Abstract
Glutathione reductase (GR; E.C. 1.6.4.2) is a flavoprotein that catalyzes the NADPH-dependent reduction of oxidized glutathione (GSSG). In this study we tested the effects of Al3+, Ba2+, Ca2+, Li+, Mn2+, Mo6+, Cd2+, Ni2+, and Zn2+ on purified bovine liver GR. In a range of 10 μM–10 mM concentrations, Al3+, Ba2+, Li+, Mn2+, and Mo6+, and Ca2+ at 5 μM–1.25 mM, had no effect on bovine liver GR. Cadmium (Cd2+), nickel (Ni2+), and zinc (Zn2+) showed inhibitory effects on this enzyme. The obtained IC50 values of Cd2+, Ni2+, and Zn2+ were 0.08, 0.8, and 1 mM, respectively. Cd2+ inhibition was non-competitive with respect to both GSSG (KiGSSG 0.221 ± 0.02 mM) and NADPH (KiNADPH 0.113 ± 0.008 mM). Ni2+ inhibition was non-competitive with respect to GSSG (KiGSSG 0.313 ± 0.01 mM) and uncompetitive with respect to NADPH (KiNADPH 0.932 ± 0.03 mM). The effect of Zn2+ on GR activity was consistent with a non-competitive inhibition pattern when the varied substrates were GSSG (KiGSSG 0.320 ± 0.018 mM) and NADPH (KiNADPH 0.761 ± 0.04 mM), respectively.
Introduction
The homodimeric flavin adenine dinucleotide (FAD)-containing glutathione reductase belongs to the family of NADPH (reduced nicotinamide adenine dinucleotide phosphate)-dependent oxidoreductases and exists in many pro- and eukaryotic organismsCitation1. Glutathione reductase (GR) is an important enzyme in maintaining the reduced state of the cell and in specialized pathwaysCitation2. Inhibition of this enzyme has been an attractive approach for various purposes, such as the development of antimalarial and anticancer agentsCitation3. The structure of a protein can be modified by some metals, and these structural alterations can cause modifications to its functionCitation4. Cd2+ and Ni2+ are heavy metals and both are toxic environmental pollutants. They cause damage to various organs and tissues following their acute or chronic exposuresCitation5,Citation6. The most sensitive parameter of metal toxicity for animals living in a chronically contaminated environment is the glutathione/oxidized glutathione (GSH/GSSG) ratio. The GSH/GSSG ratio was decreased in the livers of animals that were exposed to a high Cd2+ concentrationCitation7. In the cell, Cd2+ ions mainly accumulate in the cytosol (70%), are lower in the nucleus (15%), and lowest in the mitochondria and the endoplasmic reticulumCitation8. In the cytosol, Cd2+ binds to metallothioneinCitation9,Citation10, as well as to sulfhydrylic or histidylic groups of various proteinsCitation11. Cd2+ inhibits in vivo activities of various pancreatic proteases, such as trypsin, chymotrypsin, and carboxypeptidase A in miceCitation12. Cd2+ inhibited NADPH-cytochrome P450 reductase. The inhibitory effect is probably due to Cd2+ binding to the histidine residue of the apoenzymeCitation13. Rat kidney and liver arginase were inhibited by Cd2+ non-competitivelyCitation14. Cd2+ inhibited the flavokinase activity in a concentration-dependent manner. Furthermore, the enzyme could also be protected from the inhibitory effect of Cd2+ by GSH and dithriothreitol (DTT). This suggests that Cd2+ probably interacts with a reactive thiol group at or near the active site of the enzyme in bringing about its inhibitory effectCitation15.
Nickel is the most powerful metal carcinogen. This metal ion is capable of in vivo binding into the cell nucleus, subsequently causing promutagenic damage. Metal-mediated pathogenic effects, such as the enhancement of lipid peroxidation, the stimulation of inflammation, the inhibition of cellular antioxidant defenses, and the inhibition of DNA repair, may also contribute to this mechanismCitation16. Ni2+ inhibited bovine liver glutamate dehydrogenaseCitation17, and prolidaseCitation18 and horseradish peroxidase CCitation19.
