Abstract
Diatom genome sequences clearly reveal the presence of different systems for HCO3− uptake. Carbon-concentrating mechanisms (CCM) based on HCO3− transport and a plastid-localized carbonic anhydrase (CA, EC 4.2.1.1) appear to be more probable than the others because CAs have been identified in the genome of many diatoms. CAs are key enzymes involved in the acquisition of inorganic carbon for photosynthesis in phytoplankton, as they catalyze efficiently the interconversion between carbon dioxide and bicarbonate. Five genetically distinct classes of CAs exist, α-, β-, γ-, δ- and ζ and all of them are metalloenzymes. Recently we investigated for the first time the catalytic activity and inhibition of the δ-class CA from the marine diatom Thalassiosira weissflogii, named TweCA. This enzyme is an efficient catalyst for the CO2 hydration and its inhibition profile with sulfonamide/sulfamate and anions have also been investigated. Here, we report the detailed biochemical characterization and chemico-physical properties of the δ-CA of T. weissflogii. The δ-CA encoding gene was cloned and expressed in Artic Express cells and the recombinant protein purified to homogeneity. Interesting to note that TweCA has no intrinsic esterase activity with 4-nitrophenyl acetate (pNpA) as substrate although the phylogenetic analysis showed that δ-CAs are closer to the α-CAs than to the other classes of such enzymes.
Introduction
Phytoplankton is composed of photosynthetic prokaryotes and eukaryotes. Diatoms are the most important group of eukaryotic phytoplankton and responsible for about 40% of the total primary production in the oceansCitation1,Citation2. Diatoms are crucial for the biological carbon production. Because of their heavy siliceous walls, they export carbon to the bottom of the ocean, essential for the survival of all organisms living beneath the photic zone of the water column, and contribute significantly to the long-term sequestration of atmospheric CO2Citation1–4. As autotrophic organisms, diatoms require carbon dioxide and light for growthCitation2. The diffusion of CO2 in water is slower than in air which greatly limits the carbon acquisition by phytoplankton. The majority of microalgae have developed two types of carbon-concentrating mechanisms (CCMs) to accumulate CO2 close to the ribulose-1,5-bisphosphate carboxylase oxygenase (RuBisCO)Citation2,Citation5,Citation6: (a) CO2 and HCO3− are transported as inorganic forms by means of transporters and carbonic anhydrases (CAs, EC 4.2.1.1); (b) HCO3− is fixed into C4 compounds in a manner similar to that used by the C4 plants. Diatoms are characterized by the involvement of CCMs including active uptake of both CO2 and HCO3− and single cell C4 like metabolism.
Diatom genome sequences clearly reveal the presence of different systems for HCO3− uptake similar to that of multicellular organisms. Phaeodactylum tricornutum, for example, is a diatom containing a plastid-targeted β-CA involved in the CO2 supply to Rubisco, similar to the CCMs that are believed to exist in cyanobacteriaCitation7,Citation8. Intriguingly, in the genome of other diatoms, the plastid-localized β-CA does not appear to be present, suggesting that diatoms could posses a diversity of CCMsCitation2,Citation3. Photosynthesis in diatoms depends on a form of C4-like biochemistry and there is evidence for the role for phosphoenolpyruvate carboxylase (PEPC) in diatom photosynthesis, presumably via a C4-like CCMCitation9,Citation10. It has been hypothesized that the product of PEPC, oxaloacetate (OAA), is transported by a dedicated dicarboxylic acid transport system into the chloroplast where it is subsequently decarboxlyated by PEP carboxykinase (PCKase), thus providing a source of concentrated CO2 to RuBisCOCitation2,Citation9,Citation10. However, the CCM system based on HCO3− transport and a plastid-localized CA appears to convince more than the other CCMs because CAs have been identified in the genome of the diatoms, mostly belonging to the poorly investigated δ- and ζ-classesCitation11–15. Probably these enzymes have a significant impact on primary carbon metabolism than originally considered.
