Abstract
Context: Since AMP-activated protein kinase (AMPK) activation in skeletal muscle of obese rodents stimulates fatty acid oxidation, it is reasonable to hypothesize that pharmacological activation of AMPK might be of therapeutic benefit in obesity.
Objective: To investigate the effects of the traditional Korean anti-obesity drug GGEx18, a mixture of three herbs, Laminaria japonica Aresch (Laminariaceae), Rheum palmatum L. (Polygonaceae), and Ephedra sinica Stapf (Ephedraceae), on obesity and the involvement of AMPK in this process.
Materials and methods: After high fat diet–induced obese mice were treated with GGEx18, we studied the effects of GGEx18 on body weight, fat mass, skeletal muscle lipid accumulation, and the expressions of AMPK, peroxisome proliferator-activated receptor ά (PPARα), and PPARα target genes. The effects of GGEx18 and/or the AMPK inhibitor compound C on lipid accumulation and expression of the above genes were measured in C2C12 skeletal muscle cells.
Results: Administration of GGEx18 to obese mice for 9 weeks significantly (p < 0.05) decreased body and adipose tissue weights compared with obese control mice (p < 0.05). Lipid accumulation in skeletal muscle was inhibited by GGEx18. GGEx18 significantly (p < 0.05) increased skeletal muscle mRNA levels of AMPKα1 and AMPKα2 as well as PPARα and its target genes. Consistent with the in vivo data, GGEx18 inhibited lipid accumulation, and similar activation of genes was observed in GGEx18-treated C2C12 cells. However, compound C inhibited these effects in C2C12 cells.
Discussion and conclusion: These results suggest that GGEx18 improves obesity through skeletal muscle AMPK and AMPK-stimulated expression of PPARα and its target enzymes for fatty acid oxidation.
Introduction
Over the last decade, AMP-activated protein kinase (AMPK) has been the subject of intense investigation and considerable pharmacological research because of the key roles that it has in regulating energy homeostasis. AMPK is a serine/threonine kinase that plays an important role in energy metabolism at both cellular and whole-body levels (CitationHardie et al., 1998; CitationKemp et al., 1999). In multiple mammalian tissues, AMPK controls glucose and lipid metabolism (CitationCarling, 2004; CitationHardie, 2008; CitationLong & Zierath, 2006). The activation of AMPK results in the phosphorylation of several target molecules and subsequent fatty acid oxidation in skeletal muscle, liver, adipose tissue, and heart, as well as glucose transport in muscle, cardiac glycolysis, and the inhibition of anabolic processes and ion channel activities (CitationHardie et al., 1998; CitationKemp et al., 1999). In particular, muscle AMPK activation induces gene expression of peroxisome proliferator-activated receptor ά (PPARα), which regulates the expression of genes critical for fatty acid oxidation (CitationLee et al., 2002, 2006; CitationSuzuki et al., 2007). PPARα target genes include those involved in hydrolysis of plasma triglycerides, fatty acid uptake, binding, and fatty acid β-oxidation, thereby leading to lipid homeostasis (CitationYoon, 2009, Citation2010). Therefore, it is suggested that obesity and obesity-related metabolic diseases may be inhibited by AMPK activators.
Gyeongshingangjeehwan 18 (GGEx18), an herbal drug composed of the three medicinal plants, Rheum palmatum L. (Polygonaceae), Laminaria japonica Aresch (Laminariaceae), and Ephedra sinica Stapf (Ephedraceae), has widely been used as one of the most valuable medicines in Korea. In particular, GGEx18 has already been used as an anti-obesity drug in local clinics of Korea, although the mechanism of its action remains unknown. These three herbs are reported to have anti-obesity, anti-diabetes, and lipid-lowering effects (CitationBoozer et al., 2001, Citation2002; CitationHuang et al., 2010; CitationXue et al., 2010; CitationYou et al., 2009; CitationZheng et al., 2010), supporting the belief that GGEx18 may regulate obesity and its related metabolic disorders. These reports led to the speculation of whether high fat diet–induced obesity in mice can be regulated more efficiently when treated with a mixture of the above three herbal extracts (GGEx18) at the same time. In our previous study, we found that GGEx18 seemed to be more effective compared with individual herbs with respect to their anti-obesity effects and safety (CitationYoon et al., 2010). We therefore hypothesized that GGEx18 can regulate obesity through AMPK and PPARα pathways in skeletal muscle.
We treated high fat diet–induced obese mice with GGEx18. We also treated mouse muscle cell line C2C12 with combinations of GGEx18 or compound C, a selective inhibitor of AMPK. Compound C causes AMPK suppression, which increases lipid accumulation (CitationGao et al., 2008; CitationLiu et al., 2011). We show that body weight, adipose tissue mass, and skeletal muscle lipid accumulation were significantly reduced in GGEx18-treated mice compared with controls. Concomitantly, GGEx18 treatment increased mRNA expression of AMPKα1 and AMPKα2 as well as PPARα and its target genes. Similar to the in vivo data, GGEx18 decreased triglyceride droplets, whereas it increased the gene expression of AMPK and PPARα pathways in C2C12 cells. However, these effects in C2C12 cells were reversed by compound C. These studies suggest that GGEx18 can prevent obesity by targeting skeletal muscle AMPK and PPARα pathways.
