Abstract
Context: Docosahexaenoic acid (DHA) is one of the critical fatty acids for optimal health, which affect the expression of nerve growth factor and brain-derived neurotrophic factor in brain.
Objective: This study investigates whether DHA supplementation affects lipid peroxidation and activates the glial-derived neurotrophic factor (GDNF)-mitogen-activated protein kinase pathway (MAPK pathway) in hippocampus of natural aged rat.
Materials and methods: Rats were randomly divided into four groups; DHA was orally administered at 80 and 160 mg/kg/day to 24-month female rats for 50 days. The antioxidant parameters and GDNF-GDNF family receptor α-1 (GFRα1)-tyrosine-protein kinase receptor (RET)-MAPK-cyclic AMP response element-binding protein (CERB) pathway were assayed in natural aged rat’s hippocampus.
Results and discussion: The results demonstrated that DHA supplementation significantly increased the activities of superoxide dismutase (SOD) by 37.39 and 57.69%, glutathione peroxidase (GSH-Px) by 27.62 and 32.57% decreased TBARS level by 28.49 and 49.05%, respectively, but did not significantly affect catalase (CAT), in hippocampus, when compared with the aged group. DHA supplementation in diet resulted in an increase of DHA level in hippocampus. Furthermore, we found that DHA supplementation markedly increased the levels of GDNF and GFRα1 and the phosphorylation of RET, and led to the activation of the MAPK pathway in hippocampus tissue.
Conclusion: DHA supplementation can change fatty acids composition, improve antioxidant parameters and activate the GDNF-MAPK pathway in natural aged rat’s hippocampus.
Introduction
Docosahexaenoic acid (DHA), one of the predominant polyunsaturated fatty acids in brain, is essential for normal neural development (Innis, Citation2000). Previous studies have shown that increased DHA intake could affect brain functions as learning ability and emotion (Catalan et al., Citation2002; Lim & Suzuki, Citation2002). The efficacy of DHA is age-dependent, while DHA deficiency is associated with cognitive decline, enhancing free radical-mediated lipid peroxidation (McGahon et al., Citation1999; Montine et al., Citation2002; Nourooz-Zadeh et al., Citation1999). Oxidative damage plays a key role in the aging process, including cognitive decline (Kiray et al., Citation2004). Hence, the study of whether DHA supplementation affects lipid peroxidation is very important for elucidating DHA function in aging brain. Neurotrophic factors are important for the aging brain. Chronic neurological diseases as well as acute brain injuries may be cured with neurotrophic factors such as nerve growth factor, brain-derived neurotrophic factor and glial-derived neurotrophic factor (GDNF; Rangasamy et al., Citation2010). However, poor penetration of the blood–brain barrier and the side effects hinder the applications of these factors in neural therapy. Therefore, stimulating the endogenic production of trophic factors seems to be a more attractive approach (Ciccarelli et al., Citation2001).
Previous studies have shown that an increase in brain DHA level increased the levels of nerve growth factor and brain-derived neurotrophic factor (Acsády et al., Citation2000; Jiang et al., Citation2009a,Citationb, Citation2012). Tanriover et al. (Citation2010) reported that DHA supplement resulted in an increase of GDNF in rat suffering with Parkinson’s disease. GDNF is very important for brain function. Infusion of GDNF in the brain of old monkeys and mice could attenuate motor and cognitive deficits produced by dopaminergic and cholinergic system dysfunctions (Fischer et al., Citation1987; Grondin et al., Citation2003; Hebert & Gerhardt, 1987; Kirik et al., Citation2004). GDNF exerts its biological action via a two-component receptor complex consist of Ret receptor and GFRα1, which are glycosylphosphatidylinositol-anchored cell surface molecule (Jing et al., Citation1996). GDNF activates RET phosphorylation and then results in activation of the mitogen-activated protein kinase (MAPK) signal transduction cascade (Creedon et al., Citation1997).
Cyclic AMP response element-binding protein (CREB) functions as a transcription factor under physiological conditions through the convergence of multiple cellular signaling cascades that include increased intracellular cAMP following stimulation of G-protein coupled receptors; increased intracellular calcium through activation of voltage- or ligand-gated ion channels or the activation of neurotrophic receptor tyrosine kinases. Constitutively expressed throughout the brain, CREB is localized within the nucleus where it dimerizes and binds to the DNA cAMP response element. Transcriptional activation occurs through phosphorylation of CREB that serves as a substrate to the numerous upstream cascade pathways, many of which are thought to be involved in cognitive processing. Evidence now indicates that CREB activation represents a key stimulus-transcription coupling resulting in the translation-expression of gene involved in neuronal, i.e., synaptic plasticity required for long-term memory in response to environmental learning cues (Scott Bitner, Citation2012).
