Abstract
With each heartbeat, billions of cardiomyocytes work in concert to propagate the electrical excitation needed to effectively circulate blood. Regulated expression and timely delivery of connexin proteins to form gap junctions at the specialized cell–cell contact region, known as the intercalated disc, is essential to ventricular cardiomyocyte coupling. We focus this review on several regulatory mechanisms that have been recently found to govern the lifecycle of connexin 43 (Cx43), the short-lived and most abundantly expressed connexin in cardiac ventricular muscle. The Cx43 lifecycle begins with gene expression, followed by oligomerization into hexameric channels, and then cytoskeletal-based transport toward the disc region. Once delivered, hemichannels interact with resident disc proteins and are organized to effect intercellular coupling. We highlight recent studies exploring regulation of Cx43 localization to the intercalated disc, with emphasis on alternatively translated Cx43 isoforms and cytoskeletal transport machinery that together regulate Cx43 gap junction coupling between cardiomyocytes.
INTRODUCTION
In order to participate as functional units within an organized tissue system, individual cells utilize multi-functional adhesion proteins for structural support, cytoskeletal organization, subcellular protein localization, and precise coordination of external signals. The heart is an organ in which individual cardiomyocytes must adhere and communicate with neighboring cells along longitudinally oriented fibers in order to rapidly transmit electrical impulses and drive synchronized beat-to-beat muscle contraction. Cardiomyocytes achieve rapid coupling through gap junctions (GJs), which are associated with adhesion junction complexes at the intercalated disc (ID). GJs comprise connexin (Cx) channels formed by the pairing of abutting hexameric hemichannels (connexons) from adjacent cells (CitationUnwin & Zampighi, 1980), and provide electrical, metabolic, and immunological connectivity between cells, generally facilitating the passage of molecules less than 1 kDa in size (CitationSmyth & Shaw, 2012). Overall intercellular conductance is determined by individual GJ conductance, as well as by membrane GJ density. Intercellular conductance in the heart remains stable despite the reality that the Cx protein has a half-life of only 1–5 hours (CitationBeardslee et al., 1998; CitationShaw et al., 2007). Cxs are constantly made, transported, and degraded in living cells, and these processes are rapidly and exquisitely regulated by intracellular as well as extracellular cues.
A total of 20 and 21 Cxs have been identified in the sequenced mouse and human genomes, respectively (CitationSohl & Willecke, 2003, Citation2004). Throughout the heart, GJs are made up of specific combinations of the following Cxs: Cx40, Cx43, Cx45, and Cx30.2, of which Cx43 is the most abundantly expressed in ventricular muscle (CitationZhang & Shaw, 2013; CitationBoukens & Christoffels, 2012; CitationOyamada et al., 2013). In individual ventricular cardiomyocytes, Cx43 is localized in large aggregates at longitudinal ends of the cell, along with cadherins, desmosomes, and other ion channels (CitationDelmar, 2004; CitationSevers, 1989; CitationForbes & Sperelakis, 1985; CitationPalatinus et al., 2012). This precisely arranged Cx43 cell surface localization facilitates rapid and directional action potential propagation. Critical to ventricular myocyte coupling is the tight regulation of the Cx43 lifecycle beginning with gene expression and followed by i) ER to Golgi assembly and transport, ii) forward trafficking of de novo connexons from the Golgi to the cell surface, iii) maintenance and organization within the GJ plaque region, and (iv) retrograde transport for degradation (CitationD'hondt et al., 2013; CitationMarquez-Rosado et al., 2012) (CitationSmyth & Shaw, 2012; CitationZhang & Shaw, 2013; CitationHesketh et al., 2009; CitationThevenin et al., 2013; CitationJohnstone et al., 2012). Post-translational modification occurring at the Cx43 C-terminus is key in regulating many of these steps including channel assembly, trafficking, channel gating, and degradation.