Materials and methods
Preparation of GGEx18
GGEx18 was prepared from food-grade aqueous extracts of the three herbs L. japonica (from the southern sea of Korea), E. sinica, and R. palmatum (Hwalim, Busan, Korea), and the composition of GGEx18 is shown in . The proportions used in this study are the same as those used to treat human patients. Voucher specimens for E. sinica (FOS-05-04), L. japonica (FOS-05-05), and R. palmatum (FOS-05-06) were deposited at the Department of Formula Sciences, Dongeui University. Briefly, three dried herbs with their contents weighted were boiled together in distilled water for 22 h at 95°C. The aqueous extracts were then filtered and freeze-dried under vacuum for the production of GGEx18.
Table 1. Composition of GGEx18.
Animal treatments
Eight-week-old male wild-type C57BL/6J mice (n = 8/group) were purchased from Central Lab Animal (Seoul, Korea) and randomly divided into five groups. Mice were fed a low-fat diet (normal group; 13% kcal fat, CJ, Incheon, Korea), a high-fat diet (control group; 45% kcal fat, Research Diets, New Brunswick, NJ), or the high-fat diet supplemented with three doses of GGEx18 (125, 250, and 500 mg/kg/day) for 9 weeks. In all experiments, body weights were measured daily using a top-loading balance and the person measuring the body weights was blinded to each treatment group. At the end of the study, mice were sacrificed under diethyl ether anaesthesia and skeletal muscle tissues were harvested, weighed, snap-frozen in liquid nitrogen, and stored at −80°C until use. Additional sections of gastrocnemius muscle were prepared for histological analyses. All animal experiments were approved by the Institutional Animal Care and Use Committee of Dongeui University and followed National Research Council Guidelines.
Histological analysis
Skeletal muscle tissues were embedded in a frozen section compound (Leica, Bannockburn, IL) and frozen in liquid nitrogen. Transverse and longitudinal sections (7 µm) were cut using a cryostat at −20°C and thawed on slides. Lipid accumulation in muscle tissue was assessed by oil red O staining. Briefly, the sections were incubated with 1,2-propanediol for 1 min at room temperature and the solution was removed. The sections were stained with 0.5% oil red O solution for 2 h at room temperature, incubated with 85% 1,2-propanediol for 1 min, and rinsed with deionized water. Morphological measurements for lipid accumulation were made under light microscopic examination.
Differentiation of C2C12 cells and analysis of lipid accumulation in C2C12 myotubes
Mouse myogenic C2C12 cells were routinely cultured in Dulbecco’s modified Eagle’s medium containing 10% fetal bovine serum (Invitrogen, Carlsbad, CA), penicillin G (100 U/mL), streptomycin sulfate (100 µg/mL), amphotericin B (0.25 µg/mL), and 2-mercaptoethanol (50 µM). To induce differentiation of skeletal muscle cell line C2C12, culture medium was changed to 2% horse serum instead of 10% fetal bovine serum when cells reached 70% confluence. The cells were differentiated into multinucleated myotubes for 5 days and then treated with chemicals for 24 h. The C2C12 myotubes were fixed in 10% phosphate-buffered formalin for 30 min and stained with oil red O for 1 h for microscopic analysis. The oil red O of stained lipid droplets was dissolved in isopropanol and quantified by measuring the absorbance at 520 nm.
Reverse transcription-polymerase chain reaction (RT-PCR)
Total cellular RNA from skeletal muscle tissues and differentiated C2C12 cells (C2C12 myotubes) was prepared using the Trizol reagent (Invitrogen). After 2 μg total RNA was reverse-transcribed using Moloney murine leukemia virus reverse transcriptase (MMLV-RT; Promega, Madison, WI) and an antisense primer, cDNA was generated. The RNA was denatured for 5 min at 72°C and immediately placed on ice for 5 min. Denatured RNA was mixed with MMLV-RT, MMLV-RT buffer, and a dNTP mixture, and incubated for 1 h at 42°C. Synthesized cDNA fragments were amplified by polymerase chain reaction (PCR) in an MJ Research Thermocycler (Waltham, MA). The PCR primers used for gene expression analysis are shown in . The cDNA was mixed with PCR primers, Taq DNA polymerase (Nanohelix, Daejon, Korea), and a dNTP mixture. The reaction consisted of 30 cycles of denaturation for 1 min at 94°C, annealing for 1 min at 58–60°C, and elongation for 1 min at 72°C. The PCR products were analyzed by electrophoresis on a 1% agarose gel. Relative expression levels are presented as a ratio of target gene cDNA vs. β-actin cDNA. PCR products were quantified from agarose gels using the GeneGenius (Syngene, Cambridge, UK).
