Abstract
Context: Seaweeds of the genera Turbinaria and Padina have long been used as food and in traditional medicine for treating several diseases.
Objective: The current study determines the protective efficacy of the brown seaweeds Turbinaria ornata (Turner) J. Agardh (Sargassaceae) and Padina pavonia (Linnaeus) J.V. Lamouroux (Dictyotaceae) against liver injury induced by azoxymethane (AOM).
Materials and methods: Male Swiss mice received 10 mg/kg AOM once a week for two consecutive weeks and then 100 mg/kg daily dose of either T. ornata or P. pavonia ethanolic extract. Thirteen weeks after the first AOM administration and 24 h after the last treatment, overnight fasted mice were sacrificed and samples collected.
Results: Compared with the AOM group, both T. ornata and P. pavonia significantly decreased the activity of aminotransferases and the concentration of bilirubin while increased albumin levels in the serum. The antioxidative effect of both extracts was observed from the increased activity of superoxide dismutase and glutathione peroxidase activities in the liver, both of which were decreased by AOM. Moreover, the levels of malondialdehyde and nitric oxide were reduced, and histological findings also confirmed the antihepatotoxic activity. In addition, treatment with T. ornata and P. pavonia significantly increased PPARγ and decreased NF-κB expression in the liver of AOM-administered mice.
Discussion and conclusion: Our findings indicate that the protective function of T. ornata and P. pavonia on AOM-induced liver injury may be possibly exerted by multiple pathways including abolishment of inflammation and oxidative damage, and activation of PPARγ.
Introduction
The liver assumes an urgent part in the regulation of different physiological courses of action in the body, other than being the most paramount organ included in the detoxification of different drugs and xenobiotics in our body (Sharma et al. Citation2011). Loss of liver capacity has impeding impacts on various organs, both because of the release of harmful components from the injured liver and the loss of key hepatic detoxifying pathways (Deng et al. Citation2012). Liver is very vulnerable to damage by xenobiotics because of its continuous exposure to these toxicants through the portal blood circulation (Piñeiro-Carrero & Piñeiro Citation2004). Azoxymethane (AOM), a metabolite of 1,2-dimethylhydrazine (DMH), is a colon cancer inducing agent, which serves as a tool to evaluate colon tumour in rats and mice (Chan et al. Citation2006). AOM has likewise been utilized as a part of the investigation of the preventive efficacy of numerous natural products on colon cancer (De Robertis et al. Citation2011). It has been reported that the liver and lungs are the most affected organs during colon cancer induction using AOM (Chan et al. Citation2006).
Peroxisome proliferator-activated receptors (PPARs) are ligand-dependent transcription factors belonging to the nuclear receptor superfamily, which additionally incorporates the steroid and thyroid hormone receptors (Soldo-Jureša & Metelko Citation2009). In the liver, PPARs exert a transcriptional activity regulating several physiological functions, including inflammatory responses, carbohydrate/lipid metabolism, regenerative mechanisms, cholesterol and bile acid homeostasis and cell differentiation/proliferation (Peyrou et al. Citation2012). Three major isoforms of PPARs including PPARα, PPARβ/δ, and PPARγ have been characterized to date (Michalik & Wahli Citation2008). Currently, dysregulations of specific PPAR isoforms contribute to the development of a wide range of hepatic diseases (Peyrou et al. Citation2012). In addition, several studies reported that excessive formation of fibrotic tissue in the liver is associated with PPARγ deficiency in hepatic stellate cells (Zhang et al. Citation2012) and activation of PPARγ signalling has been found to protect the liver against fibrosis (Nan et al. Citation2009) and drug-induced inflammation (Mahmoud Citation2014).
Over the past few decades, seaweeds and their extracts represent a source of bioactive compounds with immense therapeutic potential (Smith Citation2004) and as a supplement in functional food (Senthilkumar et al. Citation2013). Seaweeds are rich in bioactive substances like polysaccharides, minerals, polyphenols, lipids, proteins and certain vitamins, with antibacterial, antifungal, antiviral, antioxidant and antidiabetic properties (Chandini et al. Citation2008; Holdt & Kraan Citation2011; Germoush Citation2013; Mahmoud, Germoush, et al. Citation2014). We recently reported the protective effects of Turbinaria ornata (Turner) J. Agardh (Sargassaceae) and Padina pavonia (Linnaeus) J.V. Lamouroux (Dictyotaceae) against AOM-induced colon carcinogenesis through attenuation of inflammation and oxidative stress (Mahmoud et al. Citation2015). To the best of our knowledge, the significance of brown seaweeds in AOM-induced hepatic injury has not previously been assessed. Therefore, the current study investigated the protective efficacy of the brown seaweeds T. ornata and P. pavonia against AOM-induced hepatotoxicity. This investigation could promote an understanding of their mechanisms, especially to modulation of hepatic PPARγ expression and AOM-induced oxidative stress and inflammation.
Materials and methods
Chemicals
AOM (cat. no. A5486), glutathione (GSH; cat. no. G-4251), pyrogallol (cat. no. P0381), thiobarbituric acid (TBA; cat. no. T5500), 5,5′-dithiobis-(2-nitrobenzoic acid) (DTNB; cat. no. D8130), 1,1,3,3-tetramethoxypropane (cat. no. 108383), agarose (cat. no. A9539) and ethidium bromide (cat. no. E7637) were purchased from Sigma (St Louis, MO). All other chemicals were of analytical grade and obtained from standard commercial supplies.
Collection of algae and extract preparation
Both T. ornata and P. pavonia were collected from the intertidal region of the Red Sea shores between Quseir and Marsa-Alam (Egypt), during the period from March to June 2011. The seaweeds were identified at the Department of Botany, Faculty of Science, Beni-Suef University (Beni Suef, Egypt). Voucher specimens were deposited at the Department of Botany, Faculty of Science, Beni-Suef University. The samples were cleaned and washed thoroughly with sea water followed by distilled water. Collected algae were washed, air-dried and ground to a fine powder then extracted by 80% aqueous ethanol (Mahmoud, Germoush, et al. Citation2014). Following filtration, the filtrate was concentrated under reduced pressure in a rotary evaporator and lyophilization. The dry yields obtained were 1.1 g/100 g of P. pavonia and 3 g/100 g of T. ornata. Extracts were stored at −20 °C until used.
Experimental animals
Twenty-four male Swiss mice weighing between 20 and 25 g were obtained from the animal house of the National Research Centre, El-Giza, Egypt. The animals were housed in plastic well-aerated cages at normal atmospheric temperature (25 ± 5 °C) and normal 12 h light/dark cycle. Mice had free access to water and were supplied daily with laboratory standard diet. All animal procedures were approved by the Institutional Ethics Committee of Beni-Suef University (Beni Suef, Egypt).