Zinc is known to affect a variety of cellular proteins and phosphorylation-dependent signaling pathwaysCitation20. Elevated Zn2+ levels result in neuronal injury in vitroCitation21, and its accumulation may contribute to neurodegeneration and associated ischemiaCitation22. The mitochondria and energy metabolism are known as subcellular targets for toxic actions of Zn2+. This divalent metal can inhibit glycolysis, the tricarboxylic acid cycle, and complexes in the electron transport chainCitation23. Toxic doses of Zn2+ inhibit intestinal alkaline phosphataseCitation24, bovine heart mitochondrial cytochrome c oxidaseCitation25, glyceraldehyde-3-phosphate dehydrogenase in cultured mouse cortical neuronsCitation26, beta amylaseCitation27, hepatocyte fructokinase, aldolase-B in a dose-dependent mannerCitation28, and lamb kidney cortex glucose-6-phosphate dehydrogenase (G6PD)Citation29. It was found that monoamine oxidase-A is inhibited by ZnSO4 in monkey brainCitation30.
In our previous study, Cd2+, Ni2+, and Zn2+ showed good to moderate inhibitory effects on yeast GR. We also established that GR is inhibited by Zn2+ (up to 2 mM) and activated above this concentrationCitation31. In the present study, we investigated some metal inhibitors of bovine liver GR.
Materials and methods
Materials
Nicotinamide adenine dinucleotide phosphate, reduced form (NADPH), oxidized glutathione (GSSG), barium acetate (Ba(C2H3O2)2), and calcium chloride (CaCl2) were obtained from Sigma Chemical Co., MO, USA. Nickel sulfate (NiSO4) was obtained from the Needham Project, and zinc sulfate (ZnSO4) was of Analar grade, from Hopkin & Williams Ltd. Aluminum chloride (AlCl3) and lithium carbonate (Li2CO3) were from Fischer, manganese sulfate (MnSO4) was from Merck, and sodium molybdate (Na2MoO4) from BDH Chemicals Ltd.
Assay of glutathione reductase
Glutathione reductase activity was determined according to a modified version of Stall’s methodCitation32. The incubation mixture contained 100 mM sodium phosphate buffer, pH 7.4, 1 mM GSSG, 200 μM NADPH, and bovine liver GR. A decrease in absorbance of NADPH at 340 nm was monitored spectrophotometrically at 37°C. A unit of activity (U) was defined as the amount of enzyme that catalyzed the oxidation of 1 μmol of NADPH in 1 min under these conditions.
Enzyme purification
Bovine liver GR was purified using 2’,5’-adenosine phosphate (ADP)–Sepharose 4B and diethylaminoethyl (DEAE)–Sepharose Fast Flow columns. The enzyme was purified 5456-fold, with a yield of 38.4%Citation33.
Inhibition studies
Activities were measured upon adding Al3+, Ba2+, Ca2+, Cd2+, Li+, Mn2+, Mo6+, Ni2+, and Zn2+ at different concentrations in the assay mixture given above for GR measurement. Assays of GR in the presence of heavy metal ions were performed in the system without enzyme-inhibitor preincubation. The reactions were initiated by adding enzyme to a substrate–inhibitor mixture.
Statistical analysis of kinetic data
The data were analyzed and the kinetic constants calculated using the following equationsCitation34 by means of a non-linear curve-fitting program, Statistica:
where v is the reaction rate, [S] is the substrate concentration, Vm is the maximal velocity, [I] is the inhibitor concentration, and Km is the Michaelis–Menten constant (substrate concentration at half the maximal velocity (Vm)).
Results
Heavy metal intoxication leads to defects in cellular uptake mechanisms in the mammalian liver and kidneyCitation35. We have analyzed such inhibition of purified bovine liver GR with several heavy metals. The results indicate that concentrations of Al3+, Ba2+, Li+, Mn2+, and Mo6+ in a range of 10 μM–10 mM and Ca2+ at 5 μM–1.25 mM concentration have no effect on bovine liver GR. However, Cd2+, Ni2+, and Zn2+ at concentrations of 0–5 mM showed an inhibitory effect on this enzyme ().
Cd2+ is a very effective enzyme inhibitor; it inhibits many enzymes such as yeast GRCitation31. We have established that bovine liver GR is inhibited by Cd2+, and its IC50 value is 0.08 mM. The obtained IC50 values of Ni2+ and Zn2+ are 0.8 and 1 mM, respectively. Bovine liver GR is inhibited by much lower concentrations of Cd2+ than of the other metals. The kinetic characterization of the inhibition effects of these metals on GR has also been studied. The data were analyzed by a non-linear curve-fitting program.