CAs are key enzymes involved in the acquisition of inorganic carbon for photosynthesis in phytoplankton, as they catalyze efficiently the interconversion between carbon dioxide and bicarbonate. Five genetically distinct classes of CAs exist, α-, β-, γ-, δ- and ζ and all of them are metalloenzymes, using Zn(II), Cd(II) or Fe(II) at their active sitesCitation16–20. The δ and ζ families are the least investigated onesCitation11,Citation12. In a recent paper, we studied the catalytic activity and inhibition of the δ-class CA from the marine diatom Thalassiosira weissflogii, named TweCA. The enzyme was an efficient catalyst for the CO2 hydration, with a kcat of 1.3 × 105 s−1 and a kcat/KM of 3.3 × 107 M−1 s−1 Citation21,Citation22. Its sulfonamide/sulfamate and anions inhibition profiles have been investigated, tooCitation21,Citation22. Here, we report, for the first time, the detailed biochemical characterization and chemico-physical properties of the δ-CA of T. weissflogii. The δ-CA encoding gene was cloned and expressed in Escherichia coli and the recombinant protein purified to homogeneity. Interesting to note that TweCA has no esterase activity with 4-nitrophenylacetate (pNpA) as substrate although the phylogenetic analysis showed that δ-CAs are closer to the α-CAs than to the other classes of CAs and this activity has been reported in the literatureCitation23.
Materials and methods
Sequence alignment
Alignment of amino acid sequences was performed using the program Bl2seq, a new computer program for creating alignments of two protein sequencesCitation24.
Phylogenetic analysis
A phylogenetic tree was constructed using the program PhyML 3.0 searching for the tree with the highest probabilityCitation25.
Cloning, protein expression and purification
The synthetic T. weissflogii gene encoding TweCA was designed and produced by Life Technologies (Invitrogen, Carlsbad, CA), a company specialized in gene synthesis. The gene contains NdeI and XhoI restrictions sites at the 5′ and 3′ ends, respectively. The synthetic gene was ligated into the expression vector pET15-b (Novagen) by T4 DNA ligase to form the expression vector pET15-b/TweCA. Arctic express DE3 competent cells (Agilent) were transformed with pET15-b/TweCA, grown at 20 °C and induced with 1 mM IPTG. 0.5 mM of ZnSO4 was added after 30 min incubation for uptake in the expressed protein. Arctic express competent cells have been engineered for improved protein processing at low temperatures, which represents one strategy for increasing the recovery of soluble protein. At 6 h post-induction, cells were harvested and disrupted by sonication at 4 °C. Following centrifugation, the supernatant was loaded onto HIS-Select HF Nickel Affinity Gel (Sigma–Aldrich) and the protein was eluted with 250 mM imidazole. At this stage of purification the enzyme was at least 90% pure.
SDS-PAGE
Sodium dodecyl sulfate (SDS)-polyacrylamide gel electrophoresis (PAGE) was performed as described previously, using 12% gelsCitation26.
Size exclusion chromatography
Recombinant TweCA was subject to high-performance liquid chromatography (HPLC) size exclusion chromatography under native conditions to determine the oligomeric state of the protein. The sample was analyzed on an HPLC Ultimate 3000 (Dionex) equipped with a diode-array UV/VIS detector. Data were collected using the Dionex Chromelon Chromatography Data System. The separation was executed on a Phenomenex column, the BioSep-SEC-s2000 (300 × 4.6 mm) calibrated with bovine catalase (240 kDa), rabbit aldolase (158 kDa), chicken egg albumin (45 kDa) and bovine cytochrome C (12.5 kDa). The mobile phase was 50 mM sodium phosphate pH 6.8 with 0.3 M sodium chloride in water running at a flow rate of 1 mL/min. The protein sample injection volume was 20 μl corresponding to 10 µg of purified TweCA. The detection UV wavelength was set at 220 and 280 nm. The column temperature was 25 °C.