Experimental design
Mice were allocated randomly into four groups (N = 6) and were subjected to the following treatments:
Group 1 (Control): Mice received two intraperitoneal injections of saline and were orally administered the vehicle 1% carboxymethylcellulose (CMC) (5 mL/kg body weight) throughout the experimental period.
Group 2 (AOM): Mice received intraperitoneal injection of 10 mg/kg AOM once a week for two consecutive weeks and were orally administered the vehicle 1% CMC throughout the experimental period.
Group 3 (AOM + T. ornata): Mice received AOM once a week for two consecutive weeks followed by 100 mg/kg body weight T. ornata extract suspended in 1% CMC.
Group 4 (AOM + P. pavonia): Mice received AOM once a week for two consecutive weeks followed by 100 mg/kg body weight P. pavonia extract suspended in 1% CMC.
Selection of the used doses of algal extracts was based on our recent studies (Mahmoud et al. Citation2013; Mahmoud, Germoush, et al. Citation2014; Mahmoud et al. Citation2015). The doses were balanced consistently as indicated by any change in body weight to keep up comparable dosage for every kg body weight over the entire period of study. Thirteen weeks after the first AOM administration and 24 h after the last treatment, overnight fasted mice were sacrificed under ether anaesthesia and samples collected.
Sample preparation
Collected blood samples were left to coagulate then centrifuged at 3000 rpm for 15 min to separate serum. Liver samples were excised and immediately perfused with ice-cold saline. Frozen liver samples (10% w/v) were homogenized in chilled saline and the homogenates were centrifuged at 3000 rpm for 15 min at 4 °C using Centurion Scientific K3 cooling centrifuge (West Sussex, UK) to separate the nuclear debris. Aliquots of the clear homogenate were prepared and used for determination of different biochemical markers. Samples from the liver were also taken and stored in liquid nitrogen for RNA isolation and others were fixed in neutral buffered formalin for histopathological investigation.
Assay of serum markers of liver damage
Serum aspartate aminotransferase (AST) and alanine aminotransferase (ALT) activities were assayed according to the method of Schumann and Klauke (Citation2003) using reagent kit purchased from Biosystems (Barcelona, Spain). Serum albumin and total bilirubin were determined following the methods of Webster (Citation1974) and Kaplan and Pesce (Citation1984) using kits supplied by Diamond Diagnostics (Cairo, Egypt).
Assay of oxidative stress and antioxidant status
Assay of lipid peroxidation
Malondialdehyde (MDA), an index for determining the extent of lipid peroxidation, was measured by the method of Preuss et al. (Citation1998) after slight modifications. Briefly, 250 μL of liver homogenate was precipitated with 75 μL of 76% trichloroacetic acid (TCA) and centrifuged at 3000 rpm for 10 min. The supernatant was collected and mixed with 175 μL of 1.07% TBA. The mixture was incubated for 30 min at 80 °C with shaking. Following incubation, 250 μL cold 90% TCA was added, and the absorbance of the pink coloured chromogen formed was determined at 532 nm. 1,1,3,3-Tetramethoxypropane served as a standard.
Assay of nitric oxide
Liver nitric oxide (NO) level was assayed as nitrite using a reagent kit purchased from Biodiagnostics (Cairo, Egypt), according to the method of Montgomery and Dymock (Citation1961). The assay is based on the Griess reaction which is a simple colorimetric reaction between sulphonamide, N-(1-naphthyl)ethylenediamine and nitrite to produce a pink azo-product with maximum absorbance at 543 nm.
Assay of GSH
GSH content was determined according to the procedure of Beutler et al. (Citation1963) with some modifications. Briefly, 200 μL liver homogenate was precipitated with 1 mL of precipitating solution containing 0.2% EDTA, 1.67% glacial metaphosphoric acid and 30% NaCl. The mixture was incubated for 5 min at room temperature followed by centrifugation at 3000 rpm for 5 min. One millilitre of the supernatant was mixed with 0.5 mL of 0.04% DTNB in 1% sodium citrate and 4 mL of 0.3 M Na2HPO4, and absorbance was measured at 412 nm.
Assay of superoxide dismutase activity
Liver superoxide dismutase (SOD) activity was determined according to the method of Marklund and Marklund (Citation1974). The reaction mixture consisted of 200 μL tissue homogenate, 500 μL Tris/EDTA buffer (pH 8.0), 100 μL of 10 mM pyrogallol and 200 μL distilled water. The absorbance at zero time and after 10 min was measured at 420 nm. One enzyme unit is defined as the enzyme activity that inhibits auto-oxidation of pyrogallol by 50%.
Assay of glutathione peroxidase activity
Glutathione peroxidase (GPx) activity was assayed according to the method of Matkovics et al. (Citation1998). Briefly, 50 μL of the liver homogenate, 350 μL Tris buffer (pH7.6), 50 μL of 3.28 mM hydrogen peroxide (H2O2) and 50 μL of 2 mM GSH were mixed and incubated for 10 min at room temperature. One hundred microliters of precipitating solution containing 1.67% glacial metaphosphoric acid, 0.2% EDTA and 30% NaCl was added, and samples were centrifuged at 3000 rpm for 5 min. The supernatant was separated and mixed with 0.5 mL of DTNB, and the absorbance was measured at 412 nm.
RNA isolation and reverse transcriptase polymerase chain reaction (RT-PCR)
Gene expression analysis was performed as we previously reported (Mahmoud Citation2013). Total RNA was isolated from liver samples using total RNA isolation kit (Fermentas, Waltham, MA). RNA was purified and spectrophotometrically quantified. First strand of cDNA was synthesized from 2 μg RNA by using reverse transcription kit (Fermentas, Waltham, MA). The produced cDNA was amplified by Green master mix (Fermentas, Waltham, MA) using the primer set listed in . The reaction tubes were placed on a double heated led thermal cycler and the reaction series included initial denaturation at 95 °C for 2 min followed by 35 cycles of denaturation at 95 °C for 30 s, annealing at Tm-5 for 30 s and extension at 72 °C for 30 s. PCR products were mixed with blue loading dye and then were loaded in 1.5% agarose gel with ethidium bromide and electrophoresed for suitable time. The resulted gels were visualized using UV transilluminator, scanned and analysed by ImageJ (NIH, Bethesda, MD). The values were normalized to β-actin and represented as percentage relative to control.
Table 1. Primer pairs used for PCR.
