Abstract
Objective: To develop and evaluate the feasibility and reliability of an alternative three-dimensional (3D) measurement system capable of characterizing tunnel position and orientation in ACL reconstructed knees.
Methods: We developed a surgically oriented 3D measurement system for characterizing femoral and tibial drill tunnels from ACL reconstructions. This is accomplished by simulating the positioning of the drill bit originally used to create the tunnels within the bone, which allows for angular and spatial descriptions along defined axes that are established with respect to previously described anatomic landmarks and radiographic views. Computer-generated digital phantoms composed of simplified geometries were used to verify proper calculation of angular and spatial measurements. We also evaluated the inter-observer reliability of the measurements using 10 surfaces generated from cadaveric knees in which ACL tunnels were drilled. The reliability of the measurements was evaluated by intraclass correlation coefficients.
Results: The digital phantom evaluation verified the measurement methods by computing angular and spatial values that matched the known values in all cases. The intraclass correlation coefficient was calculated for four users and was found to range from 0.95 to 0.99 for the femoral and tibial measurements, demonstrating near-perfect agreement.
Conclusions: The characterization of ACL tunnels has historically concentrated on two-dimensional (2D) measurements; however, it can be difficult to define ACL tunnel placement using 2D methods. We have presented novel techniques for defining graft tunnel placement from 3D surface representations of the ACL reconstructed knee. These measurements provide exact tunnel location spatially and along axes that offer the potential to comparatively analyze ACL reconstructions post-operatively using advanced imaging. These methods are reliable, and have been demonstrated to be applicable to multiple single-bundle techniques for ACL reconstruction.
Introduction
Disruption of the anterior cruciate ligament (ACL) is a common injury of the human knee, especially in athletes. It has been estimated that nearly 175,000 ACL reconstructions are performed each year, with an estimated cost of up to $1 billion Citation[1–4]. Despite generally good outcomes after ACL surgery, research continues into ways of preventing post-reconstruction problems such as the need for ACL revision reconstruction or the development of osteoarthritis. One of several potential reasons for adverse outcomes following ACL reconstruction is poor tunnel placement Citation[4], Citation[5].
Biomedical researchers have developed image processing and modeling software packages to address various issues in imaging research. In addition to the commercial software options, the BRAINS2 open-source software package is a set of tools used to process and segment three-dimensional (3D) images for the purpose of surface generation and measurement Citation[6]. BRAINS2 was originally designed for brain researchers, but others have used it to validate the accuracy of surface representations of CT images for orthopaedic structures Citation[7]. The manipulation of surface representations for surgical simulations using the National Library of Medicine's open-source Visualization Toolkit (VTK) has also been reported Citation[8], Citation[9]. These open-source tools offer flexibility in addressing a number of imaging-related research questions; here, we have applied them to the evaluation of ACL reconstruction tunnel placement variability.
The characterization of ACL tunnels has largely concentrated on two-dimensional (2D) measurements from standard radiographic views. The European Society of Sports Traumatology Knee Surgery and Arthroscopy (ESSKA) Scientific Workshop on Reconstruction of the Anterior and Posterior Cruciate Ligaments has provided guidelines for describing tunnel locations on the distal femur and proximal tibia Citation[10]. Two-dimensional tunnel evaluation studies using plain films have been performed using these guidelines and variations on them Citation[11–15]. However, it can be difficult to define actual ACL tunnel placement using 2D methods Citation[16]. Previous work has also demonstrated a wide range of inter-rater agreement based on post-ACL radiographs Citation[17].
With the advent of CT technologies, several studies have extrapolated the 2D measurements to CT slices Citation[16], Citation[18], Citation[19]. Surface generation from CT images has also been investigated as a method of tunnel placement evaluation Citation[20–24]. Three-dimensional surface-based techniques are desirable due to their ability to visualize not only the bone but also the tunnels through the bone. However, prior systems for evaluating tunnels using CT scans are not readily applied to cadaveric specimens where the malleolar axis is unavailable. In addition, previous tunnel analysis systems Citation[23], Citation[24] have referenced measurements off Blumensaat's line, which the present authors have found to be highly variable and often undulating (). Several prior techniques used to analyze the ACL and ACL reconstruction tunnels rely on descriptive anatomical landmarks such as the distance to articular cartilage and small bony ridges, which may or may not be reproducibly evident on advanced imaging after surgery Citation[14], Citation[23], Citation[25].
