Abstract
Chronic heart failure (HF) is a major health problem throughout the world. Gender has significant effects on the pathophysiology of HF. Low levels of free testosterone are independently associated with increased chronic HF symptoms and mortality. Cardiac fibrosis plays a pivotal role in structural remodeling in HF. Transforming growth factor (TGF)-β, angiotensin II and oxidative stress contribute to the activity/extent of cardiac fibrosis. Androgen deficiency can up-regulate TGF-β expression under angiotensin II stimulation in vivo. In this review, we summarized the potential mechanisms accounting for the effects of androgen on cardiac fibrosis through regulating fibrocytes activity under TGF, which can explain wound healing and cardiac fibrosis in male with acute myocardial injury and chronic HF.
Introduction
Heart failure (HF) is a common cause of hospitalization with a poor prognosis in clinical practice [Citation1–4]. Gender plays an important role in the pathophysiology of HF. Female HF patients have more diastolic HF, but male HF is associated with more cardiac ischemia [Citation5–7]. Androgen treatment was shown to improve clinical outcome in male patients with HF [Citation8,Citation9]. HF is associated with increased cardiac fibrosis and structural remodeling [Citation10,Citation11]. Transforming growth factor (TGF)-β, angiotensin (Ang) II, oxidative stress play important roles in the pathophysiology of HF and were also demonstrated to increase the activity of cardiac fibroblasts [Citation12–14]. Estrogen was shown to modulate cardiac fibrosis through inhibition of Ang II-induced collagen synthesis and gel contraction of cardiac fibroblasts [Citation15,Citation16]. Moreover, androgen was shown to modulate the effects of Ang II and possesses an anti-oxidative ability, which may modulate cardiac fibrosis and play a role in the pathophysiology of HF [Citation17,Citation18]. In this review, we summarized the potential mechanisms accounting for the effects of androgen on cardiac fibrosis and HF.
Androgen in HF
In male HF patients, anabolic hormone depletion is common, and reduced levels of androgen and insulin growth factor-1 are related to unfavorable outcomes in men with HF [Citation19]. Wehr et al. [Citation20] proved that low levels of free testosterone are independently associated with increased chronic HF mortality and symptoms [Citation21]. Therefore, androgen deficiency may be responsible for some of the features of advanced HF such as reduced mass of skeletal muscle, abnormal energy handling, cachexia, low mood, depression and fatigue [Citation8,Citation9,Citation22,Citation23]. A randomized, double-blind, placebo-controlled cross-over trial in 2003 reported that administration of testosterone via the buccal route acutely increased cardiac output in men with stable HF [Citation24]. Malkin et al. [Citation8] showed that in men with moderate severity systolic HF, testosterone therapy significantly improved their functional capacity and symptoms, but did not change their left ventricular (LV) ejection fraction. Another trial also showed that long-acting testosterone treatment could improve the functional exercise capacity, skeletal muscle performance, insulin resistance and baroreflex sensitivity, but not the LV ejection fraction, in elderly patients with chronic systolic HF [Citation25]. Moreover, Iellamo et al. [Citation26] found that testosterone therapy improves functional capacity, insulin resistance and muscle strength in women with advanced chronic HF, although the dosage of testosterone used in this study was significantly lower than those in males [Citation8,Citation9,Citation25]. Previous studies revealed that testosterone induce hypertrophy in cardiac myocytes via direct, receptor-specific mechanisms [Citation27] or mammalian target of rapamycin complex 1 pathway [Citation28]. Athletes abusing supraphysiological dose of testosterone may exhibit LV hypertrophy [Citation29,Citation30]. In contrast, there was a trend to a reduction in LV mass index in male patients with moderate severity HF [Citation8]. Therefore, different dosage of testosterone may have inconsistent effects. However, there is no information about the effects of testosterone replacement therapy specified for HF patients with hypertrophic cardiomyopathy. summarizes outcomes of clinical trials of testosterone on HF patients. It seems that testosterone supplementation acts only through peripheral mechanisms according to the latter two trials. However, whether testosterone act directly on patient’s LV diastolic function has not yet been proven in a large clinical trial.
Table 1. Clinical outcome of testosterone therapy in chronic HF.