Histopathology
The liver samples were flushed with saline and then fixed in 10% buffered formalin for at least 24 h. After fixation, the specimens were dehydrated in ascending series of ethanol, cleared in xylene, and embedded in paraffin wax. Blocks were made and 4 μm thick sections were cut by a sledge microtome. The paraffin embedded liver tissue sections were deparaffinized using xylene and ethanol. The slides were washed with phosphate buffered saline (PBS) and stained with haematoxylin and eosin (H&E). The stained slides were examined under light microscope.
Statistical analysis
Statistical analysis was performed using GraphPad Prism 5 (GraphPad Software, San Diego, CA). Results were expressed as mean ± standard error (SEM) and all statistical comparisons were made by means of the one-way ANOVA test followed by Tukey’s test post hoc analysis. A p value <0.05 was considered significant.
Results
Effect of T. ornata and P. pavonia on liver function markers
Treatment of mice with AOM induced marked impairment of liver function evidenced by a significant (p < 0.001) elevation of serum AST and ALT compared to control mice (). Oral supplementation of T. ornata as well as P. pavonia significantly (p < 0.001) ameliorated the altered serum aminotransferases. Similarly, total bilirubin concentration revealed a significant (p < 0.001) increase in serum of AOM-administered mice when compared to the control group. Treatment of AOM-induced mice with T. ornata and P. pavonia markedly decreased the elevated serum bilirubin levels; T. ornata seemed to be more potent (). However, serum albumin of the AOM group of mice was significantly (p < 0.01) declined and treatment with either tested algal extract noticeably (p < 0.001) ameliorated the diminished albumin levels, as represented in . Compared to the control, treatment with both T. ornata and P. pavonia produced a significant (p < 0.001) increase in serum albumin concentration.
Table 2. Liver function markers in control and experimental mice.
Effect of T. ornata and P. pavonia on liver oxidative stress and antioxidant markers
The AOM-induced mice exhibited a significant (p < 0.001) increase in the lipid peroxidation marker MDA when compared with the control group (). Both treatment agents produced a complete normalization (p < 0.001) of the liver MDA content. Treatment of AOM-administered mice with P. pavonia extract significantly (p < 0.01) decreased liver MDA level when compared to the control mice. Likewise, NO was significantly (p < 0.001) elevated in the liver of AOM group when compared with the control mice and decreased significantly following treatment with either T. ornata (p < 0.001) or P. pavonia (p < 0.05) extract ().
Figure 1. Liver lipid peroxidation (A) and nitric oxide (B) in control, AOM and AOM mice treated with T. ornata and P. pavonia. Data are expressed as mean ± SEM. **p < 0.01 and ***p < 0.001 versus control and #p < 0.05, ##p < 0.01 and ###p < 0.001 versus AOM. MDA, malondialdehyde; NO, nitric oxide; AOM, azoxymethane; T. ornata, Turbinaria ornata; P. pavonia, Padina pavonia; SEM, standard error of the mean.
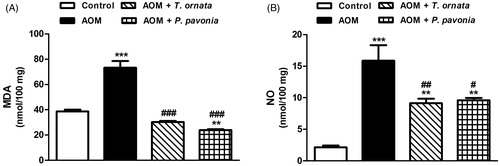
Conversely, liver GSH of the AOM control mice showed a significant (p < 0.001) decrease on comparison with the corresponding normal control mice (). Following the same manner, AOM-induced mice exhibited significantly declined activities of liver SOD (p < 0.01) and GPx (p < 0.001) as represented in , respectively. Treatment of AOM-administered mice with T. ornata and P. pavonia significantly (p < 0.001) alleviated hepatic GSH content and GPx activity. Supplementation of T. ornata produced a significant (p < 0.001) increase in liver SOD activity. Although P. pavonia extract increased liver SOD activity, the recorded effect was non-significant (p > 0.05) when compared with the AOM-intoxicated mice.
Figure 2. Liver glutathione content (A), and activity of SOD (B) and GPx (C) in control, AOM and AOM mice treated with T. ornata and P. pavonia. Data are expressed as mean ± SEM. *p < 0.05, **p < 0.01 and ***p < 0.001 versus control, ###p < 0.001 versus AOM and ††p < 0.01 versus AOM + T. ornata. GSH, glutathione; SOD, superoxide dismutase; GPx, glutathione peroxidase; AOM, azoxymethane; T. ornata, Turbinaria ornata; P. pavonia, Padina pavonia; SEM, standard error of the mean.
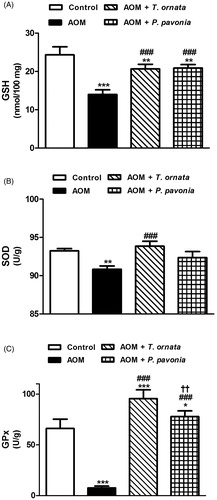
Histopathological examination
Histopathological examination of the liver sections of control mice showed normal histological structure of the hepatic lobule (). However, sections in the liver of mice treated with AOM showed fatty degeneration of the hepatocytes, blood vessel congestion, necrosis, pyknotic nuclei, inflammatory cell infiltration, karyolysis, fragmented chromatin, karyomegaly and oval cell proliferation (). Treatment of AOM-induced mice with either T. ornata () or P. pavonia () produced marked improvement in the liver architecture with more or less normal hepatocytes; however, the microscopic examination revealed the presence of mild fatty degeneration.
Figure 3. Photomicrographs of H&E stained liver sections of control, AOM and AOM mice treated with T. ornata and P. pavonia. (A and B) Liver sections of control mice showing normal histological structure of a hepatic lobule with central vein (cv) and blood sinusoid (s) (A ×400 and B ×1000), (C–F) liver sections of AOM group revealing fatty degeneration of hepatocytes (C), dilated sinusoids, congested blood vessels (D), inflammatory cell infiltration, necrosis (E), and oval cell proliferation (F) [×x400], (G and H) liver sections of AOM + T. ornata demonstrating marked improvement of liver architecture with mild fatty degeneration in some hepatocytes [×400], and (I and J) sections of AOM + P. pavonia showing more or less normal liver section, mild fatty changes and normal well differentiated nuclei [×400].