Table 2. Sequences of primers used for the RT-PCR assays.
Statistical analysis
All values are expressed as the mean ± standard deviation (SD). Statistical analysis was performed by ANOVA followed by either Tukey’s multiple comparison or Dunnett’s post hoc tests. A p value < 0.05 was considered statistically significant.
Results
Effects of GGEx18 on body weight and adipose tissue mass in high fat diet–induced obese mice
To determine whether GGEx18 affects obesity in diet-induced obese mice, we measured body weight and body weight gain. Normal mice fed a low fat diet had a final body weight of 28.36 ± 0.9 g. After 9 weeks of high fat diet, the body weight of control mice was 37.54 ± 2.13 g (32% higher than that of normal mice). By contrast, the body weight of mice fed a high fat diet supplemented with 250 or 500 mg/kg/day GGEx18 was 32.31 ± 1.93 and 33.62 ± 1.43 g, respectively (13 and 10% lower than that of control mice, respectively) (). Similarly, GGEx18 significantly decreased total adipose tissue weight of control mice by 15, 37, and 36% at all doses (125, 250, and 500 mg/kg/day, respectively) of GGEx18 used in this study (). Thus, GGEx18 treatment significantly reduced body weight gain in high fat diet–induced obese mice. In addition, GGEx18 did not have any toxic effects on other organs.
Figure 1. GGEx18 inhibits high fat diet–induced body weight gain and total adipose tissue mass in C57BL/6J mice. Adult male C57BL/6 mice were fed a low-fat diet (normal), a high-fat diet (control), or the fat diet supplemented with 125, 250, and 500 mg/kg/day GGEx18 for 9 weeks. (A) Body weights at the end of the treatment period were statistically significant between normal and control groups (p < 0.05), and between the control group and the 250 mg/kg/day and 500 mg/kg/day GGEx18 groups (p < 0.05). (B) At the end of study, adipose tissue weights were measured. All values are expressed as the mean ± SD. #p < 0.05 compared with normal group, *p < 0.05 compared with control group. Normal, low fat diet-fed mice; control, high fat diet-fed mice; GGEx18, GGEx18-treated high fat diet-fed mice.
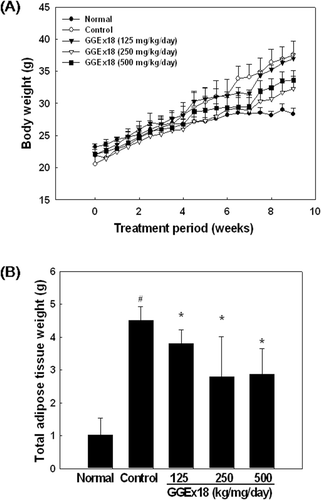
Effects of GGEx18 on lipid accumulation in skeletal muscle of high fat diet–induced obese mice
To determine the lipid accumulation in skeletal muscle, we examined cryostat sections of skeletal muscle by light microscopy after staining with oil red O. GGEx18 substantially reduced triglyceride droplets in skeletal muscle tissue. The reductions in both the number of stained fibers and the intensity of staining of the positive fibers were observed in the GGEx18-treated mice compared with controls (). Maximal reduction was achieved by GGEx18 treatment at a dose of 250 mg/kg/day. These results suggest that GGEx18 stimulates lipid catabolism in skeletal muscle.
Figure 2. GGEx18 inhibits high fat diet–induced increases in lipid accumulation in skeletal muscle of C57BL/6J mice. Adult male C57BL/6J mice were fed a low-fat diet (normal), a high-fat diet (control), or the high-fat diet supplemented with 125, 250, and 500 mg/kg/day for 9 weeks. (A) Histology showing transverse sections of skeletal muscle. (B) Higher magnitude of bracket area from (A). Shown are representative oil red O-stained sections (7-μm thick) of skeletal muscle. The number and intensity of stained fibers in the GGEx18 group were much less than that in the control group. Normal, low fat diet-fed mice; control, high fat diet-fed mice; GGEx18, GGEx18-treated high fat diet-fed mice.