Cx43 function is indispensable for normal development and maintenance of coordinated impulse propagation in the heart (CitationReaume et al., 1995; CitationHuang et al., 1998; CitationYa et al., 1998; CitationGuerrero et al., 1997; CitationThomas et al., 1998; CitationEloff et al., 2001; Citationvan Rijen et al., 2004; CitationMorley et al., 1999; CitationEckardt et al., 2004; CitationDanik et al., 2004; CitationGutstein et al., 2001; CitationShaw & Rudy, 1997). Mutations in Gja1, which encodes Cx43, are associated with atrial fibrillation, heterogeneous GJ loss, and sudden infant death (CitationThibodeau et al., 2010; CitationVan Norstrand et al., 2012). Abnormal Cx43 post-translational modifications and trafficking defects can also result in lethal arrhythmias (CitationKalcheva et al., 2007; CitationPeters et al., 1997; CitationRemo et al., 2011; CitationBeardslee et al., 2000; CitationAi & Pogwizd, 2005). In the setting of heart failure (HF), which is a growing epidemic in the United States (CitationGo et al., 2013), disease progression is characterized by extensive tissue remodeling and altered expression of cardiac ion channels (CitationSmith et al., 1991; CitationPeters et al., 1993; Hong et al., Citation2012a, Citation2012b; CitationSmyth et al., 2010). Cx43 is not only downregulated in HF patients; less Cx43 protein is localized to the ID to form GJ plaques. Common to these cardiomyopathies is aberrant Cx43 localization away from the ID.
In this review, we will discuss recent findings on the key checkpoints of the Cx43 lifecycle as summarized in . We focus our discussion on transcriptional and translational control of Cx43 expression, ER to Golgi transport, cytoskeletal regulation of Cx43 forward trafficking, and GJ organization at the dynamic ID. Given the fast Cx43 turnover rate (CitationBeardslee et al., 1998; CitationJordan et al., 1999; CitationShaw et al., 2007), regulatory cues governing the Cx43 forward trafficking step play an important role in the regulation of intercellular coupling. Understanding this regulation is critical for future interventions that aim to rescue heart function by bolstering the delivery efficiency of Cx43 cargo. Together, the regulatory mechanisms presented will serve as a solid foundation upon which new clinical treatments can be developed.
Figure 1. The Cx43 lifecycle. Key steps of the lifecycle are highlighted: transcriptional control of Cx43 expression pattern and level, mTOR-regulated alternative internal translation of Gja1 mRNA, the role of smaller N-terminally truncated Cx43 isoforms in ER-to-Golgi transport, and directed targeting to the ID via the microtubule and actin cytoskeleton.
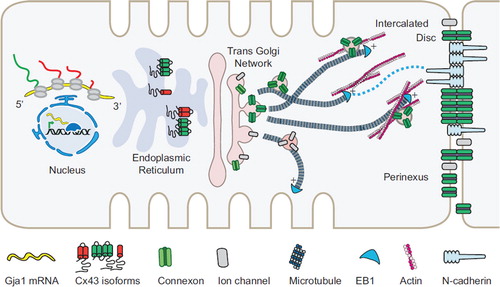
MULTILAYERED REGULATION OF CX43 BIOGENESIS
Patterning of intercellular communication via transcriptional control
Changes in Cx43 expression level and region-specific patterning in the heart can alter cell–cell coupling and the special spread of excitation. Gene expression is an early primary step in the generation of functional GJ channels, and includes transcription, RNA splicing and processing, and translation. We begin our discussion on recent studies on the control of Cx43 expression.
Cx43 resides on mouse chromosome 10 and human chromosome 6, and has a relatively simple genomic structure of just two exons (CitationFishman et al., 1991; CitationSullivan et al., 1993). The first exon encodes most of the 5′-untranslated region (5′-UTR), while the entire coding sequence plus the 3′-UTR reside within the second exon. Two alternative promoters, along with four additional non-coding exons comprising alternative 5′-UTRs, have been reported (CitationPfeifer et al., 2004). While the canonical promoter is active throughout the heart, an alternative promoter is only active in the atria and septum, but not in the ventricle. An additional intronic promoter is utilized in the ventricles only. Based on promoter choice, a total of nine Gja1 transcripts can be generated that only differ in their 5′-UTR to affect translational efficiencies of the corresponding mRNAs.