Enzyme kinetic
An applied photophysics stopped-flow instrument was used for assaying the CA catalyzed CO2 hydration activityCitation27. Phenol red (at a concentration of 0.2 mM) was used as indicator, working at the absorbance maximum of 557 nm, with 20 mM Hepes (pH 7.5, for α-CAs) or 20 mM TRIS (pH 8.3 for the β- and γ-CAs) as buffers, and 20 mM NaClO4 for maintaining a constant ionic strength, following the initial rates of the CA-catalyzed CO2 hydration reaction for a period of 10–100 s. The CO2 concentrations ranged from 1.7 to 17 mM for the determination of the kinetic parameters and inhibition constants. For each inhibitor at least six traces of the initial 5–10% of the reaction have been used for determining the initial velocity. The uncatalyzed rates were determined in the same manner and subtracted from the total observed rates. Stock solutions of inhibitor (10–50 mM) were prepared in distilled-deionized water and dilutions up to 0.01 µM were done thereafter with the assay buffer. Inhibitor and enzyme solutions were preincubated for 15 min at room temperature prior to assay, in order to allow the formation of the enzyme–inhibitor (E–I) complex or for the eventual active site mediated hydrolysis of the inhibitor. The inhibition constants were obtained by non-linear least-squares methods using PRISM 3, as reported earlier, and represent the mean from at least three different determinationsCitation28. All CA isoforms were recombinant ones obtained in-house as reported earlierCitation28.
Hydratase enzyme activity
CA activity assay was a modification of the procedure described by Chirica et al.Citation29. The assay was based on the monitoring of pH variation due to the catalyzed conversion of CO2 to bicarbonate. Bromothymol blue was used as the indicator of pH variation. The assay was performed at 0 °C adding 0.5 mL ice-cold CO2-saturated water to 0.5 mL mixtures of 25 mM Tris-SO4 buffer containing the enzyme at different concentrations. The details concerning the assay have been described by Capasso et al.16
Esterase enzyme activity
Esterase activity for pNpA hydrolysis was determined at 0 °C using a modification of the method proposed by Armstrong et al.Citation30. The reaction mixture contained 0.3 mL of freshly prepared 3 mM pNpA and 0.7 mL of 15 mM Tris sulphate buffer, pH 7.6. Forty micrograms of TweCA were added, and the catalyzed reaction was monitored reading the increase in A348 nm for 5 min. The catalyzed reactions were corrected for the non-enzymatic reaction. One enzyme unit was defined as the amount capable of producing a ΔOD348nm = 0.001 in 5 min. Acetazolamide inhibitor at different concentrations (1–10 µM) was used as control to verify that the hydrolysis of pNpA was intrinsic to the catalytic site of TweCA. Esterase activity was compared with that of the bovine α-CA (bCA II). All the reactions were performed in triplicate and in parallel with bovine CA.
Effect of temperature on the enzyme stability
To determine the enzyme stability of TweCA at different temperatures, the enzyme was incubated at 25, 40, 50, 60, 70 and 80 °C for different times (10, 30 and 60) at the concentration of 0.16 mg/mL in 10 mM Tris/HCl, pH 8.3. Enzyme aliquots (0.6 µg) were withdrawn at appropriate times and the residual activity was measured at 0 °C using CO2 as substrate. The % residual activity was calculated with reference to the enzyme activity at 25 °C. All the reactions were performed in triplicate and in parallel with bovine CA.
Results
Preparation of TweCA
IPTG induction of Arctic Express (DE3) cells transformed with the plasmid pET15-b/TweCA resulted in the production of the recombinant TweCA. Growing the cells at 20 °C, most of the CA activity was recovered in the soluble fraction of the cell extract after sonication and centrifugation. Using the affinity column (His-select HF Nickel affinity gel), TweCA was purified to apparent homogeneity, as indicated by SDS-PAGE (). TweCA is a protein of 281 amino acid residues. A subunit molecular mass of 32.0 kDa was estimated by SDS-PAGE, while the molecular mass of 32.2 kDa was calculated on the basis of the amino acid sequence. The molecular weight determined by SDS-PAGE was in agreement with that obtained by HPLC size exclusion chromatography under native conditions to determine the oligomeric state of the protein. HPLC gel-filtration chromatography of TweCA gave an estimated molecular mass of 31 kDa (data not shown).