![Figure 3. Photomicrographs of H&E stained liver sections of control, AOM and AOM mice treated with T. ornata and P. pavonia. (A and B) Liver sections of control mice showing normal histological structure of a hepatic lobule with central vein (cv) and blood sinusoid (s) (A ×400 and B ×1000), (C–F) liver sections of AOM group revealing fatty degeneration of hepatocytes (C), dilated sinusoids, congested blood vessels (D), inflammatory cell infiltration, necrosis (E), and oval cell proliferation (F) [×x400], (G and H) liver sections of AOM + T. ornata demonstrating marked improvement of liver architecture with mild fatty degeneration in some hepatocytes [×400], and (I and J) sections of AOM + P. pavonia showing more or less normal liver section, mild fatty changes and normal well differentiated nuclei [×400].](/cms/asset/f52df764-ae96-4904-927c-720b3b2b6ff9/iphb_a_1160938_f0003_c.jpg)
Effect of T. ornata and P. pavonia on liver NF-κB and PPARγ gene expression
AOM administration to the mice produced a significant (p < 0.05) up-regulation of the liver NF-κB mRNA expression (). Oral supplementation of both algal extracts produced significant (p < 0.001) down-regulation of liver NF-κB expression. By comparison to control mice, liver NF-κB mRNA expression was significantly declined following treatment of AOM-induced mice with T. ornata (p < 0.001) and P. pavonia (p < 0.01). On the other hand, liver PPARγ mRNA expression showed significant (p < 0.001) down-regulation in AOM-induced mice and was markedly increased in T. ornata (p < 0.05) and P. pavonia (p < 0.01) treated mice, as depicted in .
Figure 4. PCR analysis of liver NF-κB in control, AOM and AOM mice treated with T. ornata and P. pavonia. (A) Gel photograph depicting representative NF-κB and β-actin PCR products. (B) Corresponding densitometric analysis of the PCR products. Data are expressed as mean ± SEM. *p < 0.05, **p < 0.01 and ***p < 0.001 versus control and ###p < 0.001 versus AOM. NF-κB, nuclear factor-kappa B; AOM, azoxymethane; T. ornata, Turbinaria ornata; P. pavonia, Padina pavonia; SEM, standard error of the mean.
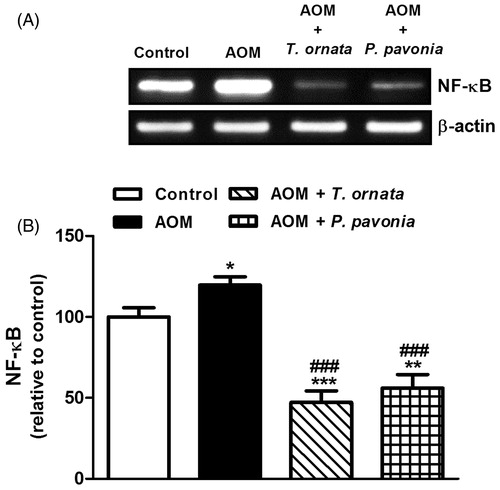
Figure 5. PCR analysis of liver PPARγ in control, AOM and AOM mice treated with T. ornata and P. pavonia. (A) Gel photograph depicting representative PPARγ and β-actin PCR products. (B) Corresponding densitometric analysis of the PCR products. Data are expressed as mean ± SEM. **p < 0.01 versus control and #p < 0.05, ##p < 0.01 versus AOM. PPARγ, peroxisome proliferator activated receptor gamma; AOM, azoxymethane; T. ornata, Turbinaria ornata; P. pavonia, Padina pavonia; SEM, standard error of the mean.
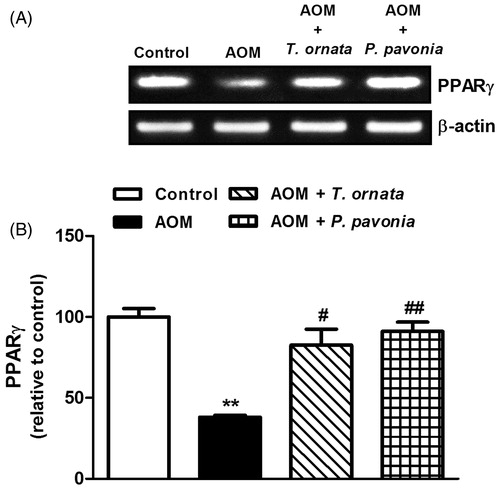
Discussion
AOM was first identified as an ingredient in cycad palms and subsequently used as a selective intestinal carcinogen (Laqueur et al. Citation1963; Waly et al. Citation2014). Several studies revealed that AOM is a potent carcinogen for the liver (Chastre et al. Citation2012) and at low doses induce hepatotoxicity with ductular hyperplasia (Shan et al. Citation2008; Khurana et al. Citation2010). Although derived from a rare plant and human exposure to AOM is infrequent, exposure to structurally related hydrazine compounds that are found in herbicides, rocket fuels, tobacco and drugs is common (Gamberini et al. Citation1998). Therefore, by use of AOM-induced hepatic injury in mice we conducted the present study to elucidate the role of brown seaweeds in mitigating AOM-induced hepatotoxicity. We hypothesized that the protective effect of the tested extracts might be mediated through up-regulation of PPARγ expression, and abrogation of inflammation and oxidative stress.
Mice treated with AOM developed liver injury characterized histologically by extensive fatty degeneration in periportal and mid-zonal regions, necrosis, dilation and congestion of blood vessels and periportal sinusoids, vascular degeneration, inflammatory cells infiltration and other histological manifestations. These findings are consistent with previous studies demonstrated that AOM induces chronic injury in murine liver (Shan et al. Citation2008; Khurana et al. Citation2010). Further, our findings confirm previous observations (Khurana et al. Citation2010) by showing that AOM administration induces oval cell expansion. The recorded oval cell proliferation in the present study has been reported in human chronic liver diseases and animal models of liver injury (Roskams et al. Citation2004; Clouston et al. Citation2005). In addition, oval cells are likewise thought to be precursors of hepatic cancer cells (Lee et al. Citation2009) and oval cell expansion has been observed before the development of candid neoplasia in animal models of hepatocarcinoma (Libbrecht et al. Citation2000).
Moreover, AOM-induced hepatic damage is evidenced by elevation of the circulating levels of aminotransferases and total bilirubin. In this context, Ramaiah (Citation2007) reported that aminotransferases are the most sensitive markers of hepatic damage and liver cell injury lead to their leakage into the blood circulation. Hence, we assume that the observed high level of these enzymes is the consequence of AOM-induced hepatic damage and loss of functional integrity of liver cell membrane. These findings are similar to those observed in the studies of Bélanger et al. (Citation2006) and Bémeur et al. (Citation2010) who reported elevated circulating aminotransferases in AOM treated mice. Oral supplementation of either T. ornata or P. pavonia extract attenuated oval cell proliferation and showed a marked improvement in the liver architecture, proving their hepatoprotective activities. Reversal of the elevated serum aminotransferases by both tested extracts refers to their membrane stabilizing efficacies. Accordingly, our recent study reported that T. ornata protected against cyclophosphamide-induced hepatotoxicity in rats (Mahmoud et al. Citation2013). Moreover, Padina tetrastromatica (Dictyotaceae) showed a hepatoprotective activity against carbon tetrachloride induced hepatotoxicity in rats (Selvi et al. Citation2014).