Figure 1. Three surface representations of cadaveric femurs demonstrating variability in Blumensaat's line (red) when viewed as sagittal sections looking towards the medial wall of the lateral femoral condyle.
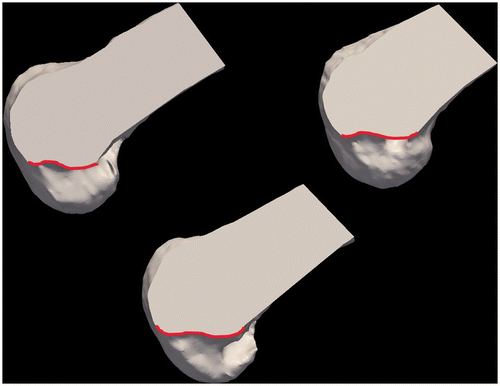
The purpose of this study was to create a reproducible ACL tunnel analysis system that allowed 3D characterization of ACL tunnels, and to evaluate the system's feasibility and reliability. Here, we describe our novel methods and evaluate the inter-observer reliability by calculating the intraclass correlation coefficients across multiple raters. We hypothesize that 3D surface representations of the knee and virtual drill bits can be used reliably to characterize ACL tunnel position and orientation in three dimensions for ACL reconstructed knees.
Materials and methods
Three-dimensional measurement system
A three-dimensional measurement system for characterizing femoral and tibial drill tunnels from ACL reconstruction was developed based on commonly used surgical and radiologic landmarks. This includes the usual “notch view” which most surgeons have during arthroscopy when performing ACL reconstruction. During this procedure, the surgeon flexes the knee at approximately 90° and views the notch approximately along the anatomic axis of the femur. Most ACL tunnel radiographic studies have been based on a perfect lateral radiograph, and our measurement technique takes this into consideration as well. Our ability to characterize the drill tunnels relies on simulating the positioning of the drill bit used to create the tunnels within the bone. Based on positioning virtual drill bits within previously generated 3D surface representations of bone, we are able to describe the drill tunnels with angular and proportional spatial measurements. The following sub-sections describe the methods used to develop a standardized 3D orientation, align the bone surfaces to this orientation, generate and align virtual drill bits, match virtual drill bits to drill tunnel apertures, and automatically measure the ACL tunnels spatially and angularly.
Standardized orientation
To ensure accurate and reproducible measurements in three dimensions, a standardized orientation is required for both the femur and the tibia. We have created separate orientations for the femur and tibia based on radiographic and anatomic views. For the femur, the Z-axis is defined by the femoral diaphysis after compensating for 7° of anatomic valgus (). This orientation is established by rotating the femur such that the intermedullary canal is linearly aligned with the Z-axis. The X-axis is defined by a line through the femoral condyles such that the lateral condyle equally overlaps the medial condyle (), which correlates with a perfect lateral 2D radiograph. This orientation is established by rotating the femur relative to the defined Z-axis such that there is perfect overlap of the femoral condyles. The Y-axis is then mathematically defined by the cross-product of the X- and Z-axes.
Figure 2. The standardized orientations of the femur and tibia. For the femur, the Z-axis is aligned with the femoral diaphysis (A) and the X-axis is defined by a line that allows the medial and lateral condyles to be aligned as in a perfect lateral 2D radiograph (B). (The figure demonstrates a femur with modified transparency to allow visualization of the overlap.) For the tibia, the X-axis is aligned with the medial-lateral slope of the tibial plateau (C) and the Z-axis is aligned such that the posterior-most aspects of the medial and lateral condyles of the tibial plateau are linearly aligned (D).
