Abstract
A biochromatographic system was used to study the direct effect of carbon nanoparticles (CNPs) on the acetylcholinesterase (AChE) activity. The AChE enzyme was covalently immobilized on a monolithic CIM-disk via its NH2 residues. Our results showed an increase in the AChE activity in presence of CNPs. The catalytic constant (kcat) was increased while the Michaelis constant (Km) was slightly decreased. This indicated an increase in the enzyme efficiency with increase of the substrate affinity to the active site. The thermodynamic data of the activation mechanism of the enzyme, i.e. ΔH* and ΔS*, showed no change in the substrate interaction mechanism with the anionic binding site. The increase of the enthalpy (ΔH*) and the entropy (ΔS*) with decrease in the free energy of activation (Ea) was related to structural conformation change in the active site gorge. This affected the stability of water molecules in the active site gorge and facilitated water displacement by substrate for entering to the active site of the enzyme.
Introduction
Since the discovery of CNPs in 1991Citation1, extensive applications have been found in the physical, chemical, and material science fields. The advantages of CNPs, such as their high-surface area, favourable electronic properties, and electrocatalytic effect, have recently attracted considerable attention for the construction of bioreactorsCitation2. Due to the well-defined structure and the chemistry stability, CNPs are extensively used as the carrier platforms for constructing various electrochemical sensorsCitation3. Carbon nanotubes (CNTs) were found to increase the performance of bioreactors. This increase was related to the physicochemical properties of the novel support. André and al suggested that CNTs affect positively proximity and enzyme orientation for its interaction with the substrate and increase the adsorption of the freshly generated product on the support in the monolithic bioreactorCitation4. However, despite the increase use of CNPs in the development of new enzymatic bioreactors, the direct effect of CNPs on the enzyme activity is still poorly studied. AChE is largely used in the bioreactorsCitation5. A biosensor based on inhibition of AChE is widely used for environmental controlCitation6,Citation7. An AChE bioreactor is the main technique currently used to test new AChE inhibitors in order to determine their inhibitory potency and mechanismCitation8–10. Thanks to the extensive use of AChE and to the available data, AChE was used in this work to study the direct effect of CNPs on the enzyme activity using a biochromatographic approach.
Experimental and method
Apparatus
The high performance liquid chromatography (HPLC) system consisted of a Hewlett-Packard quaternary pump (1050), an Agilent (G1365B, series 1100) UV-Visible detector (Paris, France) and a Rheodyne 7725i injection valve (Cotati, CA, USA) fitted with a 20 µL sample loop. UV-Visible Spectrophotometer U-2001 (Hitachi) was used for the rapid screening test. A monolithic EDA CIM-disk (12 mm × 3 mm i.d.) from BIA Separations was purchased from Interchim (Montluçon, France). This support was characterized by a high-binding capacity: 1 mL of the CIM support can bind 30 mg of human serum albumin (Assurance quality file-CIM disk – Interchim). The AChE stationary phase (i.e. the AChE disk) was prepared as below.
Chemicals
Acetylcholinesterase (AChE) type V-S from Electrophorus Electricus (electric eel) and acetylthiocholine (ATCh) chloride were purchased from Sigma-Aldrich (Saint-Quentin Fallavier-France). Glutaraldehyde, Ellman’s reagent (DTNB (5,5’ dithiobis (2-nitrobenzoic acid))) and sodium cyanoborohydride were obtained from Interchim (Montluçon, France). Monoethanolamine and hydrogen peroxide were obtained from Sigma-Aldrich (Saint-Quentin Fallavier, France). Water was obtained from an Elgastat option water purification system (Odil, Talant, France) fitted with a reverse osmosis cartridge. Sodium chloride, sodium dihydrogenophosphate and di-natriumhydrogenophosphate were obtained from Prolabo (Paris, France). Single walled CNTs (SWNTs) (0.7–1.3 × 700 nm), COOH functionalized single walled CNTs COOH-SWNTs (4–5 nm x 0.5–1.5 μm) and fullerene (C60) were obtained from Sigma-Aldrich (Saint-Quentin Fallavier-France).