Several T-box and Iroquois homeobox transcription factors, which regulate cardiac development, have been shown to pattern areas of ion channel expression throughout the heart and parts of the conduction system (CitationBoukens & Christoffels, 2012; CitationZhang et al., 2011; CitationGaborit et al., 2012; CitationBakker et al., 2008; CitationOyamada et al., 2013; CitationWiese et al., 2009). A central component of the cardiac conduction system is the sinoatrial node (SAN), which functions as the pacemaker to set the heartbeat. The atrioventricular node (AVN) then delays the traveling electrical impulse to ensure atrial ejection of blood before ventricular contraction, and to protect the ventricles from atrial arrhythmias. The ventricular conduction system, comprising the His bundle, bundle branches, and the His-Purkinje network, functions to rapidly direct the depolarizing impulse from the AVN to synchronously activate ventricular contraction.
Of the T-box transcription factor family, Tbx3 and Tbx18 can directly bind to the canonical promoter of Cx43 to pattern its region-specific expression. Tbx3 is required in developmental patterning and controls the pacemaker gene program in vivo, including repression of Cx43 transcription (Hoogaars et al., Citation2007a, Citation2007b). Tbx3 is also sufficient to downregulate Cx43, along with Cx40 and Nav1.4, in the reprogramming of mature cardiomyocytes to form SAN-like pacemaker cells (CitationBakker et al., 2012). Tbx18 acts upstream of Tbx3 and is required for specification of the SAN (CitationWiese et al., 2009). Tbx18 directly represses Cx43 transcription and cell–cell coupling in postnatal cardiomyocytes when overexpressed, and is sufficient for the reprogramming of mature cardiomyocytes to form SAN-like cells in a guinea-pig model (CitationKapoor et al., 2011, Citation2013). Common to both peacemaker cell conversion approaches is the need to suppress GJ coupling and downregulate resident channels of the ID that normally function together to drive depolarization and rapid directional impulse propagation across aligned ventricular cardiomyocytes.
Homeobox factors, which are characterized by a 60 amino acid DNA-binding domain known as the homeodomain, function within a transcriptional complex with other co-factors to switch gene cascades on or off in various tissues (CitationOyamada et al., 2013; Scott et al., Citation1989). Within this gene family, Nkx2.5 and Iroquois can function as either activators or repressors of gene transcription, depending on the cellular context. Nxk2.5 has highly evolutionarily conserved roles in heart development and function, and acts as a transcriptional repressor of Cx43 (CitationKasahara et al., 2001, Citation2003; CitationDupays et al., 2005; CitationTanaka et al., 1999; CitationBenson et al., 1999; CitationLyons et al., 1995; CitationSchott et al., 1998; CitationGoldmuntz et al., 2001). The Iroquois homeobox gene 3 (Irx3) contains a homeodomain belonging to the three amino acid loop extension (TALE) superclass (CitationGomez-Skarmeta & Modolell, 2002), and has been shown to regulate Cx expression in the ventricular conduction system to tightly control electrical excitation spread for ventricular activation (CitationZhang et al., 2011).
The ventricular conduction system is extensively coupled via GJs containing specific combinations and region-specific patterns of mostly Cx40 and Cx43 (CitationOyamada et al., 2013; CitationBoukens & Christoffels, 2012; Citationvan Veen et al., 2001). Irx3 antithetically regulates Cx40 and Cx43 whereby Irx3 indirectly activates Cx40 expression but directly represses Cx43 transcription. Irx3 loss-of-function resulted in reduced Cx40 throughout the developing ventricular conduction system, and ectopic Cx43 expression in the proximal bundle branches. These changes were accompanied by abnormal cell–cell coupling, disruption of rapid spread of ventricular excitation, as well as right bundle branch block. Taken together, Nkx2.5 and Irx3 function in the tight regulation of Cx expression, and represent a transcriptional regulatory pathway of cell–cell coupling that can contribute to arrhythmogenesis when disrupted.
Found in translation: the secret life of Gja1 mRNA
We continue our Cx43 biogenesis discussion on how such a rich Cx43 protein repertoire is generated from a two-exon encoded gene (), focusing specifically on the recent finding that multiple Cx43 isoforms are made via alternative translation (CitationSmyth & Shaw, 2013). Given its relatively simple gene structure, yet unique functions at various cellular locations, Smyth and Shaw asked whether additional mechanisms exist to explain the vast functional diversity.