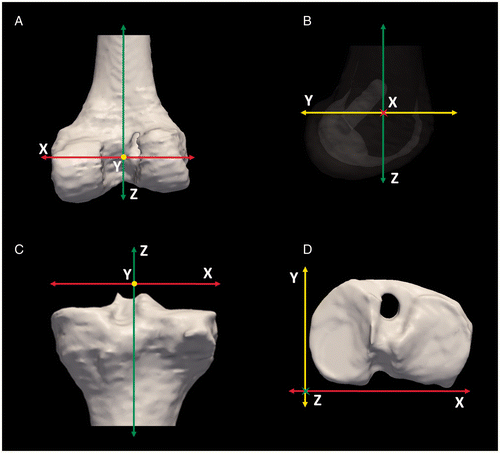
For the tibia, the X-axis is aligned with the medial-lateral slope of the tibial plateau (). This is established by aligning the tibia in an anterior-posterior fashion and then rotating the tibia such that the slope of the tibial plateau from medial to lateral is parallel to the X-axis. The Z-axis is aligned such that the posterior-most aspects of the medial and lateral condyles of the tibial plateau are linearly aligned (). This is accomplished by taking a “bird's-eye view” of the tibial plateau and ensuring that the X-axis is tangent to the posterior-most aspects of the plateau. The Y-axis is then mathematically defined by the cross-product of the X- and Z-axes.
Estimating ACL tunnel position
As an estimate of the drill bit used for the surgical procedure, a cylinder was generated with a diameter equivalent to that of the drill bit. For this study, all procedures were performed using a drill bit with a diameter of 10 mm; thus, a virtual drill bit of the same diameter was created. If another size drill bit were to be employed during the procedure, a different virtual drill bit diameter could be used. The cylinder was assigned a length sufficient to adequately traverse the entire length of the drill tunnel; a drill bit length of 50 mm was satisfactory for all specimens used in this study. To compute the position of the tunnel within the bone, the cylinder was placed within the tunnel () using custom tools Citation[8]. Modifying the transparency of the bone surfaces (as demonstrated in ) allowed for proper positioning of the virtual drill bits using the entire length of the tunnels to align the bits; this avoided tunnel aperture imperfections and deviations of the central axis of the virtual drill bit caused by fixation hardware. Measurements were based on the central axis of the simulated drill bits.
Figure 3. A cylinder with a diameter of 10 mm was used to simulate the drill bit used to create the ACL tunnels. The cylinders were manually aligned with the tunnels of the femur (A) and tibia (B).
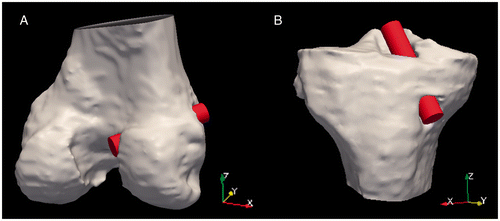
Once the virtual drill bits were aligned with the tunnels, three angles were calculated: α (Equation 1), β (Equation 2), and γ (Equation 3). These angles represent the deviation of the tunnel from the X-, Y- and Z- axes, respectively ( and ).where P1 is the centerpoint of the endcap of the virtual drill bit closest to the knee joint, P2 is the centerpoint of the endcap at the opposite end of the drill bit, Px represents P1 + 1 unit in the X-direction, Py represents P1 + 1 unit in the Y-direction, and Pz represents P1 + 1 unit in the Z-direction.
Figure 4. Tibial tunnel angles (α, β, γ) were measured based on the tunnel centerline's deviation from the surgically oriented coordinate system (A), as demonstrated looking down the Y-axis (B) and the X-axis (C). The points used to calculate the angles are labeled as p1 and p2.
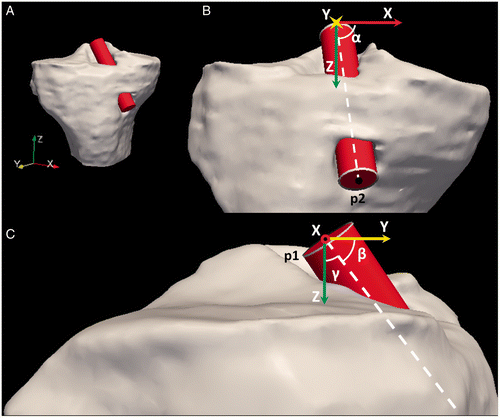
Figure 5. Femoral tunnel angles (α, β, γ) were measured based on the tunnel centerline's deviation from the surgically oriented coordinate system (A), as demonstrated looking down the Y-axis (B) and the X-axis (C). The points used to calculate the angles are labeled as p1 and p2.