Myocardial ischemia is an important factor of HF. Androgen deficiency has been documented as a risk factor for a plethora of disease associated with atherosclerosis including the metabolic syndrome, insulin resistance and diabetes [Citation31,Citation32]. Low levels of testosterone are associated with low levels of high-density lipoprotein cholesterol (HDL-C) and high levels of low-density lipoprotein cholesterol (LDL-C) [Citation33,Citation34]. Testosterone therapy was proven to reduce total cholesterol and LDL-C levels [Citation35,Citation36]. However, the effects of testosterone therapy on HDL were controversial [Citation37–41]. Testosterone therapy has been shown to increase HDL-C levels in male patients with metabolic syndrome and hypogonadism [Citation35,Citation37]. In contrast, another trials demonstrated testosterone therapy did not change HDL-C levels in hypogonadal and healthy elderly men [Citation38,Citation39] or even reduced HDL-C levels in healthy men [Citation40,Citation41]. These discrepancies might be caused by a different dosage or a route of androgen administration, or a different hypogonadal state in these patients [Citation42].
Cardiac fibrosis in HF
Adverse LV remodeling is considered an intermediate phenotype of HF [Citation10]. Cardiac fibrosis is characterized by the net accumulation of extracellular matrix (ECM) in the myocardium and the concentration of type I collagen is a major determinant of myocardial diastolic stiffness [Citation11,Citation43]. ECM remodeling is an essential process in cardiac remodeling, hypertensive cardiac hypertrophy and post-infarction healing [Citation44,Citation45]. Post-infarction remodeling is divided into an early phase (within 72 h) and late phase (beyond 72 h) [Citation46]. The infarct zone expansion, which occurs in the early phase, results from degradation of intermyocyte collagen frameworks by serine proteases and activation of matrix metalloproteinases (MMPs) released from neutrophils [Citation47,Citation48]. Infarct expansion causes wall thinning and ventricular dilatation, and enhances diastolic and systolic wall stress [Citation46]. Local Ang II is activated by wall stress and induces cardiac hypertrophy in non-infarcted zones [Citation49]. During late remodeling, TGF-β activates type I and III collagen synthesis and promotes myocardial fibrosis [Citation46]. A fundamental characteristic of hypertensive cardiac remodeling is myocardial stiffness [Citation50]. Myocardial stiffness is mostly due to changes in the composition and arrangement of ECM proteins, including type I and III collagens [Citation51,Citation52]. Compared with normotensive rats, spontaneously hypertensive rats show greater amount of pro-collagen type I in the myocardium [Citation53,Citation54]. Following the development of cardiac hypertrophy, spontaneously hypertensive rats also have more collagen cross-linking in the myocardium [Citation55]. Blood pressure increase is accompanied by increased collagen and total protein synthesis and diminished protein degradation in rodent hearts [Citation56,Citation57]. These evidences suggest that hypertension might contribute to changes in the ECM and fibrosis and lead to HF in the future. In patients with chronic HF, a higher level of cardiac fibrosis is associated with poor outcomes [Citation58]. A higher level of collagen scar formation is correlated with adverse LV function in patients after acute myocardial infarction (MI) [Citation59]. In patients with hypertensive heart disease, fibrosis may also contribute to HF and other cardiac complications [Citation60].
TGF-β and Ang II are likely to be the key driving forces culminating in fibrosis [Citation61]. TGF-β expression is elevated in response to injury [Citation62]. Increased messenger RNA levels of TGF-β1 were observed in cardiac fibroblasts isolated from rats one week after experimental MI [Citation63]. It critically modulates the cardiac fibroblast phenotype and function [Citation64]. TGF-β induces differentiation of cardiac fibroblasts to myofibroblasts, which further stimulate collagen production [Citation12,Citation65]. It also induces fibroblasts to synthesize and contract ECM protein [Citation66,Citation67]. In addition, TGF-β exerts potent matrix-preserving actions by suppressing the activity of MMP and inducing synthesis of protease inhibitors, like tissue inhibitors of metalloproteinases (TIMPs) [Citation63,Citation64]. LV hypertrophy is associated with increased levels of circulating TIMP 1 but decreased levels of circulating MMP 1 in patients with hypertension [Citation44]. Myocardial levels of Ang II increase in a number of pathologies characterized by myocardial remodeling [Citation68]. Ang II can induce proliferation of cardiac myofibroblasts from the non-infarcted zone of rat hearts with MI [Citation13]. Ang II also stimulates the pro-fibrotic effects of cardiac fibroblasts via multiple mechanisms, including increased ECM protein synthesis, decreased MMP activity and increased TIMP activity [Citation69]. Previous studies revealed that Ang II and TGF-β1 do not act independently of one another but act as a network that promotes cardiac fibrosis. Ang II stimulates TGF-β1 gene expression and protein production in cardiac fibroblasts and myofibroblasts which may act as an autocrine/paracrine stimulus to collagen formation [Citation70]. A further study indicated that Ang II was not able to induce cardiac hypertrophy and fibrosis in vivo without TGF-β [Citation71].