Primary structure and phylogenetic analysis
The amino acid sequence of TweCA was aligned with the polypeptide chain of the bovine α-CA, isoform II (). The two polypeptide sequences display 18.4% identity. Interestingly, as reported in , the metal coordination pattern in the α and δ-CAs are formed by three histidines as found in the γ-CAs, too. In the literature, the existence of five classes of CA (α, β, γ, δ and ζ) that appear to have evolved independently, has been described. Hence, we have constructed the most parsimonious phylogenetic tree in order to better investigate a possible phylogenetic relationships existing among these three classes of CAs (the α, γ and δ classes). The phylogenetic analysis was carried out using α-, γ- and δ-CAs from different prokaryotic and eukaryotic organisms (see ). From the dendrogram shown in , the α-CAs appear closely related to the δ-CAs, but clustered in a branch distinct from that of γ-CAs. These results probably indicate that there was one gene duplication event occurring in the ancestral lineage of CAs separating α- and δ-CA from γ-CA classes.
Figure 2. Alignment of the amino acid sequences of TweCA and bCAII performed using the program Bl2seq on two sequences. Numbering arrangement for TweCA is refereed to the δ system used by Lee et al. while for bCAII is referred to the human α-CA, isoform I. The metal coordination pattern in TweCA and bCAII has been indicated in gray and black bold, respectively. The asterisk (*) indicates identity at all aligned positions. The symbol (:) relates to conserved substitutions, while (.) means that semiconserved substitutions are observed. Organisms, accession numbers and cryptonyms of the sequences used in the alignment have been indicated in .
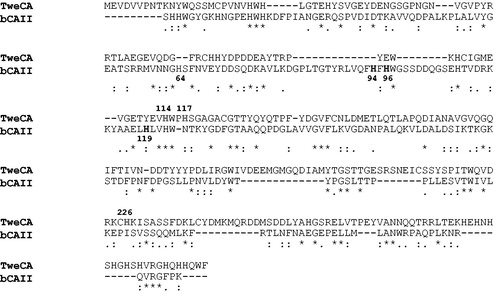
Figure 3. Phylogenetic tree of the α-, δ- and γ-CAs from selected prokaryotic and eukaryotic species. The tree was constructed using the program PhyML 3.0. Organisms, accession numbers and cryptonyms of the sequences used in the alignment have been indicated in .
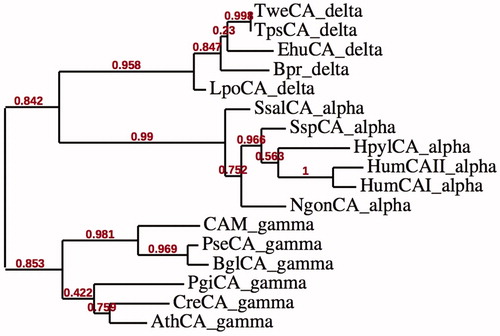
Table 1. CA class, organisms, accession numbers and cryptonyms of the sequences used in the phylogenetic analysis.
Enzyme kinetic
TweCA catalytic activity for the physiologic reaction, i.e. CO2 hydration to bicarbonate and protons, has been determined by stopped-flow technique. TweCA showed a significant catalytic activity with a kcat of 1.3 × 105 s−1 and a kcat/KM of 3.3 × 107 M−1 s−1.
Esterase activity
Since δ-CAs seem phylogenetically closer to α-CAs and, knowing that mammalian α-CAs have esterase activity, we have investigated the presence of esterase activity in TweCA. With pNpA as substrate, TweCA showed a specific activity of 487.5 U/mg of protein that is 5.4 times smaller than that of the commercially available bovine bCAII (). To verify that the hydrolysis of pNpA was intrinsic to the catalytic site of TweCA a control experiment with acetazolamide (AAZ) in the range 1–10 µM was performed. The obtained results were compared with that of bovine CA, isoform II. AAZ inhibits TweCA with a KIS of 83 nMCitation22. Interestingly, in the presence of all different concentrations of AAZ, the esterase activity was unchanged denoting that TweCA esterase activity is not intrinsic to the enzyme catalytic site () while the esterase activity of bCAII is sensitive to the presence of AAZ (). These results are in net contrast with those of Lee et al.Citation23 who recently reported that the δ-CA from T. weissflogii possesses esterase activity with this substrate, but no negative control with AAZ was performed by these authors. We can clearly state here that the δ-CAs do not possess esterase activity, similar to the β-, γ- and ζ-CAsCitation31.