Immobilization of AChE on monolithic EDA CIM-disk
The immobilization of AChE via the amino groups on a monolithic EDA CIM-disk activated with glutaraldehyde was carried out as mentioned in previous papersCitation10,Citation11.
After immobilization, the enzyme solution was analyzed using Ellman’s method in order to determine the unreacted enzyme unitsCitation12. The EDA CIM-disk was then washed, inserted in the appropriate holder, connected to the HPLC system and conditioned with a mobile phase consisting of phosphate buffer (0.05 M, pH 7.0) for 1 h at a flow-rate of 0.5 mL/min.
Preparation of CNPs solutions and relative AChE activity (RAA) evaluation
Three types of CNPs were used in this study; single walled CNTs (SWNTs), COOH functionalized single walled CNTs (COOH-SWNTs) and fullerenes (C60). Solution or dilute suspension in water/methanol mixture were prepared daily at concentration of 0.001 to 0.1 mg/mL of nanoparticles. Stock solutions were stirred before every injection of the test particle. The enzyme-disk was equilibrated at 37°C for 30 min with a phosphate buffer solution (pH = 7.4, 0.05 M) containing 1.10-4 M DTNB and 0.05 M sodium chloride. To investigate the effect of carbon nanoparticles on the AChE activity, 20 μL of a solution containing x mg/mL of CNPs and a fixed substrate concentration, i.e. ATCh (250 mM), were injected into the chromatographic system and the relative peak areas were integrated (A). The peak area at 412 nm was related to the yellow anion (YA) result by the interaction of thiocholine with Ellman’s reagent. Thus this peak was proportional to the enzyme activity, x varied from 0 to 0.06 mg/mL (for concentrations higher than 0.08 mg/L, unstable column pressure was observed). Nine values were included in this range. The Relative AChE Activity (RAA) (i.e. AChE activity relative to the value at x = 0) was then calculated. The disk was washed after every injection of CNPs by the mobile phase for 30 min at flow rate of 1 mL/min.
Kinetic parameters Vmax and Km evaluation
The enzyme-disk was equilibrated at 37°C for 30 min with a phosphate buffer solution (pH = 7.4, 0.05 M) containing 1.10-4 M DTNB and 0.05 M sodium chloride. To investigate the effect of CNPs on the AChE activity and thus on the kinetic parameters, aliquots of 20 µL containing CNPs at x mg/mL and ATCh at increasing concentration (range comprised between 3 and 300 mM) were injected into the chromatographic system and the relative peak areas at 412 nm corresponding to the yellow anion YA were integrated (A). The Michaelis–Menten trend was thus found by plotting the rate of enzymatic reaction (V) against the substrate concentration [ATCh]. The rate of the enzymatic reaction was expressed as Δ(areaYA/min) given by the expression area(YA)/(time(min), i.e. A/t(min). The time t indicates the reaction time defined by the time for complete elution of the product YA in the immobilized enzymatic microreactor, which is dependent upon flow-rate. Kinetic parameters Vmax and Km were obtained thanks to the Lineweaver–Burk equation, which is a linear transformation of the Michaelis–Menten plot. At a flow rate of 0.5 mL/min, the contact time was 0.68 min (the disk bed volume is 0.34 mL).