Considering the hippocampus plays an important role in cognitive ability and for better understanding the biochemical function of DHA in hippocampus, the present study dissects the role of DHA in the GDNF-GFRα1-RET-MAPK-CERB pathway in natural aged rat’s hippocampus. The study provides basic and useful information on the role of DHA in cognation during aging.
Materials and methods
Reagents and chemicals
DHA from cod liver oil (Sigma Chemical Co., St. Louis, MO; purity >90%); SOD, CAT, GSH-Px, CAT and TBARS kits were commercially available (Jianchen Bioengineering, Nanjing, Jiangshu Province, P.R. China); GDNF ELISA kit (Emax Immunoassay System kit; Promega, Madison, WI); RNA Trizol® Reagent (Invitrogen); cDNA Synthesis Kit (Invitrogen, Carlsbad, CA); PVDF membranes (BioRad, Hercules, CA); BCA assays (Pierce, Rockford, IL); Rabbit polyclonal anti-GFRα1 antibody (1:1000; Santa Cruz Biotechnology, Santa Cruz, CA); Ret phosphorylated Y 1062 antibody (1:700; Santa Cruz); Total Ret (1:700, Santa Cruz); anti-Phospho-c-Raf (Ser338) antibody (1:500; Cell Signaling Technology, Beverly, MA); Total anti-Raf antibody(1:400; Cell Signaling Technology); anti-phospho-Mek1/2 antibody (1:500; Cell Signaling Technology); Total Mek1/2 antibody (1:200, Cell Signaling Technology); Mouse anti-phospho Erk1/2 Thr202/Tyr 204 (1;700; Cell Signaling Technology); Total Erk1/2 antibody (1:200; Cell Signaling Technology); P-CERB(1:500; Cell Signaling Technology); β-actin (1:5000; Sigma, St. Louis, MO); secondary peroxidase-coupled anti-rabbit (1:5000; Santa Cruz Biotechnology); chemiluminescence with Super-Signal (Pierce, Appleton, WI). All regents and chemicals used were of analytical grade.
Animal treatment
Adult Sprague–Dawley female rats (5-month-old, 250 ± 20 g/body weight) and aged Sprague–Dawley female rats (24-month old, 530 ± 20 g/body weight) were purchased from Animal Experimental Center of Guangxi (Nanning, China). The rats were housed in an environment with temperature maintained at 23 ± 2 °C and humidity at 42 ± 2% under a 12:12 h light/dark cycle, with free access to water and food. The laboratory animals approved by the Animal Ethics Committee of Guangxi University and were treated according to the guidelines of laboratory animals.
After a week of adaptation, rats were randomly divided into four groups. Adult group, 5-month-old female rats (n = 20); aged group, 24-month aged female rats (n = 20); lower dose group (n = 20), 24-month female aged rats treated with DHA 80 mg/kg body weight/day; large dose group (n = 20), 24-month female aged rats treated with DHA 160 mg/kg body weight/day. DHA was administered via oral gavage for 50 successive days. DHA was dissolved in 5% gum arabic solution, pipetted into light-protecting glass vials under a nitrogen gas flow intended to diminish their auto-oxidation and stored at −80 °C. In order to eliminate the effects of daily gavages, adult group and aged group were received a similar volume of 5% gum arabic solution, respectively. The fatty acids compositions of diet are shown in .
Table 1. The comparison of fatty acids in diet and total fatty acids is about 6.8 % in diet.
Fatty acid determination in hippocampus tissue
The rats were euthanized and decapitated, and hippocampus was collected and frozen at −80 °C for subsequent biochemical analysis. The lipid of tissue was extracted using a modified method from Bligh and Dyer (Citation1959). Each sample was analyzed for the individual fatty acid by gas chromatography (Lipid Standards, FAMEs, Sigma Co., St. Louis, MO).
Measurement of antioxidant parameters in hippocampus
Hippocampus was homogenized in 1/5 (w/v) 50 mM (pH 7.4) ice-cold phosphate buffer saline solution containing a protease inhibitor cocktail (Sigma-Aldrich, St. Louis, MO) with 10 strokes at 5000 g in a Potter homogenizer. Protein concentration was determined according to Lowry et al. (Citation1951).
SOD activity assay
The principle of this assay is based on the oxidation of nicotinamide adenine dinucleotide phosphate mediated by the super oxide radical using commercially available kits. The assay for SOD was based on its ability to inhibit the oxidation of oxymine by the xanthine–xanthine oxidase system (Oyanagui, Citation1984). The hydroxylamine nitrite produced by the oxidation of oxymine had an absorbance peak at 550 nm. One unit (U) of SOD activity was defined as the amount that reduced the absorbance at 550 nm by 50%. These data are expressed as units per microgram protein.