An initial clue came from the finding that cap-independent translation occurs at internal ribosome entry sites (IRES) in eukaryotic systems (CitationCandeias et al., 2006; CitationIngolia et al., 2011). In addition to the previously known IRES in the Gja1 5’UTR (CitationSchiavi et al., 1999), we identified that the Gja1 mRNA is polycistronic, encoding at least four smaller Cx43 isoforms via alternative start site (AUG codon) selection within the coding mRNA. This alternative translation step is negatively regulated by PI3K/AKT/mTOR signaling, which promotes cap- dependent translation. While transcriptional control determines how much full-length Cx43 is made, this novel regulatory step during translation fine-tunes the precise type of Cx43 isoform that is generated in response to signaling and cellular need. As discussed below, smaller Cx43 isoforms facilitate anterograde transport of full-length Cx43 to autoregulate the degree of GJ coupling at cell–cell borders.
TRAFFICKING HIGHWAYS TO THE ID
After biogenesis, the Cx43 lifecycle continues with ER to Golgi transport, vesicular trafficking from the trans-Golgi network (TGN) to the cell surface, organization, and maintenance within the GJ plaque, and retrograde transport for degradation (CitationD'hondt et al., 2013; CitationSmyth & Shaw, 2012; CitationThevenin et al., 2013). Changes in regulation of the balance of these steps can quickly affect the amount of Cx43 available for coupling at cell–cell junctions. In this section, we focus on recent developments on targeted delivery of Cx43 to the ID, and discuss how Cx43 GJs are organized once they reach their destination. Cx43 GJ internalization, which depends on post-translational modifications of the Cx43 C-terminal tail, is also a highly regulated process that controls surface GJ availability. Interested readers, please refer to these studies (CitationSu et al., 2010; CitationFong et al., 2013; CitationCochrane et al., 2013; CitationThevenin et al., 2013; CitationFong et al., 2012; CitationJohnstone et al., 2012; Falk et al., Citation2009) for developments on this front.
Keeping up with the connexons—novel isoforms function as chaperones
Prior to forward transport along the cytoskeleton, Cx43 traverses through the ER and Golgi apparatus to oligomerize and acquire the appropriate posttranslational modifications (CitationMusil & Goodenough, 1993; CitationSmith et al., 2012). Unlike other channels such as the L-type calcium channel that utilize channel-specific auxiliary subunits for transport and function (CitationZhang & Shaw, 2013; CitationGerhardstein et al., 2000; CitationGao et al., 2001; CitationHulme et al., 2005; CitationHulme et al., 2006; CitationDomes et al., 2011; CitationFu et al., 2011), no such subunits have been identified for Cx43. Smyth and Shaw discovered alternative internal translational events as the mechanism generating N-terminally truncated Cx43 isoforms (Smith & Shaw, 2013). To understand the functional significance of the smaller isoforms, mutations of four internal AUG start sites were made to reveal Cx43 accumulation in the ER, and a marked decrease of Cx43 at the cell surface. This change in localization was rescued by overexpressing a 20-kilodalton isoform (GJA1-20K), which is the most abundantly expressed smaller isoform. When full-length Cx43 and GJA1-20K were differentially tagged with fluorescent reporters and imaged, they were found to be associated in reticulated ER structures, but only full-length Cx43 was found on the cell surface. Together, these findings indicate that the smaller isoforms can function as chaperones for Cx43 transport through the ER/Golgi, prior to vesicular trafficking along the cytoskeleton.
Cx43 isoform generation, affecting GJ-based intercellular conductance, was also found to be under mTOR signaling regulation. Inhibition of PI3K/AKT/mTOR, which normally promotes cap-dependent translation and thus full-length Cx43 levels (CitationFolkes et al., 2008; CitationFeldman et al., 2009), increased GJA1-20K chaperone expression and yielded larger GJ plaques at neonatal ventricular cardiomyocyte cell–cell borders. Many GJ-independent roles have been attributed to the Cx43 C-terminal tail (CitationPalatinus et al., 2012; CitationFrancis et al., 2011; CitationRhee et al., 2009; CitationXu et al., 2006). Involvement of mTOR indicates that mTOR inhibitors, which are common therapeutic immunosuppressants used in organ transplantation, may possibly be used to restore cell–cell coupling in failing hearts as well.