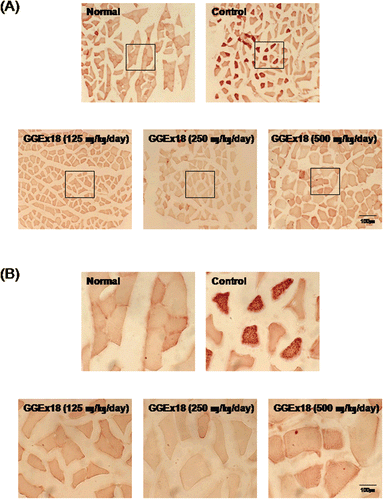
Effects of GGEx18 on skeletal muscle expression of AMPK, PPARα, and PPARα target genes in high fat diet–induced obese mice
To evaluate whether the inhibitory effects of GGEx18 on body weight gain and skeletal muscle lipid accumulation in obese mice are caused by changes in the expression of genes involved in AMPK-dependent PPARα activation in skeletal muscle, we first measured mRNA expression of AMPK, which induces lipid oxidation and thus lowers triglyceride storage of skeletal muscle (CitationCollier et al., 2006). However, obese control mice had lower mRNA levels of skeletal muscle AMPKα1 and AMPKα2 compared with lean normal mice. However, GGEx18 (250 mg/kg/day) significantly increased mRNA levels of the catalytic α1 and α2 subunits of AMPK by 96 and 111%, respectively, compared with control group. Expression of PPARα and its target genes, such as carnitine palmitoyltransferase 1 (CPT1), medium chain acyl-CoA dehydrogenase (MCAD), and very long chain acyl-CoA dehydrogenase (VLCAD), were also increased by GGEx18 treatment. Compared with controls, GGEx18-treated mice showed significant elevations in mRNA levels of PPARα, CPT1, MCAD, and VLCAD (101, 209, 45, and 159%, respectively) in skeletal muscle (). We also tested the effects of GGEx18 in obese mice on mRNA expression level of uncoupling protein 3 (UCP3), which has a PPAR response element in its promoter and is involved in the regulation of fatty acid oxidation. GGEx18 significantly increased the levels of UCP3 mRNA by 120% compared with those of control mice ().
Figure 3. GGEx18 increases the mRNA expression levels of AMPK, PPARα, and PPARα target genes in skeletal muscle of C57BL/6J mice. (A) Adult male C57BL/6J mice were fed a low-fat diet (Normal), a high-fat diet (Control), or the high-fat diet supplemented with 250 mg/kg/day GGEx18 for 9 weeks. Total cellular RNA was extracted from skeletal muscle tissue, and mRNA levels were measured using RT-PCR. All values are expressed as the mean ± SD of relative density units using β-actin as a reference. *p < 0.05 compared with control group. (B) Representative RT-PCR bands from one of the three independent experiments are shown. Control, high fat diet–fed mice; GGEx18, GGEx18 (50 mg/kg/day)-treated high fat diet–fed mice.
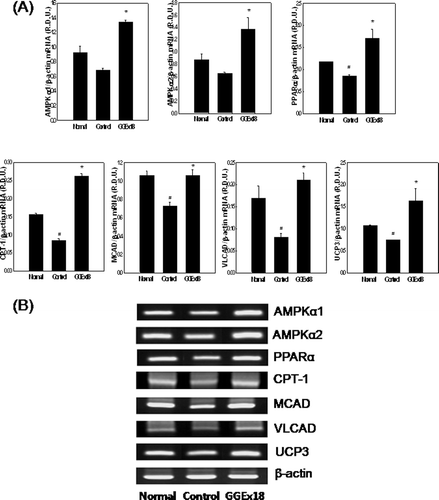
Effects of GGEx18 on lipid accumulation in C2C12 skeletal muscle cells
Accumulation of triglyceride droplets in differentiated C2C12 cells was evident, as shown by the increase in oil red O staining (). However, treatment with GGEx18 decreased lipid accumulation (). Similarly, the PPARα activators fenofibrate or Wy14,643 also decreased triglyceride droplets in C2C12 cells ( and ).
Figure 4. GGEx18 decreases triglyceride droplets in C2C12 cells. C2C12 cells were differentiated as described in the Materials and Methods section and triglycerides were stained with oil red O. (A) Non-differentiated cells (ND). Differentiated cells treated with (B) DMSO, (C) 10 μg/mL GGEx18, (D) 10 µM fenofibrate, and (E) 10 µM Wy14,643.
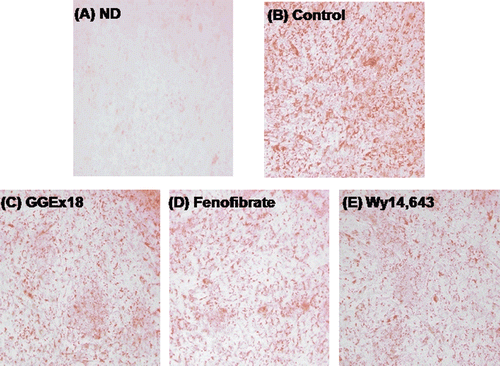
Effects of compound C on GGEx18-induced reduced lipid accumulation in C2C12 skeletal muscle cells
To demonstrate whether AMPK is involved in the GGEx18-mediated inhibition of lipid accumulation in differentiated C2C12 cells, cells were concomitantly treated with GGEx18 and the AMPK inhibitor compound C. Compound C treatment increased the GGEx18-associated reduction in triglyceride droplets (–). Triglyceride droplets were almost completely recovered to the levels of the control group after 1 or 10 μM compound C ( and ).
Figure 5. Compound C increases GGEx18-induced decreases in triglyceride droplets in C2C12 cells. C2C12 cells were differentiated as described in the Materials and Methods section and triglycerides were stained with oil red O. Differentiated cells treated with (A) DMSO, (B) 10 μg/mL GGEx18, (C) 10 μg/mL GGEx18 plus 0.1 μM compound C, (D) GGEx18 plus 1 μM compound C, and (E) GGEx18 plus 10 μM compound C. Comp C, compound C.