GSH-Px activity assay
The activity of GSH-Px was determined by quantifying the rate of oxidation of the reduced glutathione to the oxidized glutathione by H2O2. Potassium phosphate (50 mM, pH 7.0), 1 mM sodium azide, 2 mM GSH, 0.2 mM NADPH, 1 U/ml GSSG-R, 1.5 mM cumene hydroperoxide and 100 μl of sample were used as the reaction mixture (1 ml), which was incubated at 25 °C for 5 min. Cumene hydroperoxide was added to initiate the reaction. The kinetic change was spectrophotometrically recorded at 340 nm (25 °C) for 3 min. GSH-Px activity was defined as units (micromoles of oxidized NADPH per minute) per milligram of protein, whereas 1 unit (U) of GSH-Px was defined as the amount that reduced the level of GSH by 1 μmol/l in 1 min per milligram of hippocampus protein.
CAT activity assay
The reaction mixture (1 ml) consisted of 50 mM potassium phosphate (pH 7.0), 19 mM H2O2 and 20–50 μl of sample. The reaction was initiated by the addition of H2O2 and absorbance changes were measured at 240 nm (25 °C) for 30 s. The molar extinction coefficient for H2O2 is 43.6 M−1 cm−1. The CAT activity unit is defined as micromoles of H2O2 consumed per minute per milligram protein.
Thiobarbituric acid reactive substances assay
Thiobarbituric acid reactive substance (TBARS) levels were measured by a fluorometric method using 1,1,3,3- tetramethoxypropane. Tissue samples (50 μl) were placed into a tube containing 1 ml of distilled water. After the addition of 1 ml 29 mM 2-thiobarbituric acid in acetic acid (8.75 M), samples were placed in a water bath and heated for 1 h at 95–100 °C. After cooling the samples, 25 μl of 5 M HCl was added and the reaction mixture was extracted by agitation for 5 min with 3.5 ml of n-butanol. Following centrifugation, the butanol phase was separated and fluorescence of the butanol extract was measured in a spectrofluorometer using wavelengths of 525 nm for excitation and 547 nm for emission, and the results were expressed as nanomoles of TBARS per mg protein.
Quantitative analysis of GDNF protein levels by enzyme-linked immunosorbent assay
The concentration of GDNF was examined using the enzyme linked immunosorbent assay. All samples were tested in triplicate and the mean was calculated. The intra- and inter-assay coefficients of variation were <4% and <5%, respectively. The values obtained from absorbance reader were expressed as pg/mg wet weight of tissue.
Real time-PCR analysis of GFRα1 gene expression
Total RNA of hippocampal tissue was extracted from tissue, according to the manufacturer’s instructions. After deoxyribonuclease (DNase) treatment, 1 μg of the total RNA was converted into complementary DNA (cDNA) with a M-MuLV First Strand cDNA Synthesis Kit. The primers for GFRα1 mRNA (137 bp) was designed based on published sequences (NM_012959.1): Sense 5′-CAGGCAGTTCTTCGACAAGGTT-3′. Antisense: 5′-TGGGCCTCTCTCGTTCTTCA-3′.
The primers for GAPDH mRNA (203 bp) was designed based on published sequences (XR_086293.2): Sense 5′-AAGAAGGTGGTGAAGCAGGC-3′. Antisense: 5′- TCCACCACCCTGTTGCTGTA-3′.
The real-time quantitative PCR was performed using Real-Time PCR Systems (Applied Biosystems, Alameda, CA) starting with an initial step at 95 °C for 10 s, followed by 40 cycles of denaturation at 95 °C for 15 s and annealing/extension at 60 °C for 1 min. To confirm the amplification of the desired PCR products by melting curve analysis, each complete amplification step was followed by a consecutive melting cycle from 95 °C to 60 °C and then to 95 °C (at the rate of 0.03 °C/s).
The 2−ΔΔCT method of relative quantification was used to determine the fold change in expression. This was done by first normalizing the resulting threshold cycle (CT) of the target mRNAs to the CT values of the internal control β-actin in the same samples. In each test, the ΔΔCT and ratio were determined as following ΔCT(control sample) = CT(target gene) − CT(reference gene), ΔCT(test sample) = CT(target gene) − CT(reference). It was further normalized with the control (ΔΔCT = ΔCT(control sample) − ΔCT(test sample)), Ratio = 2−ΔΔCT.
Western blotting analyses of protein expression
For western blot analysis, the total protein from hippocampus tissue was quantified by BCA assays and 1× protease inhibitor cocktail. Proteins were diluted in a denaturing solution and boiled for 3 min. Proteins (50 μg) were run per lane on 7% SDS-PAGE gels and transferred onto PVDF membranes. Membrane was washed with PBS, blocked with 5% non-fat milk/TBST (0.05% Tween-20, TBS) for 1 h, and incubated in the presence of the following polyclonal antibodies: rabbit polyclonal anti-GFRα1 antibody (1:1000), Ret phosphorylated Y 1062 antibody (1:700), total Ret (1:700), anti-Phospho-c-Raf (Ser338) antibody, (1:500), total anti-Raf antibody (1: 400), anti-pMEK1/2 antibody (1:500), total MEK1/2 antibody (1:200), mouse anti-phospho Erk1/2 Thr202/Tyr 204 (1:700), total Erk1/2 antibody (1:200), P-CERB (1:500), β-actin (1:5,000) and a monoclonal antibody against antibodies were secondary peroxidase-coupled anti-rabbit (1:5000). Proteins were revealed using the enhanced chemiluminescence with Super-Signal. As a control for protein loading and transfer, membranes were stripped, incubated with the monoclonal antibody against β-actin. Images from films were digitally acquired and analyzed with a ChemiDoc System (BioRad) and densitometry analysis performed using the Quantity One Software (BioRad Labs., Hercules, CA ).