Several carcinogens have been reported to evoke hepatic oxidative stress and modify the activities of antioxidant enzymes (Aruna & Sivaramakrishnan Citation1996). The metabolism of AOM produces extremely reactive hydroxyl radical that induce oxidative stress (Khanum et al. Citation1998; Waly et al. Citation2014). The resultant reactive oxygen species (ROS) induce lipid peroxidation, protein damage and DNA fragmentation (Nencini et al. Citation2007). ROS-induced damages result in gene mutations and loss of membrane integrity (Reddy et al. Citation2009). In the present study, AOM induced a significant increase in hepatic lipid peroxidation levels. These findings are in agreement with the studies of Anilakumar et al. (Citation2010) and Gürocak et al. (Citation2013) who demonstrated elevated lipid peroxidation in the liver of AOM treated rats. Similarly, AOM-administered mice exhibited significantly elevated hepatic NO levels. In this context, Li and Billiar (Citation1999) reported that toxin-induced hepatic injury is associated with increased hepatic NO production. The produced NO reacts with superoxide anions to form the potent oxidant peroxinitrite (McKim et al. Citation2003). In addition, NO stimulates production of the pro-inflammatory cytokine, tumour necrosis factor alpha (TNF-α), through activation of the oxidant-sensitive transcription factor NF-κB in Kupffer cells (Matata & Galinanes Citation2002). On the contrary, supplementation of T. ornata as well as P. pavonia markedly decreased hepatic content of MDA and NO, suggesting their antioxidant potential. Likewise, T. ornata has been reported to protect against cyclophosphamide-induced lipid peroxidation in rats (Mahmoud et al. Citation2013). In addition, Germoush (Citation2013) demonstrated that T. ornata and P. pavonia markedly ameliorated hepatic lipid peroxidation levels in diabetic rats. Moreover, we recently reported the ameliorative and antioxidant effects of both tested seaweeds against AOM-induced colon carcinogenesis (Mahmoud et al. Citation2015) and nephrotoxicity (Mahmoud, El-Derby, et al. Citation2014).
Liver GSH content by comparison was significantly declined in AOM-treated mice. The recorded depletion of hepatic GSH might be attributed to its conjugation with AOM metabolites, thus reducing cellular GSH levels leading to oxidative stress. Consequently, AOM-administered mice exhibited reduced activities of the antioxidant enzymes, SOD and GPx. These enzymes play a critical role in maintaining the body’s defence mechanism against the destructive effects of ROS and free radicals (Chandra et al. Citation2000; Wei et al. Citation2011). T. ornata as well as P. pavonia considerably prevented GSH depletion and improved activities of hepatic SOD and GPx. More recently, we revealed that T. ornata and P. pavonia extracts potentially decreased lipid peroxidation and nitric oxide levels, and enhanced the enzymatic and non-enzymatic antioxidant defences in kidney (Mahmoud, El-Derby, et al. Citation2014) and colon (Mahmoud et al. Citation2015) of AOM-intoxicated mice.
An interesting finding of the current study is the significant increase in serum albumin levels following treatment with T. ornata and P. pavonia. This above normal increase in serum albumin could be attributed to the potent antioxidant and anti-inflammatory effects of the tested brown seaweeds. In addition, brown seaweeds are rich in proteins and a source of essential and non-essential amino acids (Černá Citation2011) and therefore, could increase albumin synthesis. Several studies conducted both in experimental animals and humans have shown that the protein content of ingested nutrients has an important role in the regulation of albumin synthesis (Caso et al. Citation2000). Indeed, amino acids have been shown to up-regulate the synthesis of albumin in hepatocytes in vitro (Flaim et al. Citation1982). The decreased serum albumin levels in AOM-administered mice could be attributed to the induced oxidative stress and inflammation. During inflammation, the cytokines serve to shunt amino acids towards positive acute-phase proteins and away from producing proteins that are unnecessary to the inflammatory process (Rothschild et al. Citation1972). Accordingly, albumin production has been reported to decrease because of its function as a negative acute phase protein in liver disease patients with an inflammatory component (Doweiko & Nompleggi Citation1991). In addition, albumin concentration dropped significantly during inflammation in human and experimental animals (Peters Citation1996). Also, the study of Sezer et al. (Citation2007) demonstrated a negative interaction between oxidative stress and serum albumin level in chronic renal failure patients. Therefore, anti-inflammatory and antioxidant molecules could possibly restore serum albumin levels to normal levels. Here, we suggest that the ameliorated serum albumin levels in AOM-induced mice following treatment with either T. ornata or P. pavonia is related to the suppression of oxidative stress and inflammation. The potent anti-inflammatory and antioxidant effects of both T. ornata and P. pavonia could be due to their ability to produce a significant down-regulation of NF-κB, and the rich content of antioxidants. The recovered serum albumin might have a role in mitigating AOM-induced oxidative stress and inflammation. Through the reduced cysteine, sulphhydryl group and methionine residues, albumin is able to scavenge hydroxyl radicals (Gutteridge Citation1986), peroxynitrite (Carballal et al. Citation2003) and hypochlorous acid (Halliwell Citation1988). However, further investigations are needed to clarify the exact mechanism through which T. ornata and P. pavonia increased serum albumin levels.
Multiple in vivo and in vitro studies reported that elevated levels of NF-κB induce apoptosis in hepatocytes and play a central role in the pathogenesis of cell injury in experimental models of induced liver damage (Garcia-Ruiz et al. Citation2003; Ding & Yin Citation2004; Mahmoud Citation2014). NF-κB is a nuclear transcription factor involved in controlling the expression of various genes including, TNF-α, interleukin-1β and cyclooxygenase-2 (Muriel Citation2007). These inflammatory mediators promote migration and infiltration of leukocytes into the injured organ and provoke the primary injury induced by the toxicant (Akcay et al. Citation2009). Elevated NF-κB expression and NO levels in the liver of AOM-administered mice in the present study may reflect the degree of inflammation. The observed hepatoprotective effect of both T. ornata and P. pavonia extracts may be explained, at least in part, by their ability to produce a highly significant down-regulation of NF-κB expression in the liver.