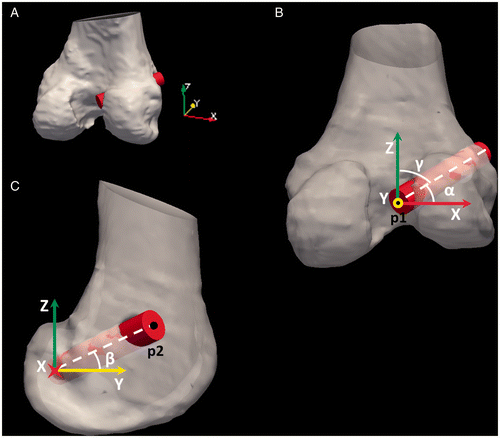
Spatial localization of the ACL drill tunnel apertures allows for correlation of surgical tunnel positioning with respect to proportional measurements of the tibial plateau and the lateral femoral condyle. To estimate the tunnel apertures from the virtual drill bits, the cylindrical surface was cropped at the tunnel aperture using custom software Citation[8]. After the virtual drill bits were cropped to match the tunnel apertures (), two spatial measurements for each bone were calculated: m/M and a/A for the tibia and n/N and c/C for the femur. For the tibia, the position of the aperture centerpoint is measured as a proportion of the distance from the anterior aspect of the tibial plateau (a) with respect to the total tibial depth (A), as shown in , and also as a proportion of the distance from the medial aspect of the tibial plateau (m) with respect to the total width of the tibial plateau (M), as shown in . These proportional measurements are readily calculated by evaluating the spatial extents of the bone surface in the standardized tibia orientation previously described.
Figure 6. The virtual drill bits were cropped to match the tunnel aperture on the femur and tibia. Several views are provided, including the posterior aspect of the distal femur (A), a sagittal view of just the lateral femoral condyle (B), and the tibia (C).
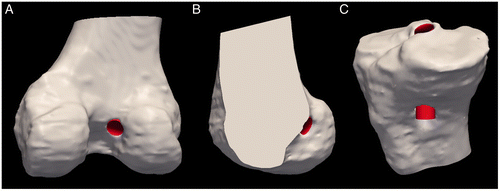
Figure 7. (A) Measurement (a/A) of the tunnel aperture centerpoint based on the anterior-posterior distance of the tibial plateau. (B) Measurement (m/M) of the tunnel aperture centerpoint based on the medial-lateral distance of the tibial plateau.
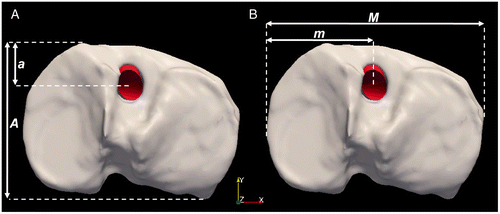
For the femur, the position of the aperture centerpoint is measured as a proportion of the distance from the distal-most aspect of the lateral condyle (c) with respect to the total diameter of the lateral condyle (C), as shown in , and also as the proportion of the distance from the posterior-most aspect of the lateral condyle (n) with respect to the apex of the intercondylar notch (N), as demonstrated in . Custom tools Citation[8] were used to isolate the lateral femoral condyle in the standardized femur orientation; this readily allowed for the described proportional measurements based on evaluating the spatial extents of the isolated lateral femoral condyle.
Evaluation using digital phantoms
To validate the angular and spatial measurements, two digital phantoms were created. Digital phantoms are computer-generated simplified geometries with known spatial and angular dimensions which are used to ensure proper calculations are performed by software. The angular measurements were validated using nine virtual drill bits in different known orientations (aligned with each major axis and between the major axes), as shown in . The spatial measurements were validated using three virtual drill bits (positioned at 25%, 50%, and 75% of the total cube height) encased in a cube and representing drill tunnels passing through the lateral femoral condyle, as shown in . The resulting calculated angular and spatial measurements were compared to the known values used to generate the digital phantoms, and were found to match the known values in all cases.