Statistical analysis
Results are expressed as the means ± SEM. Statistical analysis was performed using the SPSS program (version 17.0, SPSS, Chicago, IL). Statistical analysis included one-way analysis of variance, and inter-group comparisons were made using Tukey’s multiple comparison test. The level of significance set at p < 0.05 was considered significant: p < 0.05, p < 0.01 versus adult group; p < 0.05, p < 0.01 versus aged group.
Results
Effects of DHA on fatty acid composition in hippocampus tissue
Fatty acid composition in hippocampus is shown in . The percentage of saturated fatty acids and monounsaturated fatty acids were increased in the aged group and adult group. The percentage of saturated fatty acids was decreased between DHA supplementation groups and aged group.
Table 2. The effects of DHA on fatty acid composition in hippocampus tissues (% of total).
DHA level was significantly decreased in the aged group in comparison with the adult group; DHA level was significantly increased in hippocampus by DHA supplementation. Moreover, there was a significant increase in the ratio of DHA: AA. DHA supplementation can increase the ration of DHA: AA from 1.28 to 1.65 in the aged group and 1.74 folds in DHA-treated groups. Furthermore, DHA supplementation increased the ratio of n − 3/n − 6 (polyunsaturated fatty acids) from 0.83 to 1.03 in the aged group and 1.10 folds in DHA supplementation groups, respectively.
Effects of DHA on SOD, GSH-Px, CAT and TBARS in hippocampus tissue
Results were summarized in . In the aged group, SOD, CAT and GSH-Px activities were significantly decreased and TBARS level was significantly increased, when compared with the adult group. In DHA supplementation groups, SOD activity was significantly increased by 37.39 and 57.69%; GSH-Px activity was significantly increased by 27.62 and 32.57%, respectively, when compared with the aged group. However, CAT activity was not significantly increased in DHA supplementation groups. TBARS level in DHA supplementation groups was decreased to 28.49 and 49.05%, respectively, in hippocampus, when compared with the aged group.
Table 3. The effects of DHA on superoxide dismutase (SOD), glutathione peroxidase (GSH-Px), catalase (CAT) and thiobarbituric acid reactive substances (TBARS) in hippocampus tissues.
DHA supplementation increased the expression of GDNF, GFRα1, RET, Raf-MEK-ERK-CERB phosphorylation in hippocampus tissue
As shown in , the level of GDNF protein in the hippocampus of aged group was significantly decreased when compared with the adult group. The levels of GDNF protein in DHA supplementation groups were significantly increased when compared with the aged group. In hippocampus tissue, the levels of GDNF in DHA-treated groups were significantly increased by 23.91 and 45.54%, respectively, when compared with the aged group.
Table 4. Effect of DHA on GDNF levels in hippocampus tissues.
In , the result showed the levels of GFRα1 mRNA and protein in hippocampus. The levels of GFRα1 mRNA in hippocampus tissue of DHA supplementation groups were significantly increased when compared with the aged group. The level of GFRα1 protein in the hippocampus of aged group was significantly decreased when compared with the adult group. Compared with the aged rats, DHA supplementation significantly increased the mRNA and protein level expression of GFRα1 in hippocampus.
Figure 1. GFRa1 mRNA and protein level in the hippocampus tissue. Rat treatment with 80 mg/kg/day and 160 mg/kg/day DHA 50 days significantly influence the expression of GFRa1 in hippocampus tissue. Summary of the results derived from real-time RT-PCR. GFRa1 mRNA levels were first normalized to GAPDH, calculated by the 2–ΔΔCT method. Relative expression units were determined by dividing the apparent levels of by the expression level of adult group. Compared with the aged rats, the treatment with 80 mg/kg/day and 160 mg/kg/day DHA 50 days significantly increased the protein expression of GFRα1. The quantity of the applied protein was normalized by Western blotting analysis with anti-actin polyclonal (n = 5). aap < 0.05 versus aged group. bbp < 0.01, DHA treatment group versus aged group. n = 5 in each group.