Interactions of androgen and TGF-β
A plethora of studies evaluated interactions between androgen and the TGF-β signaling pathway. In different cell lines, androgen has different influences on the TGF-β signal pathway. TGF-β1 may be an important component of the inhibitory complex influencing androgen receptor positive cell proliferation. In adrenalcortical carcinoma cell line, dihydrotestosterone stimulated significant up-regulation of TGF-β1 messenger RNA and protein that reduced proliferation of the adrenocortical cancer cell line in vitro [Citation72]. In ovarian cancer cell line, dihydrotestosterone was able to reverse TGF-β1 growth-inhibitory action. Thus, androgens may induce ovarian cancer progression through decreasing TGF-β receptor levels, thereby allowing ovarian cancer cells to escape TGF-β1 growth inhibition [Citation73]. In prostate cancer cell line, Androgen can block TGF-β responses in prostate epithelial cells through an association of the androgen receptor with SMA and MAD-related protein (Smad)-3, which inhibits the binding of Smad3 to Smad-binding elements in TGF-β responsive promoters. This provides a possible explanation for the positive role of TGF-β in androgen-promoted prostate cancer growth [Citation74]. An important study in 2005 indicated that dehydroepiandrosterone, a type of adrenal androgen, can directly attenuate collagen type I synthesis in vitro in cardiac fibroblasts [Citation75]. However, the underlying mechanism has not yet been elucidated.
Interactions of androgen and angiotensin II
Androgen receptor gene knockout mice exhibit exacerbation of Ang II-induced cardiac fibrosis. Ang II stimulation enhanced cardiac TGF-β1 gene expression and SMA and MAD-related protein (Smad)-2 phosphorylation in androgen receptor gene knockout mice more than in wild-type mice [Citation76]. The same kind of phenomenon was also observed in the aorta after Ang II stimulation. Androgen signaling plays an protective role against Ang II-induced vascular remodeling [Citation17].
Interactions of androgen and oxidative stress
Multiple clinical and experimental evidences support the connection between oxidative stress and the severity of HF [Citation77–79]. Oxidative stress activation was demonstrated in pressure overload LV hypertrophy in mice [Citation80,Citation81]. Previous studies also proved that reactive oxygen species (ROS) derived from NADPH oxidase (Nox)-2 play important roles in the development of interstitial cardiac fibrosis [Citation82–85]. ROS stimulate cardiac fibroblasts proliferation by increasing the production of TGF-β1 [Citation14]. ROS derived from Nox-4 are also involved in TGF-β1-induced myofibroblast differentiation in the kidney and heart [Citation86,Citation87]. Blocking androgen receptor signaling may induce oxidative stress was documented in some experiments. In the rat and human prostate, castration or androgen deprivation may increase the pro-oxidant properties by significantly upregulating NADPH oxidase, and also decrease the anti-oxidant ability by reducing ROS-detoxifying enzymes such as manganese superoxide dismutase, thioredoxin1 and peroxiredoxin [Citation88–91]. Anti-oxidant therapy can alleviate oxidative stress by androgen deprivation therapy in prostate cancer [Citation92]. The androgen receptor counteracts ROS damage to the heart induced by doxorubicin in mice [Citation18]. Therefore, androgen signaling might play a protective role against toxicity from oxidative stress.