Evaluation using cadaveric specimens
Ten cadaveric knee joints were collected (4 left and 6 right); each specimen included the full knee joint and a portion of the proximal tibia and distal femur. Prior to scanning, tunnels for a single-bundle ACL reconstruction were performed by a fellowship-trained orthopaedic surgeon on each specimen, using either the medial portal, transtibial, or two-incision technique. The 10 specimens included four medial portal operations, four transtibial operations, and two two-incision operations by six fellowship-trained orthopaedic surgeons. We considered this sub-sample to be representative of the larger dataset as it included multiple surgeons and three common single-bundle ACL reconstruction techniques. A Siemens Sensation 64-slice CT scanner was used to collect 3D voxel datasets of the knee for each specimen (matrix: 1005 × 512; FOV: 261 mm × 133 mm; KVP: 120; current: 128 mA; exposure: 160 mAs) with a 0.26-mm in-plane resolution and a slice thickness of 0.75 mm. Slices spanning the entire specimen were obtained for each dataset.
The CT datasets were resampled to 1.0-mm isotropic voxels, and all left knees were mirrored along the X-axis to produce 10 right knees for analysis. The BRAINS2 software was used to manually segment the distal femur and proximal tibia Citation[6]. 3DSlicer was used to generate and smooth surface representations from the manual tracings of the 10 femoral surfaces and 10 tibial surfaces.
An average-sized femoral and tibial 3D surface reconstruction were aligned such that the surface orientations corresponded to the standardized orientation described above. The resulting bone reconstructions were verified as matching the desired standardized orientation by an experienced orthopaedic surgeon. The oriented bone surface representation acted as an atlas to which each of the specimens was mapped using an iterative closest point (ICP) rigid registration, a common tool in 3D modeling. The ICP surface registration used 100 iterations and 5000 vertices to align the specimen surfaces without modifying the original bone geometry Citation[26], Citation[27].
Four users – two biomedical engineers and two orthopaedic residents – applied the proposed measurement techniques to evaluate the femoral and tibial ACL tunnels on each of the 10 specimens. Each user was independently trained and performed placement and aperture matching of virtual drill bits in the tibia and femur for the 10 cadaveric datasets on a single occasion. The resulting virtual drill bits were processed using the 3D measurement techniques described above.
Statistical analysis
Standard descriptive statistics were calculated for the angular and spatial measurements for both the femoral and tibial measurements using Microsoft Excel®. The reliability of the raters was evaluated by computing the intraclass correlation coefficients for the described spatial and angular measures using SAS®.
Results
The ten cadaveric specimens showed average and standard deviation values of α = 60 ± 1.2°, β = 50 ± 1.1°, γ = 56 ± 1.0°, n/N = 0.54 ± 0.02, and c/C = 0.68 ± 0.02 for the femur, and α = 78 ± 1.1°, β = 59 ± 1.4°, γ = 34 ± 1.3°, a/A = 0.49 ± 0.02, and m/M = 0.46 ± 0.01 for the tibia. This demonstrates the measurement system's ability to measure three angular and two spatial measurements for each of the ten ACL reconstructed knees. The intraclass correlation coefficient was calculated for the four users and found to range from 0.95 to 0.99 for the femoral and tibial measurements ().
Table I. Intracorrelation coefficient for 4 trained users for each measurement.
Discussion
We have presented a surgically oriented tunnel localization system for ACL reconstructions based on estimating the positioning of surgically generated tunnels for the tibia and femur using surface models. Our measurement guidelines are intentionally designed to be of practical use to the orthopaedic surgeon and provide surgically oriented proportional measurements based on the ESSKA recommendations Citation[10]. By establishing a surgically oriented coordinate system, the aperture spatial measurements and the tunnel angular measurements provide a novel 3D method for describing this inherently three-dimensional procedure.
Our methods are applicable for evaluating surgical procedures that involve the placement of surgical drill bits and bone tunnels such as ACL reconstruction. We have demonstrated their application on single-bundle ACL reconstructions, including the transtibial, medial portal, and two-incision techniques. Simply incorporating a second drill bit would also allow this to serve as a method of evaluation for double-bundle ACL reconstructions. In addition, the method could be used to evaluate PCL reconstruction and other procedures involving bone tunnels for graft placement. The methods are reliable, with intraclass correlation coefficients ranging from 0.95 to 0.99 among four users with both surgical and nonsurgical backgrounds. These high values can be attributed to a small degree of variability between raters in placing the virtual drill bits and a high degree of variability in the tunnel angles themselves. Intraclass correlation coefficients investigating intra-observer variability were considered unnecessary due to the excellent inter-observer correlation coefficients.