Kinetics of cadmium inhibition of bovine liver GR
In this study, purified bovine liver GR was used in the inhibition experiments. We tried to determine the effects of Cd2+ as inhibitor on GR at different concentrations (). The effects of Cd2+ on GR from bovine liver were investigated using a Lineweaver–Burk double reciprocal plot and the initial velocity data were analyzed:
When GSSG was the varied substrate at constant NADPH concentration (0.1 mM), different fixed concentrations of Cd2+ (0–0.25 mM) were added into the assay mixture and initial velocities were measured. It can be seen that Cd2+ acted as a a non-competitive inhibitor with respect to GSSG (), and using the Statistica program, Ki was calculated as KiGSSG 0.221 ± 0.02 mM ().
When NADPH was the varied substrate, at constant and unsaturating GSSG concentration (0.7 mM), different fixed concentrations of Cd2+ (0–0.25 mM) were added into the assay mixture. It was shown that Cd2+ acted also as a non-competitive inhibitor with respect to NADPH, KiNADPH 0.113 ± 0.008 mM (, ).
Figure 2. Lineweaver–Burk double reciprocal plot of initial velocity against GSSG as varied substrate and Cd2+ (0–0.25 mM) as inhibitor at fixed NADPH (0.1 mM) concentration. ▵ 0.1 mM NADPH (constant), ×0.1 mM Cd2+, ○ 0.125 mM Cd2+, ◊ 0.15 mM Cd2+, + 0.25 mM Cd2+.
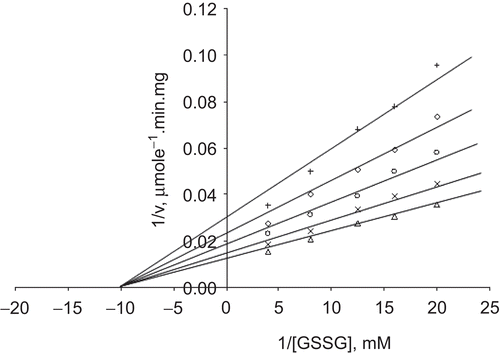
Figure 3. Lineweaver–Burk double reciprocal plot of initial velocity against NADPH as varied substrate and Cd2+ (0–0.25 mM) as inhibitor at fixed GSSG (0.7 mM) concentration. ▵ 0.7 mM GSSG (constant), ×0.1 mM Cd2+, ○ 0.125 mM Cd2+, ◊ 0.15 mM Cd2+, + 0.25 mM Cd2+.
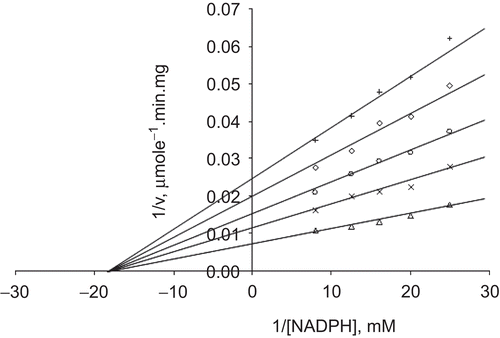
Table 1. Mechanism of inhibition of bovine liver GR by metal ions and Ki values.
Kinetics of nickel inhibition of bovine liver GR
In the inhibition of bovine liver GR by Ni2+, we used Ni2+ at different concentrations as an inhibitor. Kinetic studies on the inhibition of bovine liver GR by Ni2+ were carried out using a Lineweaver–Burk double reciprocal plot, and the initial velocity data were analyzed:
When GSSG was the varied substrate, at constant and unsaturating NADPH concentration (0.1 mM), different fixed concentrations of Ni2+ (0–0.6 mM) were added into the assay mixture and initial velocities measured. It can be seen that Ni2+ acted as a non-competitive inhibitor with respect to GSSG (), and using the Statistica program Ki was calculated as KiGSSG 0.313 ± 0.01 mM ().
When NADPH was the varied substrate, at constant and unsaturating GSSG concentration (0.7 mM), different fixed concentrations of Ni2+ (0–0.6 mM) were added into the assay mixture. It was shown that Ni2+ acted as an uncompetitive inhibitor with respect to NADPH, and Ki was calculated as KiNADPH 0.932 ± 0.03 mM (, ).