Results and discussion
A rapid screening analysis of CNPs effect
A rapid screening test to determine the CNPs effect on the non-immobilized enzyme activity was carried out using a spectrometric technique. An increase concentration of CNPs was added to the assay solution containing a fixed concentration of substrate ATCh, AChE and Ellman’s reagent at pH = 8. i.e. (50 µL CNPs (x g/L) was added to a sample contains 2600 µL DTNB (2.10-4 M), 275 µL PBS (pH = 8, 0.1 M), 25 µL AChE (1.25 U/1 mL) and 50 µL ATCh (100 mM)). The absorbance at 412 nm of the relative samples was proportional to the enzyme activity. The relative AChE activity (RAA) (i.e. AChE activity relative to CNPs concentration = 0) was calculated for every concentration of the nanoparticles every 15 s until 3 min (an example of plot was given for SWNTs in ). A RAA> 1 was observed for all studied nanoparticles where the highest RAA values were for the non functionalized SWNTs and the lowest values were for fullerene (C60). However, the RAA increased with the increase of the concentration of the nanoparticles (). After 75 s, the RAA values decreased to one indicating the catalysis of all the substrate in the medium. These results showed that the CNPs increased the catalysis rate of the AChE and this activation depended on both the CNPs concentration and its structure.
Kinetic study
The RAA for the immobilized enzyme was determined for the three CNPs using our biochromatographic process (see experimental section “Preparation of CNPs solutions and relative AChE activity (RAA) evaluation”***). An increased AChE activity was observed for all the studied CNPs (). These results confirmed the fact that the immobilization process did not change the catalytic properties of the enzyme and the chromatographic disk can be used to study the activation mechanism. The non-functionalized SWNTs showed the highest values of RAA followed by COOH-SWNTs and then by fullerene (C60) (). The catalysis rate (ΔA/t) was plotted versus the injected substrate ATCh concentration for a CNPs concentration x = 0.04 mg/mL (). The corresponding Michaelis–Menten and Lineweaver–Burk plots were obtained and the effect of CNPs on the Km and Vmax values was evaluated ( and ). For example, the Lineweaver–burk equation was
Figure 2. Relative AChE Activity (RAA) for the immobilized enzyme (i.e. the AChE activity relative to the value at x = 0) versus x (mg/mL) of CNPs.
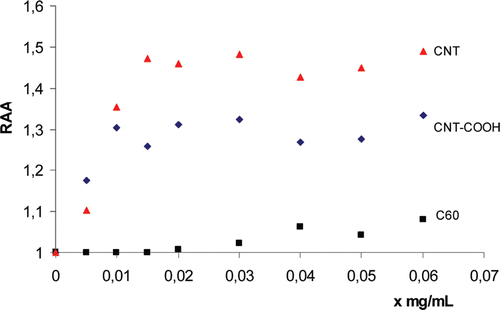
Figure 3. AChE catalysis rate (ΔA/min) versus substrate (ATCh) concentration at 0 and 0.04 mg/mL of SWNTs (Michaelis–Menten curve).
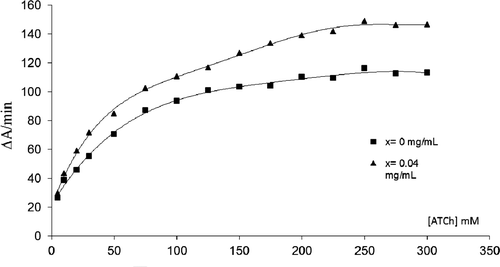
Figure 4. 1/velocity (1/V) versus 1/[substrate] (1/[ATCh]) at A) x =0 and B) x = 0,04 mg/mL of SWNTs (Lineweaver–Burk plot).