Our findings also offer a potential mechanism to explain how Cx43 plays vastly different roles independent of cell–cell communication, such as trafficking of Nav1.5 (CitationRhett et al., 2012), mitochondrial function (CitationRodriguez-Sinovas et al., 2006), and cell cycle regulation (CitationOlbina & Eckhart, 2003). It is possible that some of these functions could be carried out by alternatively translated isoforms acting at different intracellular organelles. We speculate that alternative translation could also contribute to some of the cardiac phenotypes of Cx43 C-terminal truncation mouse models that either retain, or completely lack, a wild type copy of full-length Cx43 (CitationMaass et al., 2007; CitationMaass et al., 2009).
Leaving the TGN: directed targeting along microtubules
Once the correct Cx type, isoform, and amounts are made, what regulatory cues direct final delivery of the precious cargo to form Cx43 GJs? In the secretory transport pathway, vesicles emerge from the Golgi apparatus and are transported to the cell–cell junction along the filamentous microtubule and actin networks (CitationRoss et al., 2008). This dynamic process is dependent upon coordinated action of microtubule-based motors, kinesin, cytoplasmic dynein/dynactin, as well as actin-based myosin motors. Cx43 is a short-lived protein with a half-life of only 1–5 h (CitationBeardslee et al., 1998; CitationJordan et al., 1999; CitationShaw et al., 2007), suggesting its intracellular movements are tightly regulated. How cytoskeletal transport machinery contributes to Cx43 hemichannel trafficking specificity in cardiomyocytes is an active area of investigation.
A critical aspect of Cx43 forward transport in the heart is microtubule-based localization to the ID of ventricular cardiomyocytes (CitationShaw et al., 2007; CitationSmyth et al., 2010). The ID mediates mechanical as well as electrical coupling between cardiomyocytes, and is enriched with the Ca2+-dependent adhesion molecule N-cadherin (CitationDelmar, 2004; CitationSevers, 1989; CitationPalatinus & Gourdie, 2007; CitationForbes & Sperelakis, 1985). A number of cardiomyopathies are characterized by disrupted mechanical junctions associated with altered Cx43 localization and dissociation from N-cadherin and desmosomal proteins (CitationHesketh et al., 2010; CitationAkar et al., 2004; CitationKostin et al., 2004; CitationSepp et al., 1996; CitationFidler et al., 2009; CitationOxford et al., 2007). Thus, the ability of cardiomyocytes to form adhesion contacts is critical for Cx43 GJ localization to the ID. How the microtubule-based trafficking machinery contributes to polarized localization of Cx43 GJs in ventricular cardiomyocytes is discussed below.
Data exist for multiple, but not mutually exclusive, models of Cx43 localization to the ID (CitationGaietta et al., 2002; CitationLauf et al., 2002; CitationShaw et al., 2007; CitationSmyth & Shaw, 2012; CitationZhang & Shaw, 2013; CitationJohnson et al., 2002). It has been established that microtubules participate in the delivery of Cx43 to the plasma membrane. Initial landmark studies provided evidence that de novo Cx43 appears at the perimeter of the GJs before diffusing into the plaque center (CitationJordan et al., 1999; CitationLauf et al., 2002). Based on these findings, it was proposed that Cx43 hemichannels are inserted into the general plasma membrane and quickly diffuse to the edge of GJ plaque, before slowly diffusing into the plaque center. Subsequently, it was observed that Cx43 can be inserted directly at the plaque and that membrane fluidity exists within the GJ space (Falk et al., Citation2009; CitationShaw et al., 2007; CitationMajoul et al., 2013). Plaques can also be internalized from regions away from the plaque center, and that full GJ plaque internalization can occur in a single step (Falk et al., Citation2009; CitationPiehl et al., 2007). Recent studies identified that collections of Cx43 hemichannels, which exist in a “perinexus” space around the GJ plaque, interact with scaffolding proteins as well as other ion channels (CitationRhett et al., 2011; CitationRhett et al., 2012). These studies provide evidence that the GJ plaque and the surrounding membrane domain are highly dynamic, exhibiting complex behavior of targeted insertion and internalization events to organize communication between cells.