Blumensaat's line has traditionally been a radiographic landmark important in describing the placement of ACL tunnels in two dimensions. Recently, Forsythe et al. Citation[23] and Kopf et al. Citation[24] have demonstrated the use of 3D surfaces to characterize tunnel locations using quadrants and spatial dimensions based on Blumensaat's line; however, we found this landmark difficult to define reliably in a large set of knee specimens because of significant anatomical variation and undulation of Blumensaat's line on 3D surfaces. Hence, this induced variability in the measurements, since determining the starting point was difficult. We therefore provide an alternative measurement tool based on standardized bone orientations.
Our surgically oriented coordinate system does deviate from orientations that have been proposed in the past. With respect to the femur, Grood and Suntay Citation[28] proposed orienting the Z-axis so that it corresponded to the femoral mechanical axis (partially defined with the femoral head). With respect to the tibia, they proposed aligning the Z-axis with the tibial mechanical axis (partially defined with the tibial malleoli). Forsythe et al. Citation[23] and Kopf et al. Citation[24] based their coordinate system on the recommendations of the International Society of Biomechanics and Grood and Suntay Citation[28]. During ACL reconstructions, the femoral head is typically draped and is difficult to reference intraoperatively. In our measurement system, we have aligned the diaphysis of the femur with the Z-axis; this allows measurements to be taken with respect to a portion of the femur directly visible during the procedure. Our X- and Y- axes are defined similarly to those described by Grood and Suntay Citation[28]. For the tibia, instead of orienting with respect to the tibial malleoli, we have oriented the tibia with regards to surface features of the tibial plateau. Overall, our orientations were defined by aligning the femur and tibia with landmarks that are visible during the operative procedure, which is also helpful in cadaveric studies where the entire limb is not available.
Current limitations and areas for future work revolve around manual procedures in our methodology, including manual segmentation of the images and manual placement of virtual drill bits for the measurements. Future improvements to our methods will include fully automated surface generation of the tibia and femur Citation[29], and automated placement of the virtual drill bits into the drill tunnels to foster an automated measurement system. With these improvements, this tool could be used for surgical planning, where the optimal position of the tunnel would be identified automatically.
In current practice, MRI images are typically obtained in ACL reconstruction cases requiring revision. In our pilot study, we have used surfaces generated from CT images with 1.0-mm isotropic voxels, which could be seen as a limitation of our study's clinical applicability. Future applications of our methodology to MRI images will bring our methods closer to clinical usefulness. In addition, clinical studies will also include surgical hardware used in the ACL reconstruction, which can lead to beam hardening artifacts in images. These artifacts can affect image quality depending on the type of hardware configuration chosen by the surgeon. Our study did not include subjects with hardware; however, previous studies have demonstrated that the presence of hardware can actually be helpful in identifying ACL tunnels in postoperative images Citation[17]. Based on our experience with clinical images including hardware, we do not foresee hardware interfering with our methodology of ACL tunnel measurement.
As previously mentioned, our methods offer a number of advantages by avoiding measurements on Blumensaat's line and by using surgical landmarks that are visible during the procedure; however, results obtained using our measurement system can be difficult to compare directly to previous work due to the differences in methodology. In the future, we hope to develop an estimated mathematical conversion between our methods and previous approaches to allow for comparison to prior research.
From a research perspective, this is the first tool to allow for a 3D measurement of both angular and spatial dimensions in ACL reconstruction. The techniques presented in this study were developed to evaluate 150 ACL reconstructions performed by the MOON Knee Group to investigate ACL tunnel variability. This analysis will help to establish mean and extreme values encountered in ACL tunnel placement and in multi-surgeon cohort studies. With angular and spatial localization data, this work will also potentially allow future finite element investigations to be performed on ACL reconstructed knees to optimize tunnel position and orientation. Clinically, we hope a future version of this tool will allow individual surgeons to evaluate ACL tunnel placement in cases of reconstruction failure, thereby identifying technical failures and improving their practice, or to help plan revision surgery.