Kinetics of zinc inhibition of bovine liver GR
In the inhibition of bovine liver GR by Zn2+, we used Zn2+ at different concentrations as an inhibitor. The kinetic characterization of the inhibition effects of Zn2+ on bovine liver GR was investigated using a Lineweaver–Burk double reciprocal plot, and the initial velocity data were analyzed:
When GSSG was the varied substrate, at constant and unsaturating NADPH concentration (0.1 mM), different fixed concentrations of Zn2+ (0–0.4 mM) were added into the assay mixture and initial velocities measured. It can be seen that Zn2+ acted as a non-competitive inhibitor with respect to GSSG (), and using the Statistica program Ki was calculated as KiGSSG 0.320 ± 0.01 mM ().
When NADPH was the varied substrate, at constant and unsaturating GSSG concentration (0.7 mM), different fixed concentrations of Zn2+ (0–0.4 mM) were added into the assay mixture. It was shown that Zn2+ acted as a non-competitive inhibitor with respect to NADPH (), and Ki was calculated as KiNADPH 0.761 ± 0.04 mM ().
Discussion and conclusions
In this study we have investigated the effects of Al3+, Ba2+, Ca2+, Li+, Mn2+, Mo6+, Cd2+, Ni2+, and Zn2+ on bovine liver GR. Among these metals, Al3+, Ba2+, Li+, Mn2+, and Mo6+ (10 μM–10 mM) and Ca2+ (5 μM–1.25 mM) have no effect on bovine liver GR. We did not observe any inhibitory effect; these metals may not interact with the catalytic site of this enzyme. We have found that Cd2+, Ni2+, and Zn2+ are potent inhibitors of the enzyme. Inhibitory effects of heavy metals on mammalian tissues have been established in several biochemical and kinetic studies. It is known that the liver plays the major role in the detoxification of toxic chemicals. Therefore, we primarily examined the effects of some metals on bovine liver GR.
Metals undergo redox cycling, and free radicals are generated in the pathogenesis of liver injury in metal storage diseasesCitation36. Metal-mediated formation of free radicals causes various modifications to DNA bases, enhanced lipid peroxidation, and altered calcium and sulfhydryl homeostasis. Cd2+ and Ni2+ may cause toxic effects due to their interaction with sulfhydryl groups of proteins and depletion of GSHCitation11. Our results indicate that divalent metal inhibition on bovine liver GR activity may be as a result of the interaction of these metal ions with sensitive SH groups, which are located on this enzyme. The dimeric nature and SH groups of GR are both critical for its function, because all subunits contribute with essential residues to the constitution of its active siteCitation37. Histidine and tyrosine residues play an essential role for the catalytic site as proton donor/acceptorCitation38,Citation39. Notably, cysteines generate the enzyme’s redox-active dithiol. Cys-90 forms a disulfide bridge with the other Cys-90 of the peptide chainCitation40.This redox cysteine pair is affected by the metal insertion. Specific binding of the metal to this redox thiol/thiolate pair and the catalytic histidine of EH2 cause an inhibitory effect. It was demonstrated that both the reduced dithiol center and microenvironment of the isoalloxazine ring play an important role in the inactivation of GRCitation41.
The catalytic cycle of GR has two phases: a reductive and an oxidative half-reaction. During the reductive half-reaction, FAD is reduced by NADPH and reducing equivalents are transferred to a redox-active disulfide. In the oxidative half-reaction, the resulting dithiol reacts with the glutathione disulfide and GSSG is reduced to two GSHs at the active site of GRCitation38. It was found that the binding of a heavy metal to GR has an influence on the second half-reaction. Metal ions and GSSG compete essentially with the distal Cys of the EH2 stateCitation41. Thiols and selenols easily form complexes with heavy metal ions. Divalent metal ions may interact with the sulfhydryl groups near the active site of this enzyme. If metal ions bind out of the active site, there is no competition between the substrate and the metal ions. A non-competitive inhibitor may bind to a non-substrate site on a protein and distort it to the point of non-functionalityCitation34. Also, the inhibitory effect of metal ions on GR is considered as a possible interaction between glutathione and metal ions in the aqueous system. Ni2+ binds mainly with terminal NH2 and COO groups of glutamic acid, and complexes are formed of nearly octahedral symmetryCitation42. Cd2+ ions also bind to glutathione. This binding occurs mainly via deprotonation of the SH group, with the possible formation of either Cd(glutathione) or Cd(glutathione)2 complexesCitation43.