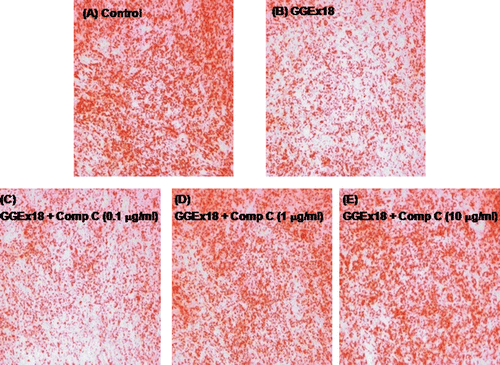
Effects of compound C on GGEx18-induced mRNA expression of AMPK, PPARα, and PPARα target genes in C2C12 skeletal muscle
Consistent with the effects of compound C on lipid accumulation in C2C12 cells, mRNA expression of AMPK, PPARα, and PPARα target genes, T were significantly increased by GGEx18, were decreased by concomitant treatment of GGEx18 and compound C. Treatment of C2C12 cells with GGEx18 increased mRNA expression of AMPKα1, AMPKα2, PPARα, and the PPARα target genes CPT-1, MCAD, VLCAD, and UCP3 by 15, 12, 34, 12, 46, 35, and 39%, respectively. However, compound C decreased these inductions by 22, 21, 33, 21, 39, 22, and 54%, respectively (). These results suggest that GGEx18 may increase fatty acid oxidation via a skeletal muscle AMPK-PPARα pathway, leading to the improvement of obesity.
Figure 6. Compound C decreases GGEx18-induced increases in mRNA expression levels of AMPK, PPARα, and PPARα target genes in C2C12 cells. C2C12 cells were differentiated as described in the Materials and Methods section. C2C12 cells were treated with DMSO, 10 μg/mL GGEx18, and 10 μg/mL GGEx18 plus 1 µM compound C. Total cellular RNA was extracted from differentiated cells and mRNA levels were measured using transcription-polymerase chain reaction (RT-PCR). All values are expressed as the mean ± SD of relative density units using β-actin as a reference. #p < 0.05 compared with DMSO group, **p < 0.05 compared with GGEx18 group. (B) Representative RT-PCR bands from one of the three independent experiments are shown.
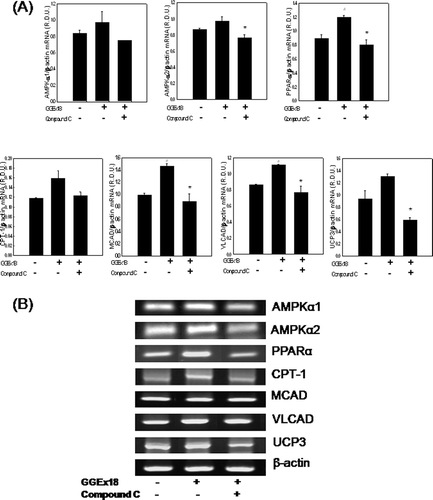
Discussion
AMPK has emerged in recent years as a major regulator of cell and whole-body metabolism. Numerous articles have reported evidence for its role in the regulation of appetite, body weight, and metabolism (CitationCarling, 2004; CitationHardie et al., 2006; CitationXue & KAhn, 2006), suggesting that AMPK is a major player in the development of obesity. GGEx18 is widely used as an anti-obesity drug in Korean local clinics, although the cellular and molecular mechanisms underlying its effects remain unknown. Therefore, we investigated the effects of GGEx18 on diet-induced obesity, as well as the involvement of AMPK in this process.
Our results demonstrate that GGEx18 decreased high fat diet–induced increases in body weight and adipose tissue mass in mice. High fat diet–fed mice had much higher body weights and fat mass compared with normal, low fat diet–fed mice. However, administration of GGEx18 to obese mice (250 and 500 mg/kg/day) for 9 weeks significantly reduced both body weight gain and total fat mass by 10–13% and 36–37%, respectively. Similarly, our human study also showed that 161 obese patients administered about 800–900 mg GGEx18 per day for 6 months had lower body weights and abdominal fat area compared with those before GGEx18 treatment (unpublished data). The herbs comprising GGEx18, R. palmatum (rhubarb), L. japonica (sea tangle), and E. sinica (ephedra), are reported to play a role in decreasing fat accumulation and body weight in animals and humans (CitationAhn et al., 2006; CitationBoozer et al., 2001, Citation2002; CitationYou et al., 2009; CitationZheng et al., 2010), suggesting that obesity can be prevented more efficiently when treated with a mixture of the three herbal extracts at the same time. Moreover, significant side effects were not observed in GGEx18-treated animals and human patients (CitationYoon et al., 2010). Although ephedra-containing dietary supplements and ephedrine have been reported to have toxicity (CitationFleming, 2008; CitationHaller and Benowitz, 2000), the contents of ephedrine of GGEx18 is low and its combination with sea tangle and rhubarb minimizes its toxicity. Thus, it seems that GGEx18 may be a safe and an effective anti-obesity drug.