Conclusions
We have presented a surgically oriented three-dimensional method for evaluating tunnel placement/orientation for ACL reconstructions. These methods are reliable, with intraclass correlation coefficients ranging from 0.95 to 0.99. We have also demonstrated the methods to be applicable to multiple single-bundle techniques of ACL reconstruction.
Acknowledgments
The authors would like to acknowledge DonJoy Orthopaedics (Carlsbad, CA) for providing the cadaveric specimens and the Multicenter Orthopaedic Outcomes Network (MOON) Knee Group, based at Vanderbilt University, including Drs. Andrish, Amendola, Brophy, Carey, Cox, Dunn, Flanigan, Jones, Kaeding, Marx, Matava, McCarty, Parker, Spindler, Vidal, Wolcott and Wright, for providing the CT scans/surgery.
Declaration of interest: The authors report no declarations of interest.
References
- Endele D, Jung C, Becker U, Bauer G, Mauch F. Anterior cruciate ligament reconstruction with and without computer navigation: A clinical and magnetic resonance imaging evaluation 2 years after surgery. Arthroscopy 2009; 25: 1067–1074
- Beynnon BD, Johnson RJ, Abate JA, Fleming BC, Nichols CE. Treatment of anterior cruciate ligament injuries, part I. Am J Sports Med 2005; 33: 1579–1602
- Lyman S, Koulouvaris P, Sherman S, Do H, Mandl LA, Marx RG. Epidemiology of anterior cruciate ligament reconstruction: Trends, readmissions, and subsequent knee surgery. J Bone Joint Surg Am 2009; 91: 2321–2328
- Kendoff D, Citak M, Voos J, Pearle AD. Surgical navigation in knee ligament reconstruction. Clin Sports Med 2009; 28: 41–50
- Beynnon BD, Johnson RJ, Abate JA, Fleming BC, Nichols CE. Treatment of anterior cruciate ligament injuries, part 2. Am J Sports Med 2005; 33: 1751–1767
- Magnotta VA, Harris G, Andreasen NC, O’Leary DS, Yuh WT, Heckel D. Structural MR image processing using the BRAINS2 toolbox. Comput Med Imaging Graph 2002; 26: 251–264
- DeVries NA, Gassman EE, Kallemeyn NA, Shivanna KH, Magnotta VA, Grosland NM. Validation of phalanx bone three-dimensional surface segmentation from computed tomography images using laser scanning. Skeletal Radiol 2008; 37: 35–42
- Tadepalli SC, Shivanna KH, Magnotta VA, Kallemeyn NA, Grosland NM. Toward the development of virtual surgical tools to aid orthopaedic FE analyses. EURASIP J Adv Signal Process 2010; 2010: 1902931–1902937
- Schroeder W, Martin K, Lorensen B. The Visualization Toolkit4th edition. Inc, Kitware, 2006
- Amis AA, Beynnon B, Blankevoort L, Chambat P, Christel P, Durselen L, Friederich N, Grood E, Hertel P, Jakob R. Proceedings of the ESSKA Scientific Workshop on Reconstruction of the Anterior and Posterior Cruciate Ligaments. Knee Surg Sports Traumatol Arthrosc 1994; 2: 124–132
- Dargel J, Schmidt-Wiethoff R, Fischer S, Mader K, Koebke J, Schneider T. Femoral bone tunnel placement using the transtibial tunnel or the anteromedial portal in ACL reconstruction: A radiographic evaluation. Knee Surg Sports Traumatol Arthrosc 2009; 17: 220–227
- Howell SM, Gittins ME, Gottlieb JE, Traina SM, Zoellner TM. The relationship between the angle of the tibial tunnel in the coronal plane and loss of flexion and anterior laxity after anterior cruciate ligament reconstruction. Am J Sports Med 2001; 29: 567–574
- Pinczewski LA, Salmon LJ, Jackson WF, von Bormann RB, Haslam PG, Tashiro S. Radiological landmarks for placement of the tunnels in single-bundle reconstruction of the anterior cruciate ligament. J Bone Joint Surg Br 2008; 90: 172–179
- Giron F, Cuomo P, Aglietti P, Bull AM, Amis AA. Femoral attachment of the anterior cruciate ligament. Knee Surg Sports Traumatol Arthrosc 2006; 14: 250–256