The anti-inflammatory, antioxidant and hepatoprotective effects of T. ornata and P. pavonia can be directly connected to their ability to up-regulate PPARγ expression in the liver. PPARγ is able to inhibit NF-κB activation and its subsequent release of NO and pro-inflammatory cytokines through binding to NF-κB subunits (Straus et al. Citation2000), competing for common transcription coactivators (Chung et al. Citation2000), or up-regulating the inhibitor kappa B (Delerive et al. Citation2000). In this context, a recent study revealed that PPARγ activation, in a dose and time-dependent manner, remarkably inhibits inflammatory cytokines-induced NF-κB transcriptional activity (Remels et al. Citation2009). Mahmoud (Citation2014) revealed that PPARγ activation suppresses NF-κB-induced release of inflammatory mediators in an experimental model of liver injury. Also, PPARγ pathway has been reported to suppress the fibrogenic activity of hepatic stellate cells (Galli et al. Citation2002). In addition, PPARγ has been demonstrated to up-regulate the expression of antioxidant enzymes through binding to their elements in the promoters of their genes (Okuno et al. Citation2008; Gong et al. Citation2012). Therefore, through their dual ability to attenuate oxidative stress and up-regulate PPARγ, brown seaweeds potentially down-regulate NF-κB expression.
The observed beneficial effects of both tested seaweeds against AOM-induced liver injury could be connected to their rich content of sulphated polysaccharides, phenolics and carotenoids. The carotenoid fucoxanthin has been reported to inhibit NF-κB in HepG2 cells (Liu et al. Citation2013). Sulphated polysaccharides such as fucoidan are known to possess immunomodulating as well as anti-inflammatory effects (Leiro et al. Citation2007). Polyphenols are able to lower NF-κB-inducing kinase (Jaganathan & Mandal Citation2009), down-regulate hepatic NF-κB, activate PPARγ and potentiate the hepatic antioxidant defence system (Mahmoud Citation2014).
Conclusion
The current study confers new information on the protective mechanisms of T. ornata and P. pavonia against AOM-induced liver damage. Our findings suggest that both T. ornata and P. pavonia have the ability to suppress inflammation and oxidative damage induced by the chemical carcinogen AOM. These effects might be attributed to the antioxidant nature and radical scavenging activity of the active constituents including fucoxanthin, fucoidan, and polyphenolics. Further, this is the first evidence that the hepatoprotective effects of brown seaweeds are mediated via up-regulation of liver PPARγ expression, pending more investigations to trace out their exact mechanistic pathways.
Funding information
This research received no specific grant from any funding agency in the public, commercial, or not-for-profit sectors.
Disclosure statement
The authors have declared that no competing interests exist.
References
- Akcay A, Nguyen Q, Edelstein CL. 2009. Mediators of inflammation in acute kidney injury. Mediators Inflamm. 2009:137072.
- Anilakumar KR, Sudarshanakrishna KR, Chandramohan G, Ilaiyaraja N, Khanum F, Bawa AS. 2010. Effect of Aloe vera gel extract on antioxidant enzymes and azoxymethane-induced oxidative stress in rats. Indian J Exp Biol. 48:837–842.
- Aruna K, Sivaramakrishnan VM. 1996. Anticarcinogenic effects of the essential oils from cumin, poppy and basil. Phytother Res. 10:577–880.
- Bélanger M, Coté J, Butterworth RF. 2006. Neurobiological characterization of an azoxymethane mouse model of acute liver failure. Neurochem Int. 48:434–440.
- Bémeur C, Desjardins P, Butterworth RF. 2010. Antioxidant and anti-inflammatory effects of mild hypothermia in the attenuation of liver injury due to azoxymethane toxicity in the mouse. Metab Brain Dis. 25:23–29.
- Beutler E, Duron O, Kelly BM. 1963. Improved method for the determination of blood glutathione. J Lab Clin Med. 61:882–888.
- Carballal S, Radi R, Kirk MC, Barnes S, Freeman BA, Alvarez B. 2003. Sulfenic acid formation in human serum albumin by hydrogen peroxide and peroxynitrite. Biochemistry. 42:9906–9914.
- Caso G, Scalfi L, Marra M, Covino A, Muscaritoli M, McNurlan MA, Garlick PJ, Contaldo F. 2000. Albumin synthesis is diminished in men consuming a predominantly vegetarian diet. J Nutr. 130:528–533.
- Černá M. 2011. Seaweed proteins and amino acids as nutraceuticals. Adv Food Nutr Res. 64:297–312.
- Chan CH, Cook D, Stanners CP. 2006. Increased colon tumor susceptibility in azoxymethane treated CEABAC transgenic mice. Carcinogenesis. 27:1909–1916.
- Chandini SK, Ganesan P, Suresh P, Bhaskar N. 2008. Seaweeds as a source of nutritionally beneficial compounds – a review. J Food Sci Technol. 45:1–13.
- Chandra J, Samali A, Orrenius S. 2000. Triggering and modulation of apoptosis by oxidative stress. Free Radic Biol Med. 29:323–333.
- Chastre A, Bélanger M, Beauchesne E, Nguyen BN, Desjardins P, Butterworth RF. 2012. Inflammatory cascades driven by tumor necrosis factor-alpha play a major role in the progression of acute liver failure and its neurological complications. PLoS One. 7:e49670.
- Chung SW, Kang BY, Kim SH, Pak YK, Cho D, Trinchieri G, Kim TS. 2000. Oxidized low density lipoprotein inhibits interleukin-12 production in lipopolysaccharide-activated mouse macrophages via direct interactions between peroxisome proliferator-activated receptor-gamma and nuclear factor-kappa B. J Biol Chem. 275:32681–32687.
- Clouston AD, Powell EE, Walsh MJ, Richardson MM, Demetris AJ, Jonsson JR. 2005. Fibrosis correlates with a ductular reaction in hepatitis C: Roles of impaired replication, progenitor cells and steatosis. Hepatology 41:809–18.
- De Robertis M, Massi E, Poeta ML, Carotti S, Morini S, Cecchetelli L, Signori E, Fazio VM. 2011. The AOM/DSS murine model for the study of colon carcinogenesis: from pathways to diagnosis and therapy studies. J Carcinog. 10:9. doi: 10.4103/1477-3163.78279.
- Delerive P, Gervois P, Fruchart JC, Staels B. 2000. Induction of IkappaBalpha expression as a mechanism contributing to the anti-inflammatory activities of peroxisome proliferator-activated receptor-alpha activators. J Biol Chem. 275:36703–36707.
- Deng L, Gui X, Xiong Y, Gao S, Yang R, Rong Y, Hu J, Yan Y. 2012. End-stage liver disease: prevalence, risk factors and clinical characteristics in a cohort of HIV-HCV coinfected Han Chinese. Clin Res Hepatol Gastroenterol. 36:572–582.
- Ding WX, Yin XM. 2004. Dissection of the multiple mechanisms of TNF-alpha-induced apoptosis in liver injury. J Cell Mol Med. 8:445–454.