Skeletal muscle is a principal tissue responsible for lipid uptake and use, and it is highly involved in the metabolic disturbances associated with lipid disorders, such as obesity and type 2 diabetes. Lipid oxidation is reduced in obese human skeletal muscle (CitationLópez-Soriano et al., 2006; CitationSimoneau et al., 1999). Markers of capacity to use fatty acids in skeletal muscle of obese individuals were lower than those in lean individuals, and fat contents within muscle have been found to be increased in obesity (CitationColberg et al., 1995; CitationPan et al., 1997; CitationSimoneau et al., 1999). We postulated that GGEx18 could regulate fat metabolism in skeletal muscle and analyzed fat content in the skeletal muscle of obese mice after GGEx18 treatment. Consistent with the effects of GGEx18 on weight reduction, we observed that GGEx18 inhibited lipid accumulation of skeletal muscle in high fat diet–fed obese mice. The reductions in both the number of stained fibers and the intensity of staining of the positive fibers were observed in GGEx18-treated mice compared with control mice. GGEx18 treatment also reduced lipid accumulation in C2C12 myotubes, to degrees which were comparable with fenofibrate and Wy14,643, activators of PPARα. Thus, it seems that GGEx18 treatment results in the lower deposition of skeletal muscle through increased fatty acid oxidation of skeletal muscle.
The AMPK pathway in skeletal muscle has been reported to have profound effects on the regulation of lipid metabolism. The ability of AMPK to induce lipid oxidation can thus lower skeletal muscle lipid deposition (CitationCollier et al., 2006; CitationLong & Zierath, 2006), maybe contributing to alleviation of obesity. AMPK activation stimulates mRNA expression of PPARα (CitationLee et al., 2006; CitationSuzuki et al., 2007), which regulates the expression of a number of genes critical for lipid metabolism, thereby leading to lipid homeostasis. We therefore examined whether this reduction in body weight, fat mass, and lipids in skeletal muscle was caused by increased expression of AMPK and AMPK-dependent PPARα in skeletal muscle of obese mice and C2C12 skeletal muscle cells. AMPKα1 and AMPKα2 mRNA levels were increased by GGEx18 treatment in both skeletal muscle tissue and cells. We then measured the AMPK downstream target PPARα and its target enzymes responsible for fatty acid β-oxidation in skeletal muscle and C2C12 cells in response to GGEx18. PPARα is known to initiate lipid-lowering effects through the transcriptional activation of mitochondrial and peroxisomal fatty acid-metabolizing enzymes (CitationAoyama et al., 1998; CitationJeong et al., 2004; CitationKroetz et al., 1998; CitationYan et al., 1998). In particular, fatty acid oxidation in skeletal muscle involves a rate-controlling step that is regulated by the PPARα target enzyme CPT-1 (CitationZarain-Herzberg & Rupp, 2002). CPT-1 transfers long-chain acyl-CoA into the mitochondria for its oxidation. Treatment of obese mice with GGEx18 significantly increased skeletal muscle mRNA levels of PPARα and CPT-1 as well as VLCAD, MCAD, and UCP3. Similar gene activation was observed in GGEx18-treated C2C12 skeletal muscle cells. These results suggest that GGEx18 stimulates fatty acid β-oxidation in skeletal muscle via AMPK-dependent PPARα activation. In addition, humans and rodents show great species differences in response to PPARα agonists. The predominant pathway of PPARα in mediating the effects of PPARα activators in rodents is known to be a peroxisomal β-oxidation system, while they act by increasing mitochondrial β-oxidation in humans (CitationYoon, 2009). Thus, our results suggest that GGEx18 can be used to treat human obesity due to its ability to increase the expression of mitochondrial β-oxidizing enzymes.
To confirm the involvement of AMPK in the above processes, we treated C2C12 cells with the AMPK inhibitor compound C. Compound C {6-[4-(2-piperidin-1-yl-etoxy)-phenyl]-3-pyridin-4-yl-pyrrazolo[1,5-a]pyrimidine} is a potent and highly selective inhibitor of AMPK that does not display significant inhibition of several structurally related kinases, including ZAPK, SYK, PKCq, PKA, and JAK3 (CitationZhou et al., 2001). Compound C treatment increased the GGEx18-mediated reduction in lipid accumulation. As expected, compound C decreased GGEx18-induced AMPKα1 and AMPKα2 mRNA levels in C2C12 cells. PPARα and its target genes for fatty acid oxidation were also significantly decreased by compound C. In parallel with this effect, 1 or 10 μM compound C markedly increased lipid accumulation to the levels of the control group. Thus, decreased AMPKα mRNA levels by compound C may inhibit PPARα activation in skeletal muscle. It is likely that GGEx18-induced fatty acid β-oxidation via the AMPK-PPARα pathway in skeletal muscle tissue may be one of the major factors leading to improved obesity.