Cd2+ is a toxic heavy metalCitation44 and its acute exposure can result in damage to several tissues, such as liver and kidneyCitation45. Parenteral administration of Cd2+ to rats causes a rapid accumulation of this metal in the liver and results in severe hepatic injuryCitation46. Cd2+ interferes with antioxidant defense mechanisms and stimulates the production of reactive oxygen species, which may act as signaling molecules in the induction of gene expression and apoptosisCitation47. It was shown that Cd2+ inhibited non-competitively lamb kidney cortex G6PDCitation29 and bovine liver GR. Nickel is the most powerful metal carcinogenCitation16, and we have demonstrated that Ni2+ inhibits both yeastCitation31 and bovine liver GR. Zinc is an essential nutrient that is required in humans and animals for many physiological functions, including immune and antioxidant function, growth, and reproductionCitation48. Zinc, at low levels, has several basic functions. However, at high levels, this metal can be highly toxicCitation49. Yeast GR was inhibited by Zn2+ non-competitively, with respect to both GSSG and NADPHCitation31. Here, we report that Zn2+ non-competitively inhibited bovine liver GR. It was shown that Cd2+, Ni2+, Zn2+, and Hg2+ inhibited microsomal 7-ethoxyresorufin O-deethylaseCitation50 and cytochrome P450 reductase in fish liverCitation51.
Inhibition of GR has been employed as a tool in research for various purposes. Investigation of inhibitors of this enzyme is vital for drug development, such as antimalarial and anticancer drugs. Because GR is a key enzyme in the antioxidant system, this study may be useful for understanding the mechanism of oxidative damage associated with heavy metal toxicity.
Acknowledgment
This work is part of a project (0701101011 and 02 G085) supported by the Hacettepe University Scientific Research Unit.
Declaration of interest: The authors report no conflicts of interest.
References
- Bauer H, Fritz-Wolf K, Winzer A, Kühner S, Little S, Yardley V, et al. A fluoro analogue of the menadione derivative 6-[2’-(3’-methyl)-1’,4’-naphthoquinolyl]hexanoic acid is a suicide substrate of glutathione reductase. Crystal structure of the alkylated human enzyme. J Am Chem Soc 2006;128:10784–94.
- Rahman Q, Agabeydi P, Afaq F, Schiffmann D, Mossman BT, Kamp DW, et al. Glutathione redox system in oxidative lung injury. Crit Rev Toxicol 1999;29:543–68.
- Seefeldt T, Dwivedi C, Peitz G, Herman J, Carlson L, Zhang Z, et al. 2-Acetylamino-3-[4-(2-acetylamino-2-carboxyethylsulfanylcarbonylamino)-phenylcarbamoylsulfanyl]propionic acid and its derivatives as a novel class of glutathione reductase inhibitors. J Med Chem 2005;48:5224–31.
- Olmo R, Blanco MD, Teijón C, Socorro JM, Teijón JM. Studies of cadmium binding to hexokinase: structural and functional implications. J Inorg Biochem 2002;89:107–14.
- Shukla GS, Singhal RL. The present status of biological effects of toxic metals in the environment: lead, cadmium, and manganese. Can J Physiol Pharmacol 1984; 62:1015–31.
- Seilkop SK, Oller AR. Respiratory cancer risks associated with low-level nickel exposure: an integrated assessment based on animal, epidemiological, and mechanistic data. Regul Toxicol Pharmacol 2003;37:173–90.
- Swiergosz-Kowalewska R, Bednarska A, Kafel A. Glutathione levels and enzyme activity in the tissues of bank vole Clethrionomys glareolus chronically exposed to a mixture of metal contaminants. Chemosphere 2006;65:963–74.
- Casalino E, Sblano C, Landriscina C. Enzyme activity alteration by cadmium administration to rats: the possibility of iron involvement in lipid peroxidation. Arch Biochem Biophys 1997;346:171–9.
- Din WS, Frazier JM. Protective effect of metallothionein on cadmium toxicity in isolated rat hepatocytes. Biochem J 1985;230:395–402.
- Waalkes M, Goering P. Metallothionein and other cadmium-binding proteins: recent developments. Chem Res Toxicol 1990;3:281–8.
- Valko M, Morris H, Cronin MT. Metals, toxicity and oxidative stress. Curr Med Chem 2005;12:1161–208.