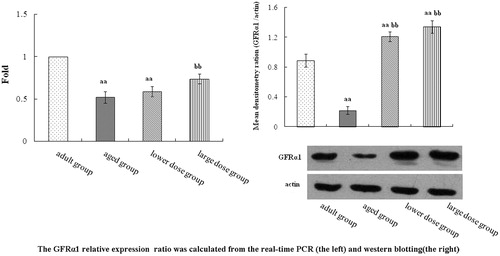
shows the results of the Western blotting analysis of Ret level in hippocampus tissue. The result showed that the level of RET in hippocampus in the aged group was significantly decreased when compared with the adult group. Compared with the aged rats, DHA supplementation significantly increased the protein expression of Ret phosphorylation in the hippocampus of aged group.
Figure 2. Western blot analysis of phosphorylation of receptor tyrosine kinase RET expression in the hippocampus tissue. Compared with aged rats treatment with 80 mg/kg/day and 160 mg/kg/day DHA 50 days significantly increased the protein expression of phosphorylation of receptor tyrosine kinase RET. The quantity of the applied protein was normalized by Western analysis with anti-RET (n = 5). aap < 0.05 versus aged group. bbp < 0.01, DHA treatment group versus aged group. n = 5 in each group.
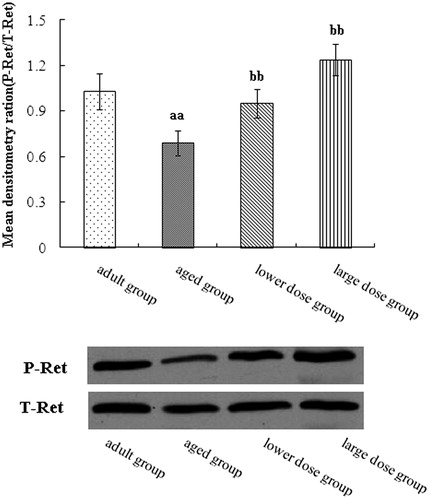
We used Western blots to assay MAPK pathway. The result showed that the Raf, MEK and ERK phosphorylation levels in the hippocampus of aged group was significantly decreased when compared with the adult group. Compared with the aged rats, DHA supplementation significantly increased the protein expression and phosphorylation levels of Raf, MEK and ERK. The results are shown in .
Figure 3. Western blot analysis of phosphorylation Raf expression in hippocampus tissue. Compared with aged rats, treatment with 80 mg/kg/day and 160 mg/kg/day DHA 50 days significantly increased the levels protein expression of phosphorylation of Raf. The quantity of the applied protein was normalized by Western analysis with anti-Raf (n = 5). aap < 0.05 versus aged group. bbp < 0.01, DHA treatment group versus aged group. n = 5 in each group.
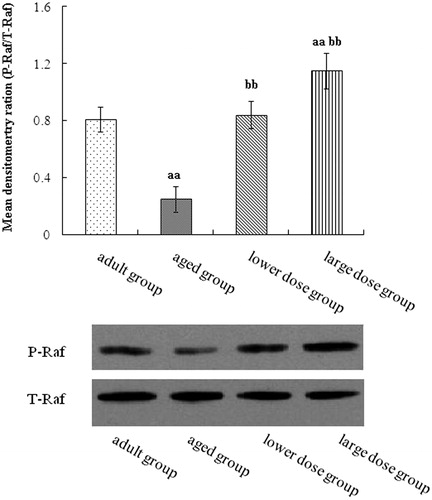
Figure 4. Western blot analysis of phosphorylation MEK expression in hippocampus tissue. Compared with aged rats, treatment with 80 mg/kg/day and 160 mg/kg/day DHA 50 days significantly increased the levels protein expression of phosphorylation of MEK. The quantity of the applied protein was normalized by Western analysis with anti-total MEK (n = 5). aap < 0.05 versus aged group. bbp < 0.01, DHA treatment group versus aged group. n = 5 in each group.
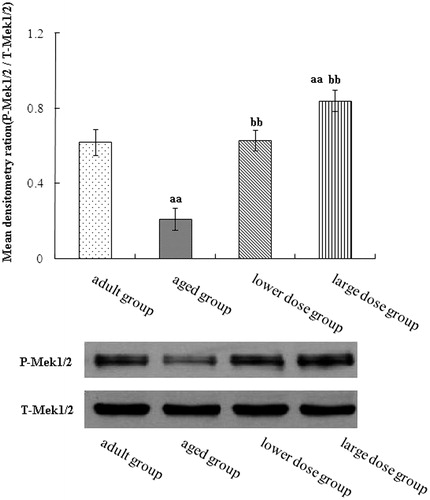
Figure 5. Western blot analysis of phosphorylation ERK expression in hippocampus tissue. Compared with aged rats, treatment with 80 mg/kg/day and 160 mg/kg/day DHA 50 days significantly increased the levels protein expression of phosphorylation of EEK. The quantity of the applied protein was normalized by Western analysis with anti-total ERK (n = 5). aap < 0.05 versus aged group. bbp < 0.01, DHA treatment group versus aged group. n = 5 in each group.