Summary
As shown in , androgen acting on cardiac fibroblasts elicits anti-fibrotic effects on the heart through down-regulating the signal pathway of TGF-β, blocking the effect of Ang II, and alleviating the pro-fibrotic properties of ROS. Androgen deficiency can promote cardiac fibroblast activation via induction greater TGF-β, Ang II and oxidative stress expression. Therefore, in the initial phase of myocardial injury, androgen might disturb the normal wound healing process. These speculated mechanisms can explain the higher LV wall rupture rate at the acute phase of MI in male mice than in female mice [Citation93–95] being reversed by androgen depletion therapy [Citation93]. On the other hand, chronic androgen deficiency might persistently stimulate the differentiation of cardiac fibroblasts to cardiac myofibroblasts through the over-expression of TGF-β and increase myocardial fibrosis in chronic disease. These effects can explain the phenomenon discovered in clinical studies that androgen deficiency is closely related to the severity and mortality rate of chronic HF. Testosterone supplementation has been documented to be beneficial for HF patients. Testosterone level should be monitored in male HF patients and testosterone therapy can be considered in HF patients.
Figure 1. Mechanisms of the anti-fibrotic effects of androgen on the heart. Androgen suppresses the differentiation of cardiac fibroblasts to cardiac myofibroblasts through blocking the effect of angiotensin II, alleviating the pro-fibrotic properties of oxidative stress and down-regulating the signal pathway of TGF-β. MMP: matrix metalloproteinases; TIMP: tissue inhibitors of metalloproteinases.
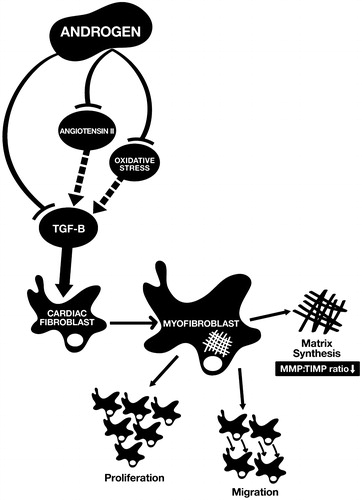
Declaration of interest
The authors report no conflicts of interest. The authors alone are responsible for the content and writing of this article.
This study was supported by a grant from Taipei Medical University-Wan Fang Hospital Grant no. 100swf05.
References
- Bonneux L, Barendregt JJ, Meeter K, et al. Estimating clinical morbidity due to ischemic heart disease and congestive heart failure: the future rise of heart failure. Am J Public Health 1994;84:20–8
- Ho KK, Pinsky JL, Kannel WB, Levy D. The epidemiology of heart failure: the Framingham study. J Am Coll Cardiol 1993;22:6A–13A
- Reitsma JB, Mosterd A, de Craen AJ, et al. Increase in hospital admission rates for heart failure in The Netherlands, 1980–1993. Heart 1996;76:388–92
- Jong P, Vowinckel E, Liu PP, et al. Prognosis and determinants of survival in patients newly hospitalized for heart failure: a population-based study. Arch Intern Med 2002;162:1689–94
- Ghali JK, Krause-Steinrauf HJ, Adams Jr.KF, et al. Gender differences in advanced heart failure: insights from the BEST study. J Am Coll Cardiol 2003;42:2128–34
- Pilote L, Dasgupta K, Guru V, et al. A comprehensive view of sex-specific issues related to cardiovascular disease. CMAJ 2007;176:S1–44
- Luann GR. Women and heart failure. Heart Lung 2001;30:87–97
- Malkin CJ, Pugh PJ, West JN, et al. Testosterone therapy in men with moderate severity heart failure: a double-blind randomized placebo controlled trial. Eur Heart J 2006;27:57–64
- Pugh PJ, Jones RD, West JN, et al. Testosterone treatment for men with chronic heart failure. Heart 2004;90:446–7
- Cheng S, Vasan RS. Advances in the epidemiology of heart failure and left ventricular remodeling. Circulation 2011;124:e516–9
- Dobaczewski M, Frangogiannis NG. Chemokines and cardiac fibrosis. Front Biosci (Schol Ed) 2009;1:391–405
- Petrov VV, Fagard RH, Lijnen PJ. Stimulation of collagen production by transforming growth factor-β1 during differentiation of cardiac fibroblasts to myofibroblasts. Hypertension 2002;39:258–63