![Figure 4. 1/velocity (1/V) versus 1/[substrate] (1/[ATCh]) at A) x =0 and B) x = 0,04 mg/mL of SWNTs (Lineweaver–Burk plot).](/cms/asset/c71de80e-f99d-4b17-ab2b-82f3044090e3/ienz_a_705835_f0004_b.gif)
(1/V) (mM-1 min) = 0.177(1/[ATCh])mM-1 + 0.0052 (r2 = 0.994) at x = 0 mg/mL of CNPs in the medium. As shown in , no change in the Michaelis–Menten plot shape was observed with an r2 > 0.9988. This means that the CNPs induced no effect on the main catalysis mechanism of the enzyme active siteCitation13. The catalysis rate and the Vmax values were higher at the presence of CNPs in the medium ( and ), while a little decrease was observed on the Km. These data indicated classical activation kinetic with an increase in the substrate affinity to the active siteCitation14–16 and suggested that CNPs affect positively proximity and enzyme orientation for its interaction with the substrate. This can be achieved by a structural orientation of histidine residue in the active site gorge, by increasing affinity of substrate to the peripheral site or by increasing the rate of substrate entering to the active site following a structural conformational change. No direct effect was observed of carbon nanoparticles on the ATCh hydrolysis. This was confirmed by the saturation plateau of the Michaelis–Menten curves at the steady state indicating no additional hydrolysis of the substrate after achieving the maximum velocity (Vmax) by the active site. The Michaelis–Menten approach assumed that a rapid equilibrium was established between the free reactants (enzyme (E) + substrate (S)) and the transition state complex (ES), followed by slower conversion of the ES complex back to free enzyme (E) and product (P). However there were a series of rapid chemical events following ES complex formation. For simplicity, the overall rate for these collective chemical steps can be described by a single first-order rate constant kcat proportional to the Vmax values (i.e. kcat = Vmax/[E0] where [E0] was the immobilized AChE concentrationCitation13). The catalytic efficiency of an enzyme is best defined by the ratio of the kinetic constants, kcat/KmCitation17. This ratio increased with the presence of CNPs in the medium, indicating an increase in the catalytic efficiency of the enzyme (). The AChE active site consists of a gorge that is 2 nm deep, which is lined with aromatic residuesCitation18,Citation19. The gorge contains two sites of ligand binding; an acylation site near the base of the gorge and a peripheral site at the mouth of the gorge, about 10–20 Å from the acylation site. The peripheral site of AChE is also named the “Aromatic gorge” because nearly 40% of its lining is composed of a ring consisting of 14 conserved aromatic amino acidsCitation20 and many other hydrophobic residues. The inhibition and activation of acetylcholinesterase are linked to the functions of the hydrophobicity index, presence of a cationic cluster, and the accessible arginine residueCitation14,Citation21. The activation of the AChE in presence of CNPs might be related to the interaction between the aromatic residues of the peripheral site and the hydrophobic surface of the CNPs. That led to specific conformation change and increased the substrate interaction with the active site. This was confirmed by the decrease of the activation effect when the nanoparticles were functionalized by hydrophilic residues. For example the RAA was decreased from 1.45 for the non-functionalized CNT to 1.27 for the COOH-CNT at x = 0.05 mg/mL (). On the other hand, the hydrophobic attraction depends on the geometry shape of the hydrophobic surface and the angle of contactCitation22. This explained why the spherical structure of fullerene C60 showed less effect on the AChE activity than the CNTs ( and ).
Table 1. Michaelis constant (Km mmol), the maximum velocity (Vmax mmol/min), the catalytic constant (kcat mmol/min/U) and the catalytic efficiency (kcat/Km) for x mg/mL of CNPs.
Thermodynamic origins of the AChE activation
An analysis of the changes in enzyme activity that accompany changes in temperature is a practical method to explain the substrate-enzyme interaction and the activation mechanism. The rate of a reaction can be related to the activation energy (Ea) for attaining the reaction transition state by the Arrhenius equation:
and
where T, KB, h, R were respectively the absolute temperature (K), Boltzmann constant (1.38 × 10–23 J/K), Planck’s constant (6.626 × 10–34 Js) and Gas constant (8.314 J K−1 mol−1). From the straight line of eq.(1) the enthalpy ΔH*(J/mol) of the AChE activation was calculated as slope and the entropy ΔS* (J/mol/K) of the AChE activation from ln(KB/h) + ΔS*/R as intercept on Y-axis from Arrhenius plotCitation13,Citation23.
