, Kitamura K, Onimura R, Shimizu S. Intrafractional tumor motion: Lung and liver. Semin Radiat Oncol 2004; 14: 10–18
- Sharp G C, Jiang S B, Shimizu S, Shirato H. Prediction of respiratory tumour motion for real-time image-guided radiotherapy. Phys Med Biol 2004; 49: 425–440
- Vedam S S, Keall P J, Docef A, Todor D A, Kini V R, Mohan R. Predicting respiratory motion for four-dimensional radiotherapy. Med Phys 2004; 31: 2274–2283
- Webb S. Conformal intensity-modulated radiotherapy (IMRT) delivered by robotic linac – testing IMRT to the limit?. Phys Med Biol 1999; 44: 1639–1654
- Webb S. Conformal intensity-modulated radiotherapy (IMRT) delivered by robotic linac – conformality versus efficiency of dose delivery. Phys Med Biol 2000; 45: 1715–1730
- Schweikard A, Bodduluri A, Adler J R. Planning for camera-guided robotic radiosurgery. IEEE Trans Robotics Automation 1998; 14: 951–962
- Adler J R, Murphy M J, Chang S D, Hancock S L. Image-guided robotic radiosurgery. Neurosurgery 1999; 44: 1299–1307
- Li J G, Xing L. Inverse planning incorporating organ motion. Med Phys 2000; 27: 1573–1578
- Unkelbach J, Oelfke U. Incorporating organ movements in inverse planning: assessing dose uncertainties by Bayesian inference. Phys Med Biol 2005; 50: 121–139
- Schweikard A, Shiomi H, Adler J R. Respiration tracking in radiosurgery without fiducials. IJMRCAS 2005; 1: 19–27
- Chang S D, Main W, Martin D P, Gibbs I C, Heilbrun M P. An analysis of the accuracy of the CyberKnife: a robotic frameless stereotactic radiosurgical system. Neurosurgery 2003; 52: 140–147
- Yu C, Main W, Taylor D, Kuduvalli G, Apuzzo M L, Adler J R. An anthropomorphic phantom study of the accuracy of CyberKnife spinal radiosurgery. Neurosurgery 2004; 55: 1138–1149
- Simonova G, Novotny J, Jr, Liscak R. Low-grade gliomas treated by fractionated gamma knife surgery. J Neurosurg 2005; 102(S)19–24
- Mehta V K, Lee Q T, Chang S D, Cherney S, Adler J R. Image guided stereotactic radiosurgery for lesions in proximity to the anterior visual pathways: A preliminary report. Technol Cancer Res Treat 2002; 1: 173–180
- Pham C J, Chang S D, Gibbs I C, Jones P, Heilbrun M P, Adler J R. Preliminary visual field preservation after staged CyberKnife radiosurgery for perioptic lesions. Neurosurgery 2004; 54: 799–812
- Chang S D, Gibbs I C, Sakamoto G T, Lee E, Oyelese A, Adler J R. Staged stereotactic irradiation for acoustic neuroma. Neurosurgery 2005; 56: 1254–1263
- Ishihara H, Saito K, Nishizaki T, Kajiwara K, Nomura S, Yoshikawa K, Harada K, Suzuki M. CyberKnife radiosurgery for vestibular schwannoma. Minim Invasive Neurosurg 2004; 47: 290–293
- Romanelli P, Heit G, Chang S D, Martin D, Pham C, Adler J R. CyberKnife radiosurgery for trigeminal neuralgia. Stereotact Funct Neurosurg 2003; 81: 105–109
- Lim M, Villavicencio A T, Burneikiene S, Chang S D, Romanelli P, McNeely L, McIntyre M, Thramann J J, Adler J R. CyberKnife radiosurgery for idiopathic trigeminal neuralgia. Neurosurg Focus 2005; 18(5)E9
- Hamilton A J, Lulu B A, Fosmire H, Stea B, Cassady J R. Preliminary clinical experience with linear accelerator-based spinal stereotactic radiosurgery. Neurosurgery 1995; 36: 311–319
- Lax I, Blomgren H, Naslund I, Svanstrom R. Stereotactic radiotherapy of malignancies in the abdomen. Methodological aspects. Acta Oncol 1994; 33: 677–683
- Blomgren H, Lax I, Goranson H, Kraepelien T, Nilsson B, Naslund I, Svanstrom R, Tilikidis A. Radiosurgery for tumors in the body: clinical experience using a new method. J Radiosurg 1998; 1: 63–74
- Blomgren H, Lax I, Naslund I, Svanstrom R. Stereotactic high dose fraction radiation therapy of extracranial tumors using an accelerator. Clinical experience of the first thirty-one patients. Acta Oncol 1995; 34: 861–870
- Bilsky M H, Yamada Y, Yenice K M, Lovelock M, Hunt M, Gutin P H, Leibel S A. Intensity-modulated stereotactic radiotherapy of paraspinal tumors: A preliminary report. Neurosurgy 2004; 54: 823–831
- Ryu S, Yin F F, Rock J, Zhu J, Chu A, Kagan E, Rogers L, Ajlouni M, Rosenblum M, Kim J H. Image-guided and intensity-modulated radiosurgery for patients with spinal metastasis. Cancer 2003; 97: 2013–2018