- Staufenberger S, Jacobs M, Brandstätter K, et al. Angiotensin II type1 receptor regulation and differential trophic effects on rat cardiac myofibroblasts after acute myocardial infarction. J Cell Physiol 2001;187:326–35
- Li P-F, Dietz R, von Harsdorf R. Superoxide induces apoptosis in cardiomyocytes, but proliferation and expression of transforming growth factor-beta1 in cardiac fibroblasts. FEBS Lett 1999;448:206–10
- Stewart JrJA, Cashatt DO, Borck AC, et al. 17β-estradiol modulation of angiotensin II-stimulated response in cardiac fibroblasts. J Mol Cell Cardiol 2006;41:97–107
- Zhou L, Shao Y, Huang Y, et al. 17β-estradiol inhibits angiotensin II-induced collagen synthesis of cultured rat cardiac fibroblasts via modulating angiotensin II receptors. Eur J Pharmacol 2007;567:186–92
- Ikeda Y, Aihara K, Yoshida S, et al. Androgen-androgen receptor system protects against angiotensin II-induced vascular remodeling. Endocrinology 2009;150:2857–64
- Ikeda Y, Aihara K, Akaike M, et al. Androgen receptor counteracts doxorubicin-induced cardiotoxicity in male mice. Mol Endocrinol 2010;24:1338–48
- Jankowska EA, Biel B, Majda J, et al. Anabolic deficiency in men with chronic heart failure: prevalence and detrimental impact on survival. Circulation 2006;114:1829–37
- Wehr E, Pilz S, Boehm BO, et al. Low free testosterone is associated with heart failure mortality in older men referred for coronary angiography. Eur J Heart Fail 2011;13:482–88
- Güder G, Frantz S, Bauersachs J, et al. Low circulating androgens and mortality risk in heart failure. Heart 2010;96:504–9
- Barrett-Connor E, von Mühlen DG, Kritz-Silverstein D. Bioavailable testosterone and depressed mood in older men: the Rancho Bernardo Study. J Clin Endocrinol Metab 1999;84:573–77
- Rumsfeld JS, Havranek E, Masoudi FA, et al. Depressive symptoms are the strongest predictors of short-term declines in health status in patients with heart failure. J Am Coll Cardiol 2003;42:1811–7
- Pugh PJ, Jones TH, Channer KS. Acute haemodynamic effects of testosterone in men with chronic heart failure. Eur Heart J 2003;24:909–15
- Caminiti G, Volterrani M, Iellamo F, et al. Effect of long-acting testosterone treatment on functional exercise capacity, skeletal muscle performance, insulin resistance, and baroreflex sensitivity in elderly patients with chronic heart failure: a double-blind, placebo-controlled, randomized study. J Am Coll Cardiol 2009;54:919–27
- Iellamo F, Volterrani M, Caminiti G, et al. Testosterone therapy in women with chronic heart failure: a pilot double-blind, randomized, placebo-controlled study. J Am Coll Cardiol 2010;56:1310–6
- Marsh JD, Lehmann MH, Ritchie RH, et al. Androgen receptors mediate hypertrophy in cardiac myocytes. Circulation 1998;98:256–61
- Altamirano F, Oyarce C, Silva P, et al. Testosterone induces cardiomyocyte hypertrophy through mammalian target of rapamycin complex 1 pathway. J Endocrinol 2009;202:299–307
- Sachtleben TR, Berg KE, Elias BA, et al. The effects of anabolic steroids on myocardial structure and cardiovascular fitness. Med Sci Sports Exerc 1993;25:1240–5
- Urhausen A, Hölpes R, Kindermann W. One- and two-dimensional echocardiography in bodybuilders using anabolic steroids. Eur J Appl Physiol Occup Physiol 1989;58:633–40
- Traish AM, Guay A, Feeley R, Saad F. The dark side of testosterone deficiency: I. Metabolic syndrome and erectile dysfunction. J Androl 2009;30:10–22
- Traish AM, Saad F, Guay A. The dark side of testosterone deficiency: II. Type 2 diabetes and insulin resistance. J Androl 2009;30:23–32
- Barrett-Connor E. Lower endogenous androgen levels and dyslipidemia in men with non-insulin-dependent diabetes mellitus. Ann Intern Med 1992;117:807–11