In conclusion, these studies demonstrate that GGEx18 treatment increases the expression of AMPK, PPARα, and PPARα target genes involved in fatty acid β-oxidation in skeletal muscle of obese mice and C2C12 skeletal muscle cells. These changes led to reduced skeletal muscle fat deposition and improved obesity in obese animals. In addition to activation of AMPK in skeletal muscle, it is known that AMPK activation in liver and adipose tissue decreases lipid accumulation, whereas AMPK activation in hypothalamus increases appetite (CitationLong & Zierath, 2006). Further studies will be necessary not only to investigate the effects of GGEx18 on AMPK expression and activity in several organs including liver, adipose tissue, and hypothalamus but also to determine AMPK-mediated GGE18 functions for potential therapeutic implications. Since the anti-diabetic drugs rosiglitazone and metformin stimulate AMPK activity, GGEx18 may also be useful for treating metabolic diseases, such as insulin resistance and type 2 diabetes, in addition to obesity.
Acknowledgements
This work was supported by the National Research Foundation of Korea (NRF) Grant funded by the Korea Government (MEST) (NRF-2010-0027498 and NRF-2010-0017313).
Declaration of interest
The authors report no conflicts of interest. The authors alone are responsible for the content and writing of the paper.
References
- Ahn IS, Do MS, Choi BH, Kong CS, Kim SO, Han MS, Park KY. (2006). Reduced leptin secretion by fucoidan-added Kochujang and anti-adipogenic effect of fucoidan in mouse 3T3-L1 adipocytes. J Food Sci 11:31–35.
- Aoyama T, Peters JM, Iritani N, Nakajima T, Furihata K, Hashimoto T, Gonzalez FJ. (1998). Altered constitutive expression of fatty acid-metabolizing enzymes in mice lacking the peroxisome proliferator-activated receptor alpha (PPARalpha). J Biol Chem, 273, 5678–5684.
- Boozer CN, Daly PA, Homel P, Solomon JL, Blanchard D, Nasser JA, Strauss R, Meredith T. (2002). Herbal ephedra/caffeine for weight loss: a 6-month randomized safety and efficacy trial. Int J Obes Relat Metab Disord, 26, 593–604.
- Boozer CN, Nasser JA, Heymsfield SB, Wang V, Chen G, Solomon JL. (2001). An herbal supplement containing Ma Huang-Guarana for weight loss: a randomized, double-blind trial. Int J Obes Relat Metab Disord, 25, 316–324.
- Carling D. (2004). The AMP-activated protein kinase cascade–a unifying system for energy control. Trends Biochem Sci, 29, 18–24.
- Colberg SR, Simoneau JA, Thaete FL, Kelley DE. (1995). Skeletal muscle utilization of free fatty acids in women with visceral obesity. J Clin Invest, 95, 1846–1853.
- Collier CA, Bruce CR, Smith AC, Lopaschuk G, Dyck DJ. (2006). Metformin counters the insulin-induced suppression of fatty acid oxidation and stimulation of triacylglycerol storage in rodent skeletal muscle. Am J Physiol Endocrinol Metab, 291, E182–E189.
- Fleming RM. (2008). Safety of ephedra and related anorexic medications. Expert Opin Drug Saf, 7, 749–759.
- Gao Y, Zhou Y, Xu A, Wu D. (2008). Effects of an AMP-activated protein kinase inhibitor, compound C, on adipogenic differentiation of 3T3-L1 cells. Biol Pharm Bull, 31, 1716–1722.
- Haller CA, Benowitz NL. (2000). Adverse cardiovascular and central nervous system events associated with dietary supplements containing ephedra alkaloids. N Engl J Med, 343, 1833–1838.
- Hardie DG. (2008). AMPK: a key regulator of energy balance in the single cell and the whole organism. Int J Obes (Lond), 32 Suppl 4, S7–12.
- Hardie DG, Carling D, Carlson M. (1998). The AMP-activated/SNF1 protein kinase subfamily: metabolic sensors of the eukaryotic cell? Annu Rev Biochem, 67, 821–855.
- Hardie DG, Hawley SA, Scott JW. (2006). AMP-activated protein kinase–development of the energy sensor concept. J Physiol (Lond), 574, 7–15.
- Huang L, Wen K, Gao X, Liu Y. (2010). Hypolipidemic effect of fucoidan from Laminaria japonica in hyperlipidemic rats. Pharm Biol, 48, 422–426.
- Jeong S, Han M, Lee H, Kim M, Kim J, Nicol CJ, Kim BH, Choi JH, Nam KH, Oh GT, Yoon M. (2004). Effects of fenofibrate on high-fat diet-induced body weight gain and adiposity in female C57BL/6J mice. Metab Clin Exp, 53, 1284–1289.