- Aglietti P, Zaccherotti G, Menchetti PP, De Biase P. A comparison of clinical and radiological parameters with two arthroscopic techniques for anterior cruciate ligament reconstruction. Knee Surg Sports Traumatol Arthrosc 1995; 3: 2–8
- Hoser C, Tecklenburg K, Kuenzel KH, Fink C. Postoperative evaluation of femoral tunnel position in ACL reconstruction: Plain radiography versus computed tomography. Knee Surg Sports Traumatol Arthrosc 2005; 13: 256–262
- Warme BA, Ramme AJ, Willey MC, Britton CL, Flint JH, Amendola AS, Wolf BR, MOON Knee Group. Reliability of early postoperative radiographic assessment of tunnel placement after anterior cruciate ligament reconstruction. Arthroscopy 2012 (in press)
- Bedi A, Raphael B, Maderazo A, Pavlov H, Williams RJ 3rd. Transtibial versus anteromedial portal drilling for anterior cruciate ligament reconstruction: A cadaveric study of femoral tunnel length and obliquity. Arthroscopy 2010; 26: 342–350
- Chan YS, Lo YP, Lien LC, Fu CJ, Wan YL, Hsu KY, Wang CJ, Chen WJ. Improved divergence angles with femoral interference screw placement through the tibial tunnel as measured by multiplanar reconstruction computed tomography. Arthroscopy 2009; 25: 54–61
- Abebe ES, Moorman CT 3rd, Dziedzic TS, Spritzer CE, Cothran RL, Taylor DC, Garrett WE Jr, DeFrate LE. Femoral tunnel placement during anterior cruciate ligament reconstruction: An in vivo imaging analysis comparing transtibial and 2-incision tibial tunnel-independent techniques. Am J Sports Med 2009; 37: 1904–1911
- Miller MD, Gerdeman AC, Miller CD, Hart JM, Gaskin CM, Golish SR, Clancy WG Jr. The effects of extra-articular starting point and transtibial femoral drilling on the intra-articular aperture of the tibial tunnel in ACL reconstruction. Am J Sports Med 2010; 38: 707–712
- Tsuda E, Ishibashi Y, Fukuda A, Yamamoto Y, Tsukada H, Ono S. Tunnel position and relationship to postoperative knee laxity after double-bundle anterior cruciate ligament reconstruction with a transtibial technique. Am J Sports Med 2010; 38: 698–706
- Forsythe B, Kopf S, Wong AK, Martins CA, Anderst W, Tashman S, Fu FH. The location of femoral and tibial tunnels in anatomic double-bundle anterior cruciate ligament reconstruction analyzed by three-dimensional computed tomography models. J Bone Joint Surg Am 2010; 92: 1418–1426
- Kopf S, Forsythe B, Wong AK, Tashman S, Anderst W, Irrgang JJ, Fu FH. Nonanatomic tunnel position in traditional transtibial single-bundle anterior cruciate ligament reconstruction evaluated by three-dimensional computed tomography. J Bone Joint Surg Am 2010; 92: 1427–1431
- Tsukada H, Ishibashi Y, Tsuda E, Fukuda A, Toh S. Anatomical analysis of the anterior cruciate ligament femoral and tibial footprints. J Orthop Sci 2008; 13: 122–129
- Sharp GC, Lee SW, Wehe DK, Invariant features and the registration of rigid bodies. In: Proceedings of the IEEE International Conference on Robotics and Automation 1999 (ICRA 1999), Detroit, MI, May 1999. pp 932–937
- Sharp GC, Lee SW, Wehe DK. ICP registration using invariant features. IEEE Trans Pattern Anal Mach Intell 2002; 24: 90–102
- Grood ES, Suntay WJ. A joint coordinate system for the clinical description of three-dimensional motions: Application to the knee. J Biomech Eng 1983; 105: 136–144
- Ramme AJ, Criswell AJ, Wolf BR, Magnotta VA, Grosland NM. EM segmentation of the distal femur and proximal tibia: A high-throughput approach to anatomic surface generation. Ann Biomed Eng 2011; 39: 1555–1562