- Barud W, Palusiński R, Bełtowski J, Wójcicka G. Inverse relationship between total testosterone and anti-oxidized low density lipoprotein antibody levels in ageing males. Atherosclerosis 2002;164:283–8
- Saad F, Gooren L, Haider A, Yassin A. An exploratory study of the effects of 12 month administration of the novel long-acting testosterone undecanoate on measures of sexual function and the metabolic syndrome. Arch Androl 2007;53:353–7
- Saad F, Gooren LJ, Haider A, Yassin A. A dose-response study of testosterone on sexual dysfunction and features of the metabolic syndrome using testosterone gel and parenteral testosterone undecanoate. J Androl 2008;29:102–5
- Zitzmann M, Nieschlag E. Androgen receptor gene CAG repeat length and body mass index modulate the safety of long-term intramuscular testosterone undecanoate therapy in hypogonadal men. J Clin Endocrinol Metab 2007;92:3844–53
- Zgliczynski S, Ossowski M, Slowinska-Srzednicka J, et al. Effect of testosterone replacement therapy on lipids and lipoproteins in hypogonadal and elderly men. Atherosclerosis 1996;121:35–43
- Uyanik BS, Ari Z, Gümüs B, et al. Beneficial effects of testosterone undecanoate on the lipoprotein profiles in healthy elderly men: a placebo controlled study. Jpn Heart J 1997;38:73–82
- Thompson PD, Cullinane EM, Sady SP, et al. Contrasting effects of testosterone and stanozolol on serum lipoprotein levels. JAMA 1989;261:1165–8
- Bagatell CJ, Heiman JR, Matsumoto AM, et al. Metabolic and behavioral effects of high-dose, exogenous testosterone in healthy men. J Clin Endocrinol Metab 1994;79:561–7
- Traish AM, Abdou R, Kypreos KE. Androgen deficiency and atherosclerosis: the lipid link. Vascul Pharmacol 2009;51:303–3
- Weber K, Janicki J, Shroff S, et al. Collagen remodeling of the pressure-overloaded, hypertrophied nonhuman primate myocardium. Circ Res 1988;62:757–65
- Laviades C, Varo N, Fernández J, et al. Abnormalities of the extracellular degradation of collagen type I in essential hypertension. Circulation 1998;98:535–40
- Factor SM, Robinson TF, Dominitz R, Cho SH. Alterations of the myocardial skeletal framework in acute myocardial infarction with and without ventricular rupture: a preliminary report. Am J Cardiovasc Pathol 1987;1:91–7
- Sutton MG, Sharpe N. Left ventricular remodeling after myocardial infarction: pathophysiology and therapy. Circulation 2000;101:2981–8
- Erlebacher JA, Weiss JL, Weisfeldt ML, Bulkley BH. Early dilation of the infarcted segment in acute transmural myocardial infarction: role of infarct expansion in acute left ventricular enlargement. J Am Coll Cardiol 1984;4:201–8
- Cleutjens JP, Kandala JC, Guarda E, et al. Regulation of collagen degradation in the rat myocardium after infarction. J Mol Cell Cardiol 1995;27:1281–92
- Sadoshima J, Jahn L, Takahashi T, et al. Molecular characterization of the stretch-induced adaptation of cultured cardiac cells: an in vitro model of load-induced cardiac hypertrophy. J Biol Chem 1992;267:10551–60
- Berk BC, Fujiwara K, Lehoux S. ECM remodeling in hypertensive heart disease. J Clin Invest 2007;117:568–75
- Weber KT, Pick R, Jalil JE, et al. Patterns of myocardial fibrosis. J Mol Cell Cardiol 1989;21:121–31
- Weber KT. Cardiac interstitium in health and disease: the fibrillar collagen network. J Am Coll Cardiol 1989;13:1637–52
- Diez J, Hernandez M. Is the extracellular degradation of collagen type I fibers depressed in spontaneously hypertensive rats with myocardial fibrosis? Circulation 1996;94:2998
- Díez J, Panizo A, Gil MJ, et al. Serum markers of collagen type I metabolism in spontaneously hypertensive rats: relation to myocardial fibrosis. Circulation 1996;93:1026–32