- Shimada H, Funakoshi T, Waalkes MP. Acute, nontoxic cadmium exposure inhibits pancreatic protease activities in the mouse. Toxicol Sci 2000;53:474–80.
- Casalino E, Calzaretti G, Sblano C, Landriscina C. Cadmium-dependent enzyme activity alteration is not imputable to lipid peroxidation. Arch Biochem Biophys 2000;383:288–95.
- Tormanen CD. Inhibition of rat liver and kidney arginase by cadmium ion. J Enzyme Inhib Med Chem 2006;21:119–23.
- Bandyopadhyay D, Chatterjee AK, Data AG. Effect of cadmium, mercury and copper on partially purified hepatic flavokinase of rat. Mol Cell Biochem 1997;167:73–80.
- Kasprzak KS. Possible role of oxidative damage in metal-induced carcinogenesis. Cancer Invest 1995;13:411–30.
- Ghobadi S, Nemat-Gorgani M, Golabi SM, Zare HR, Moosavi-Movahedi AA. Nickel-induced substrate inhibition of bovine liver glutamate dehydrogenase. J Enzyme Inhib Med Chem 2000;15:497–508.
- Miltyk W, Surazynski A, Kasprzak KS, Fivash MJ Jr, Buzard GS, Phang JM. Inhibition of prolidase activity by nickel causes decreased growth of proline auxotrophic CHO cells. J Cell Biochem 2005;94:1210–17.
- Keyhani J, Keyhani E, Zarchipour S, Tayefi-Nasrabadi H, Einollahi N. Stepwise binding of nickel to horseradish peroxidase and inhibition of the enzymatic activity. Biochim Biophys Acta 2005;1722:312–23.
- Wu W, Graves LM, Jaspers I, Devlin RB, Reed W, Samet JM. Activation of the EGF receptor signaling pathway in human airway epithelial cells exposed to metals. Am J Physiol 1999;277:924–31.
- Choi DW, Yokoyama M, Koh J. Zinc neurotoxicity in cortical cell culture. Neuroscience 1988;24:67–79.
- Koh JY, Suh SW, Gwag BJ, He YY, Hsu CY, Choi DW. The role of zinc in selective neuronal death after transient global cerebral ischemia. Science 1996;272:1013–16.
- Dineley KE, Votyakova TV, Reynolds IJ. Zinc inhibition of cellular energy production: implications for mitochondria and neurodegeneration. J Neurochem 2003;85:563–70.
- Yora T, Sakagishi Y. Comparative biochemical study of alkaline phosphatase isozymes in fish, amphibians, reptiles, birds and mammals. Comp Biochem Physiol B Biochem Mol Biol 1986;85:649–58.
- Kuznetsova SS, Azarkina NV, Vygodina TV, Siletsky SA, Konstantinov AA. Zinc ions as cytochrome C oxidase inhibitors: two sites of action. Biochemistry (Mosc) 2005;70:128–36.
- Sheline CT, Behrens MM, Choi DW. Zinc-induced cortical neuronal death: contribution of energy failure attributable to loss of NAD(+) and inhibition of glycolysis. J Neurosci 2000;20:3139–46.
- Dahot MU, Saboury AA, Moosavi-Movahedi AA. Inhibition of beta-amylase activity by calcium, magnesium and zinc ions determined by spectrophotometry and isothermal titration calorimetry. J Enzyme Inhib Med Chem 2004;19:157–60.
- Coyle P, Tichelman E, Pauw R, Philcox J, Rofe A. Zinc inhibition of hepatic fructose metabolism in rats. Biol Trace Elem Res 2003;92:41–54.
- Tandogan B, Ulusu NN. Effects of cadmium and zinc ions on purified lamb kidney cortex glucose-6-phosphate dehydrogenase activity. J Enzyme Inhib Med Chem 2006;21:225–30.
- Egashira T, Takayama F, Sakai K. Inhibition by Zn(2+) of A-form monoamine oxidase in monkey brain mitochondria. J Pharmacol Sci 2003;91:239–45.
- Tandogan B, Ulusu NN. The inhibition kinetics of yeast glutathine reductase by some metal ions. J Enzyme Inhib Med Chem 2007;22:489–95.
- Acan NL, Tezcan EF. Sheep brain glutathione reductase: purification and general properties. FEBS Lett 1989;250:72–4.