There was a decrease in Vmax with the decrease in the temperature (). The activation energy (Ea) calculated from Arrhenius plots for AChE at 37°C was 54 kJ/mol ( and ). The positive Ea was because it represents energy which must be put in to reach the unlikely transition state. This value decreased with the presence of CNPs. For example, it decreased to 53 kJ/mol in presence of 0.04 mg/mL of SWNTs. This means that the enzyme is more easily activated to react with the substrate in the presence of CNPs. For many reactions the decreased free energy in the transition state will result from decreased enthalpy. In other cases, the lowered Ea might be expected to result from high entropy in the transition state as structured interface water is released to the bulk phaseCitation24. In this study, the CNPs increased the enthalpy of AChE activation (). The lowered activation energy was result then from the increase of ΔS* (increased from –104 to –72 J/mol/K in presence of SWNTs). Therefore, the increase of substrate affinity to the enzyme when CNPs was added to the medium was not related to change of its interaction with the peripheral anionic binding site. The increase of ΔH* and ΔS* with decrease in the Ea indicated a structural conformation change in the active site gorge. This affected the stability of water molecules in the active site gorge and facilitated water displacement by substrate for entering to the active siteCitation24–26.
Table 2. Activation energy (Ea), enthalpy (ΔH*) and entropy (ΔS*)of attaining the reaction transition state (AChE-Substrate, ATCh) versus x mg/mL of single carbon nanotubes (SWNTs).
Conclusion
These results explained the direct effect of CNPs on the AChE. The CNPs, and in particularly the non-functionalized SWNTs showed a direct effect on the AChE activity without significant change in the association and catalysis mechanism. These nanoparticles can be used at low concentration in the preparation of AChE bioreactors to increase its efficiency. On the other hands, the toxicity of CNPs toward this enzyme and other enzymes must be further evaluated before using in the therapeutic domain.
Declaration of interest
The authors reports no conflicts of interest.
References
- Iijima S. Helical, microtubules of graphitic carbon. Nature 1991;354:56–58.
- Lin Y, Taylor S, Li H, Fernando KAS, Qu L, Wang W, Gu L, Zhou B, Sun YP. Advances toward bioapplications of carbon nanotubes. J Mat Chem. 14; 2004: 527–541.
- Hu C, Hu H. Carbon nanotube-based electrochemical sensors: principles and applications in biomedical systems. J Sensor. 2009, ID 187615, 40 pages.
- André C, Agiovlasileti D, Guillaume YC. Peculiarities of a novel bioenzymatic reactor using carbon nanotubes as enzyme activity enhancers: application to arginase. Talanta 2011;85:2703–2706.
- Pohanka M, Jun D, Kalasz H, Kuca K. Cholinesterase biosensor construction - a review. Protein Pept Lett 2008;15:795–798.
- Bucur B, Fournier D, Danet A, Marty JL. Biosensors based on highly sensitive acetylcholinesterases for enhanced carbamate insecticides detection. Analytica Chimica Acta 2006;562:115–121.
- Kok FN, Hasirci V. Determination of binary pesticide mixtures by an acetylcholinesterase - choline oxidase biosensor. Biosensors & Bioelectronics 2004;19:661–665.
- He P, Davies J, Greenway G, Haswell SJ. Measurement of acetylcholinesterase inhibition using bienzymes immobilized monolith micro-reactor with integrated electrochemical detection. Anal Chim Acta 2010;659:9–14.
- Bartolini M, Cavrini V, Andrisano V. Characterization of reversible and pseudo-irreversible acetylcholinesterase inhibitors by means of an immobilized enzyme reactor. J Chromatogr A 2007;1144:102–110.
- Ibrahim F, Guillaume YC, Thomassin M, André C. Magnesium effect on the acetylcholinesterase inhibition mechanism: a molecular chromatographic approach. Talanta 2009;79:804–809.