- Norton GR, Tsotetsi J, Trifunovic B, et al. Myocardial stiffness is attributed to alterations in cross-linked collagen rather than total collagen or phenotypes in spontaneously hypertensive rats. Circulation 1997;96:1991–8
- Gordon EE, Kira Y, Demers LM, Morgan HE. Aortic pressure as a determinant of cardiac protein degradation. Am J Physiol 1986;250:C932–8
- Morgan HE, Siehl D, Chua BH, Lautensack-Belser N. Faster protein and ribosome synthesis in hypertrophying heart. Basic Res Cardiol 1985;80:115–8
- Zannad F, Alla F, Dousset B, et al. Limitation of excessive extracellular matrix turnover may contribute to survival benefit of spironolactone therapy in patients with congestive heart failure: insights from the randomized aldactone evaluation study (RALES). Circulation 2000;102:2700–6
- Uusimaa P, Risteli J, Niemelä M, et al. Collagen scar formation after acute myocardial infarction: relationships to infarct size, left ventricular function, and coronary artery patency. Circulation 1997;96:2565–72
- Díez J. Mechanisms of cardiac fibrosis in hypertension. J Clin Hypertens (Greenwich) 2007;9:546–50
- Leask A. Potential therapeutic targets for cardiac fibrosis: TGFbeta, angiotensin, endothelin, CCN2, and PDGF, partners in fibroblast activation. Circ Res 2010;106:1675–80
- Kane CJ, Hebda PA, Mansbridge JN, Hanawalt PC. Direct evidence for spatial and temporal regulation of transforming growth factor beta 1 expression during cutaneous wound healing. J Cell Physiol 1991;148:157–73
- Schiller M, Javelaud D, Mauviel A. TGF-beta-induced SMAD signaling and gene regulation: consequences for extracellular matrix remodeling and wound healing. J Dermatol Sci 2004;35:83–92
- Mauviel A. Transforming growth factor-beta: a key mediator of fibrosis. Methods Mol Med 2005;117:69–80
- Desmoulière A, Geinoz A, Gabbiani F, Gabbiani G. Transforming growth factor-beta 1 induces alpha-smooth muscle actin expression in granulation tissue myofibroblasts and in quiescent and growing cultured fibroblasts. J Cell Biol 1993;122:103–11
- LeRoy EC, Trojanowska MI, Smith EA. Cytokines and human fibrosis. Eur Cytokine Netw 1990;1:215–9
- Verrecchia F, Mauviel A. Transforming growth factor-beta signaling through the Smad pathway: role in extracellular matrix gene expression and regulation. J Invest Dermatol 2002;118:211–5
- Swynghedauw B. Molecular mechanisms of myocardial remodeling. Physiol Rev 1999;79:215–62
- Porter KE, Turner NA. Cardiac fibroblasts: at the heart of myocardial remodeling. Pharmacol Ther 2009;123:255–78
- Campbell SE, Katwa LC. Angiotensin II stimulated expression of transforming growth factor-beta1 in cardiac fibroblasts and myofibroblasts. J Mol Cell Cardiol 1997;29:1947–58
- Schultz JEJ, Witt SA, Glascock BJ, et al. TGF-beta1 mediates the hypertrophic cardiomyocyte growth induced by angiotensin II. J Clin Invest 2002;109:787–96
- Zatelli MC, Rossi R, degli Uberti EC. Androgen influences transforming growth factor-beta1 gene expression in human adrenocortical cells. J Clin Endocrinol Metab 2000;85:847–52
- Evangelou A, Jindal SK, Brown TJ, Letarte M. Down-regulation of transforming growth factor beta receptors by androgen in ovarian cancer cells. Cancer Res 2000;60:929–35
- Chipuk JE, Cornelius SC, Pultz NJ, et al. The androgen receptor represses transforming growth factor-beta signaling through interaction with Smad3. J Biol Chem 2002;277:1240–8
- Iwasaki T, Mukasa K, Yoneda M, Ito S, et al. Marked attenuation of production of collagen type I from cardiac fibroblasts by dehydroepiandrosterone. Am J Physiol Endocrinol Metab 2005;288:E1222–8
- Ikeda Y, Aihara K, Sato T, et al. Androgen receptor gene knockout male mice exhibit impaired cardiac growth and exacerbation of angiotensin II-induced cardiac fibrosis. J Biol Chem 2005;280:29661–6