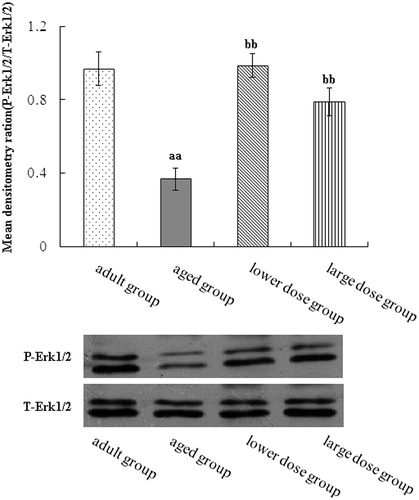
Figure 6. Western blot analysis of phosphorylation CERB expression in hippocampus tissue. Compared with aged rats, treatment with 80 mg/kg/day and 160 mg/kg/day DHA 50 days significantly increased the levels protein expression of phosphorylation of CERB. The quantity of the applied protein was normalized by Western analysis with actin. aap < 0.05 versus aged group. bb p < 0.01, DHA treatment group versus aged group. n = 5 in each group.
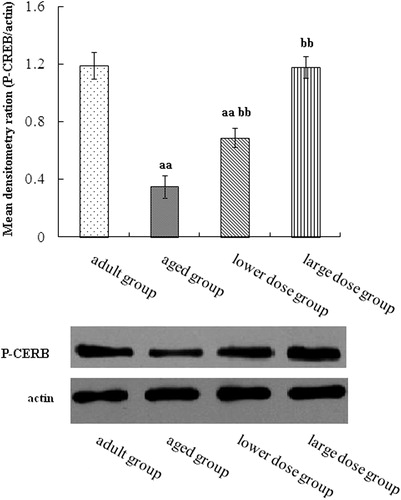
Discussion
In this study, the results showed that DHA supplementation increased total n − 3 and n − 6 polyunsaturated fatty acid and the ratio of n − 3/n − 6 (polyunsaturated fatty acids) and DHA/AA in natural aged rat’s hippocampus which may be the basic function DHA in natural aged rat’s hippocampus.
Reactive oxygen species become an active field in aging research because of their potential involvement in many degenerative processes and in many neurodegenerative diseases such as Alzheimer’s and Parkinson’s diseases (Tabner et al., Citation2001). These reactive oxygen species are scavenged by endogenous antioxidants including SOD and GSH-Px. TBARS are by-product of lipid peroxidation induced by free radicals and widely used as a biomarker of oxidative stress (Mori et al., Citation2003). We found that DHA not only decreased TBARS level but also increased SOD and GSH-Px activities in natural aged rat’s hippocampus tissue. Therefore, we speculate that DHA can protect brain from lipid peroxidation. Our findings indicate that dietary DHA supplementation decreases lipid peroxidation in natural aged rat’s hippocampus. A number of reports have also shown a very pronounced inhibition of TBARS generation with dietary DHA supplementation (Hossain et al., Citation1999; Song & Miyazawa, Citation2001; Songur et al., Citation2004; Wu et al., Citation2004). Moreover, DHA supplementation can increase the ratio of n − 3/n − 6 polyunsaturated fatty acid, implying somewhat the relationship between the ratio of n − 3/n − 6 and antioxidative defense.
GDNF has a neurorestorative property, which makes it a potential therapeutic agent for neurodegenerative disorders (Airaksinen & Saarma, Citation2002; Gash et al., Citation1996). Chao and Lee (Citation1999) have demonstrated that one of the cellular mechanisms underlying the neuroprotective action of GDNF is through activation of the antioxidant enzyme systems in rat striatum. The toxic effects of a variety of reactive oxygen species generating compounds on cultured primary neurons or a neuronal cell line were completely prevented by neurotrophic factors, such as GDNF and BDNF (Gong et al., Citation1999; Mena et al., Citation1997). On the other hand, eicosapentaenoic acid can be efficiently converted to DHA by increasing the DHA content in glial cells (Kawashima et al., Citation2010). Therefore, we can conclude that DHA has antioxidant ability, partially through GDNF and DHA may significantly increase the number of glial cells or protect glial cells from free radical oxidative, and eventually lead to an increase of GDNF level in the aged process.
The absence of GDNF, GFRα1 and Ret do not associate with each other and Ret is not present in lipid rafts. To activate RET in a more controlled, ligand-dependent manner, both GDNF and GFRα1 were required (Hebert & Gerhardt, Citation1997). GDNF can trigger RET phosphorylation in cooperatively with GFRα1 and induce intracellular signaling (Hebert & Gerhardt, Citation1997; Tansey et al; Citation2000). Activation of RET induces signaling through MAPK pathway to ERK, which initiate various functions in cells (Treanor et al., Citation1996). Our experimental results showed that DHA increased the levels of GFRα1 mRNA and protein GFRα1 and the RET’s phosphorylation.