- Bartolini M, Cavrini V, Andrisano V. Monolithic micro-immobilized-enzyme reactor with human recombinant acetylcholinesterase for on-line inhibition studies. J Chromatogr A 2004;1031:27–34.
- Ellman GL, Courtney KD, Andres V Jr, Feather-Stone RM. A new and rapid colorimetric determination of acetylcholinesterase activity. Biochem Pharmacol 1961;7:88–95.
- Copeland RA. Enzymes. A practical introduction to structure, mechanism, and data analysis, Second ed. Analyt Biochem 2001;291:172.
- Schallreuter KU, Elwary SM, Gibbons NC, Rokos H, Wood JM. Activation/deactivation of acetylcholinesterase by H2O2: more evidence for oxidative stress in vitiligo. Biochem Biophys Res Commun 2004;315:502–508.
- Yang M, Zhang J, Zhu KY, Xuan T, Liu X, Guo Y, Ma E. Increased activity and reduced sensitivity of acetylcholinesterase associated with malathion resistance in a field population of the oriental migratory locust, Locusta migratoria manilensis (Meyen). Pesticide Biochemistry and Physiology 2008;91:32–38.
- André C, Ibrahim F, Gharbi T, Herlem G, Guillaume YC. Experimental studies of OH° radical/pressure dependence of arginase activity using a molecular chromatography approach. J Chromatogr B Analyt Technol Biomed Life Sci 2010;878:2826–2830.
- Bustos-Jaimes I, Mora-Lugo R, Calcagno ML, Farrés A. Kinetic studies of Gly28:Ser mutant form of Bacillus pumilus lipase: changes in k(cat) and thermal dependence. Biochim Biophys Acta 2010;1804:2222–2227.
- Rosenberry TL, Rabl CR, Neumann E. Binding of the neurotoxin fasciculin 2 to the acetylcholinesterase peripheral site drastically reduces the association and dissociation rate constants for N-methylacridinium binding to the active site. Biochemistry 1996;35:685–690.
- De Ferrari GV, Canales MA, Shin I, Weiner LM, Silman I, Inestrosa NC. A structural motif of acetylcholinesterase that promotes amyloid β-peptide fibril formation. Biochemistry 2001;40:10447–10457.
- Harel M, Schalk I, Ehret-Sabatier L, Bouet F, Goeldner M, Hirth C, Axelsen PH, Silman I, Sussman JL. Quaternary ligand binding to aromatic residues in the active-site gorge of acetylcholinesterase. Proc Nat Acad Sci 1993;90:9031–9035.
- Ranaei-Siadat SO, Riazi GH, Sadeghi M, Chang LS, Lin SR, Eghtesadi-Araghi P et al. Modification of substrate inhibition of synaptosomal acetylcholinesterase by cardiotoxins. J Biochem Mol Biol 2004;37:330–338.
- Yuyang L, Xianqiong Chen JHX, Xin JH. Super-hydrophobic surfaces from a simple coating method: a bionic nanoengineering approach. Nanotechnology 2006;17: 3259–3265.
- Low PS, Bada JL, Somero GN. Temperature adaptation of enzymes: roles of the free energy, the enthalpy, and the entropy of activation. Proc Nat Acad Sci 1973;70:430–432.
- Loftfield RB, Eigner EA, Pastuszyn A, Lovgren TN, Jakubowski H. Conformational changes during enzyme catalysis: role of water in the transition state. Proc Nat Acad Sci 1980;77:3374–3378.
- Koellner G, Kryger G, Millard CB, Silman I, Sussman JL, Steiner T. Active-site gorge and buried water molecules in crystal structures of acetylcholinesterase from Torpedo californica. J Mol Biol 2000;296:713–735.
- Henchman RH, Tai K, Shen T, McCammon JA. Properties of water molecules in the active site gorge of acetylcholinesterase from computer simulation. Biophys J 2002;82:2671–2682.