- Mallat Z, Philip I, Lebret M, et al. Elevated levels of 8-iso-prostaglandin F2alpha in pericardial fluid of patients with heart failure: a potential role for in vivo oxidant stress in ventricular dilatation and progression to heart failure. Circulation 1998;97:1536–9
- McMurray J, Chopra M, Abdullah I, et al. Evidence of oxidative stress in chronic heart failure in humans. Eur Heart J 1993;14:1493–8
- Valgimigli M, Merli E, Malagutti P, et al. Hydroxyl radical generation, levels of tumor necrosis factor-alpha, and progression to heart failure after acute myocardial infarction. J Am Coll Cardiol 2004;43:2000–8
- Byrne JA, Grieve DJ, Bendall JK, et al. Contrasting roles of NADPH oxidase isoforms in pressure-overload versus angiotensin II-induced cardiac hypertrophy. Circ Res 2003;93:802–5
- Maytin M, Siwik DA, Ito M, et al. Pressure overload-induced myocardial hypertrophy in mice does not require gp91phox. Circulation 2004;109:1168–71
- Bendall JK, Cave AC, Heymes C, et al. Pivotal role of a gp91phox-containing NADPH oxidase in angiotensin II-induced cardiac hypertrophy in mice. Circulation 2002;105:293–6
- Grieve DJ, Byrne JA, Siva A, et al. Involvement of the nicotinamide adenosine dinucleotide phosphate oxidase isoform Nox2 in cardiac contractile dysfunction occurring in response to pressure overload. J Am Coll Cardiol 2006;47:817–26
- Johar S, Cave AC, Narayanapanicker A, et al. Aldosterone mediates angiotensin II-induced interstitial cardiac fibrosis via a Nox2-containing NADPH oxidase. FASEB J 2006;20:1546–8
- Park YM, Park MY, Suh Y-L, Park JB. NAD(P)H oxidase inhibitor prevents blood pressure elevation and cardiovascular hypertrophy in aldosterone-infused rats. Biochem Biophys Res Commun 2004;313:812–7
- Cucoranu I, Clempus R, Dikalova A, et al. NAD(P)H oxidase 4 mediates transforming growth factor-beta1–induced differentiation of cardiac fibroblasts into myofibroblasts. Circ Res 2005;97:900–7
- Bondi CD, Manickam N, Lee DY, et al. NAD(P)H oxidase mediates TGF-beta1-induced activation of kidney myofibroblasts. J Am Soc Nephrol 2010;21:93–102
- Tam NNC, Gao Y, Leung YK, Ho SM. Androgenic regulation of oxidative stress in the rat prostate: involvement of NAD(P)H oxidases and antioxidant defense machinery during prostatic involution and regrowth. Am J Pathol 2003;163:2513–22
- Best CJM, Gillespie JW, Yi Y, et al. Molecular alterations in primary prostate cancer after androgen ablation therapy. Clin Cancer Res 2005;11:6823–34
- Pang ST, Dillner K, Wu X, et al. Gene expression profiling of androgen deficiency predicts a pathway of prostate apoptosis that involves genes related to oxidative stress. Endocrinology 2002;143:4897–906
- Shan W, Zhong W, Zhao R, Oberley TD. Thioredoxin 1 as a subcellular biomarker of redox imbalance in human prostate cancer progression. Free Radic Biol Med 2010;49:2078–87
- Shiota M, Song Y, Takeuchi A, et al. Antioxidant therapy alleviates oxidative stress by androgen deprivation and prevents conversion from androgen dependent to castration resistant prostate cancer. J Urol 2012;187:707–14
- Cavasin MA, Sankey SS, Yu AL, et al. Estrogen and testosterone have opposing effects on chronic cardiac remodeling and function in mice with myocardial infarction. Am J Physiol Heart Circ Physiol 2003;284:H1560–9
- Cavasin MA, Tao Z, Menon S, Yang XP. Gender differences in cardiac function during early remodeling after acute myocardial infarction in mice. Life Sci 2004;75:2181–92
- Gao XM, Xu Q, Kiriazis H, et al. Mouse model of post-infarct ventricular rupture: time course, strain- and gender-dependency, tensile strength, and histopathology. Cardiovasc Res 2005;65:469–77