- Doweiko JP, Nompleggi DJ. 1991. The role of albumin in human physiology and pathophysiology, Part III: Albumin and disease states. J Parenter Enteral Nutr. 15:476–483.
- Flaim KE, Peavy DE, Everson WV, Jefferson LS. 1982. The role of amino acids in the regulation of protein synthesis in perfused rat liver. I. Reduction in rates of synthesis resulting from amino acid deprivation and recovery during flow-through perfusion. J Biol Chem. 257:2932–2938.
- Galli A, Crabb DW, Ceni E, Salzano R, Mello T, Svegliati-Baroni G, Ridolfi F, Trozzi L, Surrenti C, Casini A. 2002. Antidiabetic thiazolidinediones inhibit collagen synthesis and hepatic stellate cell activation in vivo and in vitro. Gastroenterology. 122:1924–1940.
- Gamberini M, Cidade MR, Valotta LA, Armelin MC, Leite LC. 1998. Contribution of hydrazines-derived alkyl radicals to cytotoxicity and transformation induced in normal c-myc-overexpressing mouse fibroblasts. Carcinogenesis. 19:147–155.
- Garcia-Ruiz C, Colell A, Marí M, Morales A, Calvo M, Enrich C, Fernández-Checa JC. 2003. Defective TNF-alpha-mediated hepatocellular apoptosis and liver damage in acidic sphingomyelinase knockout mice. J Clin Invest. 111:197–208.
- Germoush MO. 2013. Antioxidant and anti-inflammatory effects of Padina pavonia and Turbenaria ornata in streptozotocin/nicotinamide diabetic rats. Life Sci J. 10:1265–1271.
- Gong P, Xu H, Zhang J, Wang Z. 2012. PPAR expression and its association with SOD and NF-κB in rats with obstructive jaundice. Biomed Res – India. 23:551–560.
- Gürocak S, Karabulut E, Karadağ N. 2013. Preventive effects of resveratrol against azoxymethane induced damage in rat liver. Asian Pac J Cancer Prev. 14:2367–2370.
- Gutteridge JM. 1986. Antioxidant properties of the proteins caeruloplasmin, albumin and transferrin. A study of their activity in serum and synovial fluid from patients with rheumatoid arthritis. Biochim Biophys Acta. 869:119–127.
- Halliwell B. 1988. Albumin – an important extracellular antioxidant? Biochem Pharmacol. 37:569–571.
- Holdt SL, Kraan S. 2011. Bioactive compounds in seaweed; functional food applications and legislation. J Appl Phycol. 23:543–597.
- Jaganathan SK, Mandal M. 2009. Antiproliferative effects of honey and of its polyphenols: a review. J Biomed Biotechnol. 2009:1–13.
- Kaplan LA, Pesce AJ. 1984. Clinical chemistry: theory, analysis, and correlation. St. Louis (MO): Mosby.
- Khanum F, Anilakumar KR, Sudarshana Krishna KR, Viswanathan KR. 1998. Effects of feeding fresh garlic and garlic oil on detoxifying enzymes and micronuclei formation in rats treated with azoxymethane. Int J Vitam Nutr Res. 68:208–213.
- Khurana S, Shah N, Cheng K, Shiu B, Samimi R, Belo A, Shant J, Drachenberg C, Wess J, Raufman JP. 2010. Scopolamine treatment and muscarinic receptor subtype-3 gene ablation augment azoxymethane-induced murine liver injury. J Pharmacol Exp Ther. 333:639–649.
- Laqueur GL, Mickelsen O, Whiting MG, Kurland LT. 1963. Carcinogenic properties of nuts from Cycas circinalis L. indigenous to Guam. J Natl Cancer Inst. 31:919–951.
- Lee TK, Castilho A, Ma S, Ng IO. 2009. Liver cancer stem cells: implications for a new therapeutic target. Liver Int. 29:955–965.
- Leiro JM, Castro R, Arranz JA, Lamas J. 2007. Immunomodulating activities of acidic sulphated polysaccharides obtained from the seaweed Ulva rigida C. Agardh. Int Immunopharmacol. 7:879–888.
- Li J, Billiar TR. 1999. Nitric oxide. IV. Determinants of nitric oxide protection and toxicity in liver. Am J Physiol. 276:G1069–G1073.
- Libbrecht L, Desmet V, Van Damme B, Roskams T. 2000. The immunohistochemical phenotype of dysplastic foci in human liver: correlation with putative progenitor cells. J Hepatol. 33:76–84.
- Liu CL, Lim YP, Hu ML. 2013. Fucoxanthin enhances cisplatin-induced cytotoxicity via NFκB-mediated pathway and downregulates DNA repair gene expression in human hepatoma HepG2 cells. Mar Drugs. 11:50–66.
- Mahmoud AM. 2013. Hematological alterations in diabetic rats; role of adipocytokines and effect of citrus flavonoids. EXCLI J. 12:647–657.
- Mahmoud AM. 2014. Hesperidin protects against cyclophosphamide-induced hepatotoxicity by upregulation of PPARγ and abrogation of oxidative stress and inflammation. Can J Physiol Pharmacol. 92:717–724.
- Mahmoud AM, Abdella EM, El-Derby AM, Abdella EM. 2015. Protective effects of Turbinaria ornata and Padina pavonia against azoxymethane-induced colon carcinogenesis through modulation of PPAR gamma, NF-κB and oxidative stress. Phytother Res. 29:737–748.
- Mahmoud AM, El-Derby AM, Elsayed KNM, Abdella EM. 2014. Brown seaweeds ameliorate renal alterations in mice treated with the carcinogen azoxymethane. Int J Pharm Pharmaceut Sci. 6:365–369.
- Mahmoud AM, Germoush MO, Elgebaly HA, Elsayed KNM, Hassan S, Mousa NM, Hussein OE. 2014. Antidiabetic and insulin sensitizing effects of brown seaweeds in streptozotocin/nicotinamide diabetic rats. Asian J Pharm Clin Res. 7:74–78.
- Mahmoud AM, Hussein OE, Ramadan SA. 2013. Amelioration of cyclophosphamide-induced hepatotoxicity by the brown seaweed Turbinaria ornata. Int J Clin Toxicol. 1:9–17.
- Marklund SL, Marklund G. 1974. Involvement of the superoxide anion radical in the autoxidation of pyrogallol and a convenient assay for superoxide dismutase. Eur J Biochem. 47:469–474.
- Matata BM, Galinanes M. 2002. Peroxynitrite is an essential component of cytokines production mechanism in human monocytes through modulation of nuclear factor-kappa B DNA binding activity. J Biol Chem. 277:2330–2335.