Table 2. Esterase activity for TweCA and bCA II in absence and presence of AAZ (acetazolamide) used at different concentrations (1–10 µM).
Thermostability studies
We have investigated the effect of the temperature on the stability of TweCA and compared with that of the bovine α-CA (bCA II). The enzyme stability was studied in the 25–80 °C temperature range incubating the enzyme for 10, 30 and 60 min (). After incubation at the indicated times, enzyme activity was determined using CO2 as substrate as described by Capasso et al.Citation16. Interestingly, TweCA enzyme was stable up to 80 °C when the incubation time did not exceed 30 min. As shown in , the residual activity for 30 min and 80 °C is still 40%, and when the incubation time is 60 min the enzyme is completely inactivated at 80 °C. Otherwise, the bovine enzyme was inactivated at temperatures higher than 60 °C () for all incubation times. These studies indicate that the δ-CA from T. weissflogii was more stable at high temperatures and retained its activity for a longer time (e.g. 30 min) when compared to the mammalian enzyme. Probably, δ-CAs are characterized by a more compactness tridimensional structure than that of α-CAs.
Discussion
One third of the CO2 released in the atmosphere by anthropogenic activities is absorbed by the oceansCitation32, determining a dramatic change in seawater chemistry because CO2 reacts with water to form carbonic acid (H2CO3), which being unstable, dissociates into hydrogen ions (H+) and bicarbonate ions (HCO3−)Citation33. Diatoms are a key component of the biological carbon pump that exports carbon into the ocean, contributing significantly to the long-term sequestration of atmospheric CO2. Diatom genome sequences reveal the presence of different systems for HCO3− uptake most of which are phylogenetically affiliated with those found in metazoans. P. tricornutum containing a plastid-targeted β-CA suggest that the localization and role of the CAs in diatoms are main determinants of primary carbon metabolismCitation3. Considering this fact, with the help of the recombinant DNA technology, a δ-CA was identified in the genome of T. weissflogii and heterologously expressed in E. coli. The enzyme shared an identity of 18% with bCA II, a CA belonging to the α class. The alignment of the α and δ polypeptide chains allowed us to realize that these two CA-classes have in common the metal coordination pattern formed by three histidines, which is also present in the γ-CAs. Phylogenic analysis conducted on α-, γ- and δ-CAs separate these families into two clades, one formed by α and δ-CA sequences, and the other represented by γ-CAs. The observed pattern can be explained assuming the occurrence of an ancient CA duplication which took place before the separation of the α- and δ-CAs from γ-CAs. As described in a different paperCitation21, the β- and ζ-CAs are also related to each other but phylogenetically more distant from the α-, γ- and δ-CA clusters. Interestingly, the β- and ζ-CAs have a metal coordination pattern formed by two cysteines and one histidine, which is different from that of α-, γ- and δ-CAs.
A comparison of the biochemical properties of the δ-diatom and the α-mammalian enzyme showed: (a) a subunit molecular mass of 32.0 kDa for the δ-CA estimated by SDS-PAGE in agreement with that obtained by HPLC size exclusion chromatography under native conditions. (b) TweCA enzyme is an efficient catalyst for the CO2 hydration reaction with a kcat of 1.3 × 105 s−1 and a kcat/KM of 3.3 × 107 M−1 s−1, similar to that of the α-class, physiologically relevant human isoform hCA I.; (c) TweCA lacks esterase activity; (d) TweCA is more stable than the mammalian enzyme at high temperatures (>70 °C).