- Kemp BE, Mitchelhill KI, Stapleton D, Michell BJ, Chen ZP, Witters LA. (1999). Dealing with energy demand: the AMP-activated protein kinase. Trends Biochem Sci, 24, 22–25.
- Kroetz DL, Yook P, Costet P, Bianchi P, Pineau T. (1998). Peroxisome proliferator-activated receptor alpha controls the hepatic CYP4A induction adaptive response to starvation and diabetes. J Biol Chem, 273, 31581–31589.
- Lee WJ, Kim M, Park HS, Kim HS, Jeon MJ, Oh KS, Koh EH, Won JC, Kim MS, Oh GT, Yoon M, Lee KU, Park JY. (2006). AMPK activation increases fatty acid oxidation in skeletal muscle by activating PPARalpha and PGC-1. Biochem Biophys Res Commun, 340, 291–295.
- Lee Y, Yu X, Gonzales F, Mangelsdorf DJ, Wang MY, Richardson C, Witters LA, Unger RH. (2002). PPAR alpha is necessary for the lipopenic action of hyperleptinemia on white adipose and liver tissue. Proc Natl Acad Sci USA, 99, 11848–11853.
- Liu JF, Ma Y, Wang Y, Du ZY, Shen JK, Peng HL. (2011). Reduction of lipid accumulation in HepG2 cells by luteolin is associated with activation of AMPK and mitigation of oxidative stress. Phytother Res, 25, 588–596.
- Long YC, Zierath JR. (2006). AMP-activated protein kinase signaling in metabolic regulation. J Clin Invest, 116, 1776–1783.
- López-Soriano J, Chiellini C, Maffei M, Grimaldi PA, Argilés JM. (2006). Roles of skeletal muscle and peroxisome proliferator-activated receptors in the development and treatment of obesity. Endocr Rev, 27, 318–329.
- Pan DA, Lillioja S, Kriketos AD, Milner MR, Baur LA, Bogardus C, Jenkins AB, Storlien LH. (1997). Skeletal muscle triglyceride levels are inversely related to insulin action. Diabetes, 46, 983–988.
- Simoneau JA, Veerkamp JH, Turcotte LP, Kelley DE. (1999). Markers of capacity to utilize fatty acids in human skeletal muscle: relation to insulin resistance and obesity and effects of weight loss. FASEB J, 13, 2051–2060.
- Suzuki A, Okamoto S, Lee S, Saito K, Shiuchi T, Minokoshi Y. (2007). Leptin stimulates fatty acid oxidation and peroxisome proliferator-activated receptor alpha gene expression in mouse C2C12 myoblasts by changing the subcellular localization of the alpha2 form of AMP-activated protein kinase. Mol Cell Biol, 27, 4317–4327.
- Xue B, Kahn BB. (2006). AMPK integrates nutrient and hormonal signals to regulate food intake and energy balance through effects in the hypothalamus and peripheral tissues. J Physiol (Lond), 574, 73–83.
- Xue J, Ding W, Liu Y. (2010). Anti-diabetic effects of emodin involved in the activation of PPARgamma on high-fat diet-fed and low dose of streptozotocin-induced diabetic mice. Fitoterapia, 81, 173–177.
- Yan ZH, Karam WG, Staudinger JL, Medvedev A, Ghanayem BI, Jetten AM. (1998). Regulation of peroxisome proliferator-activated receptor alpha-induced transactivation by the nuclear orphan receptor TAK1/TR4. J Biol Chem, 273, 10948–10957.
- Yoon M. (2009). The role of PPARalpha in lipid metabolism and obesity: focusing on the effects of estrogen on PPARalpha actions. Pharmacol Res, 60, 151–159.
- Yoon M. (2010). PPARa in obesity: sex difference and estrogen involvement. PPAR Res, 2010, 584296.
- Yoon KH, Lee HY, Jung YS, Seo BI, Park KY, Yoon M, Shin SS. (2010). Modulation of obesity by Gyeongshingangjeehwan 18 in ob/ob mice. Kor J Herbology 25:1–9.
- You JS, Sung MJ, Chang KJ. (2009). Evaluation of 8-week body weight control program including sea tangle (Laminaria japonica) supplementation in Korean female college students. Nutr Res Pract, 3, 307–314.
- Zarain-Herzberg A, Rupp H. (2002). Therapeutic potential of CPT I inhibitors: cardiac gene transcription as a target. Expert Opin Investig Drugs, 11, 345–356.
- Zheng CD, Duan YQ, Gao JM, Ruan ZG. (2010). Screening for anti-lipase properties of 37 traditional Chinese medicinal herbs. J Chin Med Assoc, 73, 319–324.
- Zhou G, Myers R, Li Y, Chen Y, Shen X, Fenyk-Melody J, Wu M, Ventre J, Doebber T, Fujii N, Musi N, Hirshman MF, Goodyear LJ, Moller DE. (2001). Role of AMP-activated protein kinase in mechanism of metformin action. J Clin Invest, 108, 1167–1174.