- Matkovics B, Szabo L, Varga IS. 1998. Determination of enzyme activities in lipid peroxidation and glutathione pathways (in Hungarian). Laboratoriumi Diagnosztika. 15:248–249.
- McKim SE, Gäbele E, Isayama F, Lambert JC, Tucker LM, Wheeler MD, Connor HD, Mason RP, Doll MA, Hein DW, et al. 2003. Inducible nitric oxide synthase is required in alcohol-induced liver injury: studies with knockout mice. Gastroenterology. 125:1834–1844.
- Michalik L, Wahli W. 2008. PPARs mediate lipid signaling in inflammation and cancer. PPAR Res. 2008;134059.
- Montgomery HAC, Dymock JF. 1961. The determination of nitrite in water. Analyst. 86:414–416.
- Muriel P. 2007. Cytokines in liver disease. In: Sahu S, ed. Hepatotoxicity: from genomics to in vitro and in vivo models. Chichester: Wiley. p. 371–389.
- Nan YM, Fu N, Wu WJ, Liang BL, Wang RQ, Zhao SX, Zhao JM, Yu J. 2009. Rosiglitazone prevents nutritional fibrosis and steatohepatitis in mice. Scand J Gastroenterol. 44:358–365.
- Nencini C, Giorgi G, Micheli L. 2007. Protective effect of silymarin on oxidative stress in rat brain. Phytomedicine. 14:129–135.
- Okuno Y, Matsuda M, Kobayashi H, Morita K, Suzuki E, Fukuhara A, Komuro R, Shimabukuro M, Shimomura I. 2008. Adipose expression of catalase is regulated via a novel remote PPARgamma-responsive region. Biochem Biophys Res Commun. 366:698–704.
- Peters T. Jr 1996. All about albumin: biochemistry, genetics, and medical applications. San Diego (CA): Academic Press.
- Peyrou M, Ramadori P, Bourgoin L, Foti M. 2012. PPARs in liver diseases and cancer: epigenetic regulation by microRNAs. PPAR Res. 2012:757803.
- Piñeiro-Carrero VM, Piñeiro EO. 2004. Liver. Pediatrics 113:1097–1106.
- Preuss HG, Jarrell ST, Scheckenbach R, Lieberman S, Anderson RA. 1998. Comparative effects of chromium, vanadium and gymnema sylvestre on sugar-induced blood pressure elevations in SHR. J Am Coll Nutr. 17:116–123.
- Ramaiah S. 2007. A toxicologist guide to the diagnostic interpretation of hepatic biochemical parameters. Food Chem Toxicol. 45:1551–1557.
- Reddy BV, Sundari JS, Balamurugan E, Menon VP. 2009. Prevention of nicotine and streptozotocin treatment induced circulatory oxidative stress by bis-1,7-(2-hydroxyphenyl)-hepta-1,6-diene-3,5-dione in diabetic rats. Mol Cell Biochem. 331:127–133.
- Remels AH, Langen RC, Gosker HR, Russell AP, Spaapen F, Voncken JW, Schrauwen P, Schols AM. 2009. PPARgamma inhibits NF-kappaB-dependent transcriptional activation in skeletal muscle . Am J Physiol Endocrinol Metab. 297:E174–E183.
- Roskams TA, Theise ND, Balabaud C, Bhagat G, Bhathal PS, Bioulac-Sage P, Brunt EM, Crawford JM, Crosby HA, Desmet V, et al. 2004. Nomenclature of the finer branches of the biliary tree: canals, ductules, and ductular reactions in human livers. Hepatology. 39:1739–1745.
- Rothschild MA, Oratz M, Schreiber SS. 1972. Albumin synthesis (part 2). N Engl J Med. 286:816–821.
- Schumann G, Klauke R. 2003. New IFCC reference procedures for the determination of catalytic activity concentrations of five enzymes in serum: preliminary upper reference limits obtained in hospitalized subjects. Clin Chim Acta. 327:69–79.
- Selvi CGB, Panneerselvam A, Santhanam A. 2014. Hepatoprotective effects of brown algae Padina tetrastromatica against carbon tetrachloride induced hepatoxicity. Int J Pharm Bio Sci. 5:66–76.
- Senthilkumar K, Manivasagan P, Venkatesan J, Kim SK. 2013. Brown seaweed fucoidan: biological activity and apoptosis, growth signaling mechanism in cancer. Int J Biol Macromol. 60:366–374.
- Sezer MT, Akin H, Demir M, Erturk J, Aydin ZD, Savik E, Tunc N. 2007. The effect of serum albumin level on iron-induced oxidative stress in chronic renal failure patients. J Nephrol. 20:196–203.
- Shan W, Nicol CJ, Ito S, Bility MT, Kennett MJ, Ward JM, Gonzalez FJ, Peters JM. 2008. Peroxisome proliferator-activated receptor-beta/delta protects against chemically induced liver toxicity in mice. Hepatology. 47:225–235.
- Sharma SK, Arogya SM, Bhaskarmurthy DH, Agarwal A, Velusami CC. 2011. Hepatoprotective activity of the Phyllanthus species on tert-butyl hydroperoxide (t-BH)-induced cytotoxicity in HepG2 cells. Pharmacogn Mag. 7:229–233.
- Smith AJ. 2004. Medical and pharmaceutical applications of seaweed natural products – a review. J Appl Phycol. 16:245–262.
- Soldo-Jureša D, Metelko Ž. 2009. PPARγ-A new concept of treatment? Diabetol Croat. 38:23–29.
- Straus DS, Pascual G, Li M, Welch JS, Ricote M, Hsiang CH, Sengchanthalangsy LL, Ghosh G, Glass CK. 2000. 15-Deoxy-delta 12,14-prostaglandin J2 inhibits multiple steps in the NF kappa B signaling pathway. Proc Natl Acad Sci USA. 97:4844–4849.
- Waly MI, Al-Rawahi AS, Al Riyami M, Al-Kindi MA, Al-Issaei HK, Farooq SA, Al-Alawi A, Rahman MS. 2014. Amelioration of azoxymethane induced-carcinogenesis by reducing oxidative stress in rat colon by natural extracts. BMC Complement Altern Med. 14:60.
- Webster D. 1974. A study of the interaction of bromocresol green with isolated serum globulin fractions. Clin Chim Acta. 53:109–115.
- Wei XJ, Hu TJ, Chen JR, Wei YY. 2011. Inhibitory effect of carboxymethylpachymaran on cyclophosphamide-induced oxidative stress in mice. Int J Biol Macromol. 49:801–805.
- Zhang F, Lu Y, Zheng S. 2012. Peroxisome proliferator-activated receptor-γ cross-regulation of signaling events implicated in liver fibrogenesis. Cell Signal. 24:596–605.