Our studies have given new insights on the biochemical properties of TweCA. Recently Lee et al.Citation23 observed that TweCA (named by these authors TWCA1) showed two distinct bands with masses of 35 and 70 kDA. The authors attributed the second band to the dimeric form of the enzyme. Our experiments, SDS-PAGE and HPLC size exclusion chromatography, have suggested that the purified TweCA is a monomer with a subunit mass of 32 kDA. Lee et al.Citation23, also reported that TweCA is catalytically active in the hydrolysis of pNpA. Here, we have investigated TweCA ability to catalyze the esterase reaction. Using the spectrophotometric assay we detected a specific activity 5.4 times lower that that of the α-CA, bCA II (). However, this esterase activity was persistent even when TweCA was incubated for 15 min with different concentrations of AAZ (1–10 µM) and then assayed spectrophotometrically for the pNpA hydrolysis (). On the contrary, the esterase activity of the mammalian bCA II was completely eliminated (i.e. inhibited) when the enzyme was treated with AAZ (). These experiments denote that TweCA lacks an active site capable to hydrolyze pNpA even if X-ray absorption spectroscopy at the Zn K-edge indicated that the active site of the marine diatom T. weissflogii CA is strikingly similar to that of mammalian α-CACitation34. The higher thermostability of the diatom enzyme respect to the mammalian enzyme might be attributed to a more compact structure of TweCA.
In conclusion, this paper demonstrates that the recombinant δ-CA (TweCA) is catalytically active for the hydration of CO2 but not for the hydrolysis of pNpA. Besides, the phylogenetic analysis showed that δ-CAs are filogenetically more correlated to the α-CAs than the other CAs classes. Future studies on this class of enzymes will provide new insights in understanding the role of δ-CA in the algal CCM used by many photosynthetic organisms because the δ-class is widely spread among marine phytoplankton.
Declaration of interest
The authors report no conflicts of interest. This study was financed in part by an FP7 EU project (Metoxia).
References
- Fernie AR, Obata T, Allen AE, et al. Leveraging metabolomics for functional investigations in sequenced marine diatoms. Trends Plant Sci 2012;17:395–403
- Giordano M, Beardall J, Raven JA. CO2 concentrating mechanisms in algae: mechanisms, environmental modulation, and evolution. Annu Rev Plant Biol 2005;56:99–131
- Bowler C, Vardi A, Allen AE. Oceanographic and biogeochemical insights from diatom genomes. Ann Rev Mar Sci 2010;2:333–65
- Depauw FA, Rogato A, Ribera d'Alcala M, Falciatore A. Exploring the molecular basis of responses to light in marine diatoms. J Exp Bot 2012;63:1575–91
- Ashida H, Saito Y, Kojima C, et al. A functional link between RuBisCO-like protein of Bacillus and photosynthetic RuBisCO. Science 2003;302:286–90
- Sultemeyer DF, Miller AG, Espie GS, et al. Active CO2 transport by the green alga Chlamydomonas reinhardtii. Plant Physiol 1989;89:1213–19
- Harada H, Nakatsuma D, Ishida M, Matsuda Y. Regulation of the expression of intracellular beta-carbonic anhydrase in response to CO2 and light in the marine diatom Phaeodactylum tricornutum. Plant Physiol 2005;139:1041–50
- Tanaka Y, Nakatsuma D, Harada H, et al. Localization of soluble beta-carbonic anhydrase in the marine diatom Phaeodactylum tricornutum. Sorting to the chloroplast and cluster formation on the girdle lamellae. Plant Physiol 2005;138:207–17
- Johnston AM, Raven JA, Beardall J, Leegood RC. Carbon fixation. Photosynthesis in a marine diatom. Nature 2001;412:40–1
- Roberts K, Granum E, Leegood RC, Raven JA. C3 and C4 pathways of photosynthetic carbon assimilation in marine diatoms are under genetic, not environmental, control. Plant Physiol 2007;145:230–5
- Alterio V, Langella E, Viparelli F, et al. Structural and inhibition insights into carbonic anhydrase CDCA1 from the marine diatom Thalassiosira weissflogii. Biochimie 2012;94:1232–41