Raf-MEK-ERK (MAPK) signaling pathway was measured, the present data showed that the levels phosphorylation levels of Raf, MEK and ERK were increased in natural aged rat’s hippocampus by DHA supplementation. Activated Ras binds to the Raf serine/threonine kinases and recruits them to the cell membrane. Activated Raf is phosphorylated and activates MEK, and then MEK phosphorylates and activates ERK. The Raf-MEK-ERK cascade is scaffolded by the kinase suppressor of Ras. Activated ERK has many substrates in the cytosol and can enter the cell nucleus to regulate the gene expression by phosphorylating transcription factors such as CERB (Peng et al., Citation2010). Our study further proved that the level of phosphorylation of CERB was increased by the DHA supplement. However, these experiments do not rule out other pathways for GDNF-RET signaling, but do demonstrate that the MAPK is one of pathways that RET signals are transmitted to the cell nucleus. It means that DHA can improve the GDNF pathway in natural aged rat’s hippocampus.
In conclusion, our work demonstrated that DHA can reduce the lipid peroxidation in natural aged rats’ hippocampus. Moreover, DHA supplementation can upregulate GDNF and GFRα1 and regulate RET, MAPK phosphorylation and ERK phosphorylation. It implied that DHA activates CERB through the MAPK pathway, at least partly, in aged rats’ hippocampus. It means that DHA can protect brain in the natural aging process. However, the molecular mechanism of DHA on GDNF pathway in natural aging process still requires further study.
Declaration of interest
This research was funded by Guangxi University doctoral Project fund (NO. X090015). The authors report no conflicts of interest. The authors alone are responsible for the content and writing of this article.
References
- Acsády L, Pascual M, Rocamora N, et al. (2000). Nerve growth factor but not neurotrophin-3 is synthesized by hippocampal GABAergic neurons that project to the medial septum. J Neurosci 98:23–31
- Airaksinen MS, Saarma M. (2002). The GDNF family: Signaling, biological functions and therapeutic value. Nat Rev Neurosci 3:383–94
- Bligh EG, Dyer W. (1959). A rapid method of total lipid extraction and purification. Can J Biochem Physiol 37:911–17
- Catalan J, Moriguchi T, Slotnick B, et al. (2002). Cognitive deficits in docosahexaenoic acid-deficient rats. Behav Neurosci 116:1022–31
- Chao CC, Lee EH. (1999). Neuroprotective mechanism of glial cell line-derived neurotrophic factor on dopamine neurons: Role of antioxidation. Neuropharmacology 38:913–16
- Ciccarelli R, Ballerini P, Sabatino G, et al. (2001). Involvement of astrocytes in purine-mediated reparative processes in the brain. Int J Dev Neurosci 19:395–414
- Creedon DJ, Tansey MG, Baloh RH, et al. (1997). Neurturin shares receptors and signal transduction pathways with glial cell line-derived neurotrophic factor in sympathetic neurons. Proc Natl Acad Sci USA 94:7018–23
- Fischer W, Wictorin K, Björklund A, et al. (1987). Amelioration of cholinergic neuron atrophy and spatial memory impairment in aged rats by nerve growth factor. Nature 329:65–8
- Gash DM, Zhang Z, Ovadia A, et al. (1996). Functional recovery in GDNF treated Parkinsonian monkeys. Nature 380:252–5
- Gong L, Wyatt RJ, Baker I, Masserano JMB. (1999). Brain-derived and glial cell line-derived neurotrophic factors protect a catecholaminergic cell line from dopamine-induced cell death. Neurosci Lett 263:153–6
- Grondin R, Cass WA, Zhang Z, et al. (2003). Glial cell line-derived neurotrophic factor increases stimulus-evoked dopamine release and motor speed in aged rhesus monkeys. J Neurosci 23:1974–80
- Hebert MA, Gerhardt GA. (1997). Behavioral and neurochemical effects of intranigral administration of glial cell line-derived neurotrophic factor on aged Fischer 344 rats. J Pharmacol Exp Ther 282:760–8
- Hossain MS, Hashimoto M, Gamoh S, Masumura S. (1999). Antioxidative effects of docosahexaenoic acid in the cerebrum versus cerebellum and brainstem of aged hypercholesterolemic rats. J Neurochem 72:1133–8
- Innis SM. (2000). The role of dietary n-6 and n-3 fatty acids in the developing brain. Dev Neurosci 22:474–80
- Jiang LH, Shi Y, Wang LS, Yang ZH. (2009a). The influence of orally administered docosahexaenoic acid on cognitive ability in aged mice. J Nutr Biochem 20:735–41