- Shiu A S, Chang E L, Ye J S, Lii M, Rhines L D, Mendel E, Weinberg J, Singh S, Maor M H, Mohan R, Cox J D. Near simultaneous computed tomography image-guided stereotactic spinal radiotherapy: An emerging paradigm for achieving true stereotaxy. Int J Radiat Oncol Biol Phys 2003; 57: 605–613
- Yenice K M, Lovelock D M, Hunt M A, Lutz W R, Fournier-Bidoz N, Hua C H, Yamada J, Bilsky M, Lee H, Pfaff K, Spirou S V, Amols H I. CT image-guided intensity-modulated therapy for paraspinal tumors using stereotactic immobilization. Int J Radiat Oncol Biol Phys 2003; 55: 583–593
- Ryu S I, Chang S D, Kim D H, Murphy M J, Le Q T, Martin D P, Adler J R. Image-guided hypo-fractionated stereotactic radiosurgery to spinal lesions. Neurosurgery 2001; 49: 838–846
- Gerszten P C, Ozhasoglu C, Burton S A, Vogel W, Atkins B, Kalnicki S, Welch W C. CyberKnife frameless stereotactic radiosurgery for spinal lesions: Clinical experience in 125 Cases. Neurosurgery 2004; 55: 89–99
- Gerszten P C, Germanwala A, Burton S A, Welch W C, Ozhasoglu C, Vogel W J. Combination kyphoplasty and spinal radiosurgery: A new treatment paradigm for pathological fractures. Neurosurg Focus 2005; 18(3)E8
- Herfarth K K, Debus J, Lohr F, Bahner M L, Rhein B, Fritz P, Hoss A, Schlegel W, Wannenmacher M F. Stereotactic single-dose radiation therapy of liver tumors: Results of a Phase I/II trial. J Clin Oncol 2001; 19: 164–170
- Timmerman R, Papiez L, McGarry R, Likes L, DesRosiers C, Frost S, Williams M. Extracranial stereotactic radioablation: Results of a Phase I study in medically inoperable stage I non-small cell lung cancer. Chest 2003; 124: 1946–1955
- Uematsu M, Shioda A, Taira H, Wong J, Hama Y, Kusano S. Computed tomography (CT)-guided stereotactic radiation therapy (SRT) for stage I non-small cell lung cancer (NSCLC): 8-year results of 50 initial patients. Int J Radiat Oncol Biol Phys 2003; 57(S2)S281
- Fuss M, Thomas C R. Stereotactic body radiation therapy: An ablative treatment option for primary and secondary liver tumors. Ann Surgical Oncol 2004; 11: 130–138
- Whyte R I, Crownover R, Murphy M J, Martin D P, Rice T W, DeCamp M M, Rodebaugh R, Weinhous M S, Le Q T. Stereotactic radiosurgery for lung tumors: preliminary report of a Phase I trial. Ann Thorac Surg 2003; 75: 1097–1101
- Romanelli P, Chang S D, Koong A, Adler J R. Extracranial radiosurgery using the CyberKnife. Techniques in Neurosurgery 2003; 9: 226–231
- Koong A C, Le Q T, Ho A, Fong B, Fisher G, Cho C, Ford J, Poen J, Gibbs I C, Mehta V K, Kee S, Trueblood W, Yang G, Bastidas J A. Phase I study of stereotactic radiosurgery in patients with locally advanced pancreatic cancer. Int J Radiat Oncol Bio Phys 2004; 58: 1017–1021
- Koong A C, Christofferson E, Le Q T, Goodman K A, Ho A, Kuo T, Ford J M, Fisher G A, Greco R, Norton J, Yang G P. Phase II study to assess the efficacy of conventionally fractionated radiotherapy followed by a stereotactic radiosurgery boost in patients with locally advanced pancreatic cancer. Int J Radiat Oncol Biol Phys 2005; 63: 320–323
- Dilmanian F A, Qu Y, Liu S, Cool C D, Gilbert J, Hainfeld J F, Kruse C A, Laterra J, Lenihan D, Nawrocki M M, Pappas G, Sze C I, Yuasa T, Zhong N, Zhong Z, McDonald J W. X-ray microbeams: Tumor therapy and central nervous system research. Nucl Instrum Methods Phys Res 2005; 548: 30–37
- Beckmann F, Heise K, Kolsch B, Bonse U, Rajewsky M F, Bartscher M, Biermann T. Three-dimensional imaging of nerve tissue by X-ray phase-contrast microtomography. Biophysical J 1999; 76: 98–102
- Brasse D, Humbert B, Mathelin C, Rio M C, Guyonnet J L. Towards an inline reconstruction architecture for micro-CT systems. Phys Med Biol 2005; 50: 5799–5811
- Ritman E L. Micro-computed tomography of the lungs and pulmonary-vascular system. Proc Am Thorac Soc 2005; 2: 477–480
- Stickel J R, Cherry S R. High-resolution PET detector design: modelling components of intrinsic spatial resolution. Phys Med Biol 2005; 50: 179–195
- Chatziioannou A F. Instrumentation for molecular imaging in preclinical research. Micro-PET and micro-SPECT. Proc Am Thorac Soc 2005; 2: 533–536
- Kim E E. Targeted molecular imaging. Korean J Radiol 2003; 4: 201–210