- De Simone G, Supuran CT. (In)organic anions as carbonic anhydrase inhibitors. J Inorg Biochem 2012;111:117–29
- Supuran CT. Carbonic anhydrases as drug targets – an overview. Curr Top Med Chem 2007;7:825–33
- Supuran CT. Carbonic anhydrases – an overview. Curr Pharm Des 2008;14:603–14
- Supuran CT, Scozzafava A. Carbonic anhydrases as targets for medicinal chemistry. Bioorg Med Chem 2007;15:4336–50
- Capasso C, De Luca V, Carginale V et al. Biochemical properties of a novel and highly thermostable bacterial alpha-carbonic anhydrase from Sulfurihydrogenibium yellowstonense YO3AOP1. J Enzyme Inhib Med Chem 2012;27:892–7
- Capasso C, Supuran CT. Sulfa and trimethoprim-like drugs – antimetabolites acting as carbonic anhydrase, dihydropteroate synthase and dihydrofolate reductase inhibitors. J Enzyme Inhib Med Chem 2013 . [Epub ahead of print]. doi: 10.3109/14756366.2013.787422
- Capasso C, Supuran CT. Anti-infective carbonic anhydrase inhibitors: a patent and literature review. Expert Opin Ther Pat 2013;23:693–704
- Del Prete S, Isik S, Vullo D, et al. DNA cloning, characterization, and inhibition studies of an alpha-carbonic anhydrase from the pathogenic bacterium Vibrio cholerae. J Med Chem 2012;55:10742–8
- Del Prete S, Vullo D, De Luca V, et al. A highly catalytically active gamma-carbonic anhydrase from the pathogenic anaerobe Porphyromonas gingivalis and its inhibition profile with anions and small molecules. Bioorg Med Chem Lett 2013;23:4067–71
- Del Prete S, Vullo D, Scozzafava A, et al. Cloning, characterization and anion inhibition study of the δ-class carbonic anhydrase (TweCA) from the marine diatom Thalassiosira weissflogii. Bioorg Med Chem. 2013 . [Epub ahead of print]. doi: 10.1016/j.bmc.2013.10.045
- Vullo D, Del Prete S, Osman SM, et al. Sulfonamide inhibition studies of the δ-carbonic anhydrase from the diatom Thalassiosira weissflogii. Bioorg Med Chem Lett. 2013 . [Epub ahead of print]. doi: 10.1016/j.bmcl.2013.11.021
- Lee RBY, Smith JAC, Rickaby REM. Cloning, expression and characterization of the δ-carbonic anhydrase of Thalassiosira weissflogii (Bacillariophyceae). J Phycol 2013;49:170–7
- Cantalloube H, Labesse G, Chomilier J, et al. Automat and BLAST: comparison of two protein sequence similarity search programs. Comput Appl Biosci 1995;11:261–72
- Guindon S, Gascuel O. A simple, fast, and accurate algorithm to estimate large phylogenies by maximum likelihood. Syst Biol 2003;52:696–704
- Laemmli UK, Favre M. Maturation of the head of bacteriophage T4. I. DNA packaging events. J Mol Biol 1973;80:575–99
- Khalifah RG. The carbon dioxide hydration activity of carbonic anhydrase. I. Stop-flow kinetic studies on the native human isoenzymes B and C. J Biol Chem 1971;246:2561–73
- Carta F, Temperini C, Innocenti A, et al. Polyamines inhibit carbonic anhydrases by anchoring to the zinc-coordinated water molecule. J Med Chem 2010;53:5511–22
- Chirica LC, Elleby B, Jonsson BH, Lindskog S. The complete sequence, expression in Escherichia coli, purification and some properties of carbonic anhydrase from Neisseria gonorrhoeae. Eur J Biochem 1997;244:755–60
- Armstrong JM, Myers DV, Verpoorte JA, Edsall JT. Purification and properties of human erythrocyte carbonic anhydrases. J Biol Chem 1966;241:5137–49
- Innocenti A, Supuran CT. Paraoxon, 4-nitrophenyl phosphate and acetate are substrates of alpha- but not of beta-, gamma- and zeta-carbonic anhydrases. Bioorg Med Chem Lett 2010;20:6208–12
- Zhao D, Wu S, Yin Y. Responses of terrestrial ecosystems' net primary productivity to future regional climate change in China. PLoS One 2013;8:e60849
- Feely RA, Sabine CL, Lee K, et al. Impact of anthropogenic CO2 on the CaCO3 system in the oceans. Science 2004;305:362–6
- Cox EH, McLendon GL, Morel FM, et al. The active site structure of Thalassiosira weissflogii carbonic anhydrase 1. Biochemistry 2000;39:12128–30