- Ulusu NN, Tandogan B. Purification and kinetic properties of glutathione reductase from bovine liver. Mol Cell Biochem 2007;303:45–51.
- Segel IH. Enzyme Kinetics, 3rd ed. Toronto: John Wiley and Sons, 1975:100–59.
- Markovich D, James KM. Heavy metals mercury, cadmium, and chromium inhibit the activity of the mammalian liver and kidney sulfate transporter sat-1. Toxicol Appl Pharmacol 1999;154:181–7.
- Barreto R, Kawakita S, Tsuchiya J, Mineli E, Pavasuthipaisit K, Helmy A, et al. Metal-induced oxidative damage in cultured hepatocytes and hepatic lysosomal fraction: beneficial effect of a curcumin/absinthium compound. Chin J Dig Dis 2005;6(1):31–6.
- Karplus PA, Schulz GE. Substrate binding and catalysis by glutathione reductase as derived from refined enzyme: substrate crystal structures at 2 A resolution. J Mol Biol 1989;210:163–80.
- Arscott LD, Veine DM, Williams CH Jr. Mixed disulfide with glutathione as an intermediate in the reaction catalyzed by glutathione reductase from yeast and as a major form of the enzyme in the cell. Biochemistry 2000;39:4711–21.
- Deonarain MP, Berry A, Scrutton NS, Perham RN. Alternative proton donors/acceptors in the catalytic mechanism of the glutathione reductase of Escherichia coli: the role of histidine-439 and tyrosine-99. Biochemistry 1989;28:9602–7.
- Untucht-Grau R, Schirmer RH, Schirmer I, Krauth-Siegel RL. Glutathione reductase from human erythrocytes: amino-acid sequence of the structurally known FAD-binding domain. Eur J Biochem 1981;120:407–19.
- Picaud T, Desbois A. Interaction of glutathione reductase with heavy metal: the binding of Hg(II) or Cd(II) to the reduced enzyme affects both the redox dithiol pair and the flavin. Biochemistry 2006;45:15829–37.
- Formicka-Kozłowska G, Kozłowski H, Jezowska-Trzebiatowska B. Metal glutathione interaction in aqueous solution. Nickel(II), cobalt(II) and copper(II) complexes with oxidized glutathione. Acta Biochim Pol 1979;2:239–48.
- Leverrier P, Montigny C, Garrigos M, Champeil P. Metal binding to ligands: cadmium complexes with glutathione revisited. Anal Biochem 2007;371:215–28.
- Bertin G, Averbeck D. Cadmium: cellular effects, modifications of biomolecules, modulation of DNA repair and genotoxic consequences (a review). Biochimie 2006;88:1549–59.
- Goering PL, Waalkes MP, Klaassen CD. Cadmium toxicity. In: Goyer RA, Cherian MG, eds. Handbook of Experimental Pharmacology; Toxicology of Metals, Biochemical Effects. New York: Springer-Verlag, 1994:189–214.
- Dudley RE, Svoboda DJ, Klaassen CD. Acute exposure to cadmium causes severe liver injury in rats. Toxicol Appl Pharmacol. 1982;65:302–13.
- Waisberg M, Joseph P, Hale B, Beyersmann D. Molecular and cellular mechanisms of cadmium carcinogenesis. Toxicology 2003;192:95–117.
- Sun JY, Jing MY, Weng XY, Fu LJ, Xu ZR, Zi NT, et al. Effects of dietary zinc levels on the activities of enzymes, weights of organs, and the concentrations of zinc and copper in growing rats. Biol Trace Elem Res 2005;107:153–65.
- Franco JL, Trivella DB, Trevisan R, Dinslaken DF, Marques MR, Bainy AC, et al. Antioxidant status and stress proteins in the gills of the brown mussel Perna perna exposed to zinc. Chem Biol Interact 2006;160:232–40.
- Bozcaarmutlu A, Arinc E. Inhibitory effects of divalent metal ions on liver microsomal 7-ethoxyresorufin O-deethylase (EROD) activity of leaping mullet. Mar Environ Res 2004;58:521–4.
- Bozcaarmutlu A, Arinc E. Effect of mercury, cadmium, nickel, chromium and zinc on kinetic properties of NADPH-cytochrome P450 reductase purified from leaping mullet (Liza saliens). Toxicol In Vitro 2007;21:408–16.