- Jiang LH, Yan S, Long Y, Yang ZR. (2009b). The influence of orally administered docosahexaenoic acid on monoamine neurotransmitter, nerve growth factor and brain-derived neurotrophic factor in aged mice. Pharm Biol 47:584–91
- Jiang LH, Liang QY, Shi Y. (2012). Pure docosahexaenoic acid can improve depression behaviors and affect HPA axis in mice. Eur Rev Med Pharmacol Sci 16:1765–73
- Jing S, Wen D, Yu Y, et al. (1996). GDNF-induced activation of the ret protein tyrosine kinase is mediated by GDNFR-alpha, a novel receptor for GDNF. Cell 85:1113–24
- Kawashima A, Harada T, Kami H, et al. (2010). Effects of eicosapentaenoic acid on synaptic plasticity, fatty acid profile and phosphoinositide 3-kinase signaling in rat hippocampus and differentiated PC12 cells. J Nutr Biochem 21:268–77
- Kiray M, Uysal N, Sönmez A, et al. (2004). Positive effects of deprenyl and estradiol on spatial memory and oxidant stress in aged female rat brains. Neurosci Lett 354:225–8
- Kirik D, Georgievska B, Björklund A. (2004). Localized striatal delivery of GDNF as a treatment for Parkinson disease. Nat Neurosci 7:105–10
- Lim SY, Suzuki H. (2002). Dose-response effect of docosahexaenoic acid ethyl ester on maze behavior and brain fatty acid composition in adult mice. Int J Vitam Nutr Res 72:77–84
- Lowry OH, Rosebrough NJ, Farr AL, Randall RJ. (1951). Protein measurement with the Folin phenol reagent. J Biol Chem 193:265–75
- McGahon BM, Martin DS, Horrobin DF, Lynch MA. (1999). Age-related changes in synaptic function: Analysis of the effect of dietary supplementation with omega-3 fatty acids. J Neurosci 94:305–14
- Mena MA, Casarejos MJ, Carazo A, et al. (1997). Glia protect fetal midbrain dopamine neurons in culture from L-DOPA toxicity through multiple mechanisms. J Neural Transm 104:317–28
- Montine TJ, Neely MD, Quinn JF, et al. (2002). Lipid peroxidation in aging brain and Alzheimer’s disease. J Free Radic Biol Med 33:620–6
- Mori TA, Woodman RJ, Burke V, et al. (2003). Effect of eicosapentaenoic acid and docosahexaenoic acid on oxidative stress and inflammatory markers in treated-hypertensive type 2 diabetic subjects. Free Radic Biol Med 35:772–81
- Nourooz-Zadeh J, Liu EH, Yhlen B, et al. (1999). F4-isoprostanes as specific marker of docosahexaenoic acid peroxidation in Alzheimer’s disease. J Neurochem 72:734–40
- Oyanagui Y. (1984). Reevaluation of assay methods and establishment of kit for superoxide dismutase activity. Anal Biochem 142:290–6
- Peng S, Zhang Y, Zhang JN, et al. (2010). ERK in learning and memory: A review of recent research. Int J Mol Sci 11:222–32
- Rangasamy SB, Soderstrom K, Bakay RA, Kordower JH. (2010). Neurotrophic factor therapy for Parkinson’s disease. Progr Brain Res 184:237–64
- Scott Bitner R. (2012). Cyclic AMP response element-binding protein (CREB) phosphorylation: A mechanistic marker in the development of memory enhancing Alzheimer’s disease therapeutics. Biochem Pharmaco 83:705–14
- Song JH, Miyazawa T. (2001). Enhanced level of n-3 fatty acid in membrane phospholipids induces lipid peroxidation in rats fed dietary docosahexaenoic acid oil. Atherosclerosis 155:9–18
- Songur A, Sarsilmaz M, Sogut S, et al. (2004). Hypothalamic superoxide dismutase, xanthine oxidase, nitric oxide, and malondialdehyde in rats fed with fish ω-3 fatty acids. Progr Neuropsychopharmacol Biol Psychiatry 28:693–8
- Tabner BJ, Turnbull S, El-Agnaf O, Allsop D. (2001). Production of reactive oxygen species from aggregating proteins implicated in Alzheimer's disease, Parkinson's disease and other neurodegenerative diseases. Curr Top Med Chem 6:507–17
- Tanriover G, Seval-Celik Y, Ozsoy O, et al. (2010). The effects of docosahexaenoic acid on glial derived neurotrophic factor and neurturin in bilateral rat model of Parkinson’s disease. Folia Histochem Cytobiol 48:434–41
- Tansey MG, Baloh RH, Milbrandt J, Johnson EM Jr. (2000). GFRa-mediated localization of RET to lipid rafts is required for effective downstream signaling, differentiation and neuronal survival. Neuron 25:611–23
- Treanor JJ, Goodman L, de Sauvage F, et al. (1996). Characterization of a multicomponent receptor for GDNF. Nature 382:80–3
- Wu A, Ying Z, Gomez-Pinilla F. (2004). Dietary omega-3 fatty acids normalize BDNF levels, reduce oxidative damage, and counteract learning disability after traumatic brain injury in rats. J Neurotrauma 21:1457–67