Given the low density of Cx43 hemichannels in membrane regions well away from the GJ plaque, and the technical difficulty of distinguishing Cx43 already inserted into the membrane from submembranous collections in the cytoplasm, the lateral diffusion coefficient of membrane-bound Cx43 has been difficult to quantify. It is possible that hemichannels could diffuse within the plasma membrane before arriving at the GJ plaque. Our directed targeting paradigm is based on the observation that de novo Cx43 hemichannels are targeted directly to adhesion junctions with specificity directed by the Cx43 hemichannel, microtubule plus-end tracking proteins EB1 and p150(Glued), and the adherens junction structure including β-catenin. In the directed targeting model, dynamic microtubules act as highways that terminate and anchor at adherens junction complexes to allow direct delivery of Cx43 cargo (CitationShaw et al., 2007; CitationSmyth et al., 2010, Citation2012). Based on these studies, instead of long-range lateral diffusion, local hemichannel movement within the plaque and the perinexus is likely to occur to organize GJs and modulate coupling conductance.
In line with the short Cx43 half-life and constant need to maintain cellular coupling in the heart, the dynamic model of directed forward trafficking offers an efficient way to control GJ coupling in response to changes in the cardiomyocyte microenvironment. A similar cytoskeleton-based directed-targeting paradigm is conserved for Cav1.2 delivery to the cardiac T-tubule, which is a specialized membrane subdomain facilitating calcium influx deep within the cardiomyocyte to drive excitation–contraction coupling (Hong et al., Citation2012b). In particular, the BIN1 scaffolding protein was shown to anchor microtubules laden with Cav1.2 channels to T-tubules, similar to the role of N-cadherin in anchoring EB1/p150(Glued)-tipped microtubules at the ID. Further work supporting this model revealed that Cx43 and Cav1.2 were decreased at their respective membrane subdomains, along with displacement of their associated trafficking machinery, in the setting of heart disease (Hong et al., Citation2012a, Citation2012b; CitationSmyth et al., 2010). We speculate that this trafficking paradigm can be generalizable to other cardiac ion channels, and be explored in context of other cytoskeletal elements and anchor proteins.
Actin’ on cargo trafficking specificity
In addition to microtubule-based trafficking, the actin cytoskeleton is implicated in hemichannel delivery to the cell–cell junction. In cardiomyocytes, α actin comprises the thin filaments of the sarcomere (CitationBoateng and Goldspink, 2008), while β and γ actin form filamentous actin (F-actin) not associated with generating contractile force (CitationHayakawa et al., 1996). In line with an organizational role, formation and maintenance of membrane subdomains such as the ID, caveolae, and T-tubules depend upon F-actin (CitationNoorman et al., 2009; CitationItoh et al., 2005). However, actin is also highly dynamic, known to regulate intracellular vesicular transport through motor protein-based trafficking and vesicular fusion with the cell membrane (CitationJaiswal et al., 2009; CitationRogers & Gelfand, 2000), as well as mediate long-range vesicular trafficking in plants and mouse oocytes (CitationSchuh, 2011; CitationAkkerman et al., 2011). Moreover, dye transfer studies identified the dependence of GJ formation and maintenance on actin (CitationTheiss and Meller, 2002; CitationQu et al., 2009; CitationThomas et al., 2001).
Building on these roles, we set out to test whether F-actin directly participates in the regulation of Cx43 trafficking to form GJs between cardiomyocytes (CitationSmyth et al., 2012). We found that Cx43 co-immunoprecipitates with β actin, and colocalizes with non-sarcomeric actin structures along the vesicular transport pathway at the perinuclear region, as well as ID regions. Using live-cell imaging, we determined the time course and directionality of Cx43 cargo movement relative to actin. Cx43 cargo slowed down when associated with actin, with the majority traveling at speeds slower than that of microtubule-based transport (> 0.25 μm/s) (CitationFort et al., 2011; CitationShaw et al., 2007), but consistent with myosin-based transport on actin (CitationHoward, 1997). This finding is consistent with previous studies of actin–myosin based transport of melanosomes, slowing of endocytic vesicles at actin-rich regions in the cell cortex (CitationAschenbrenner et al., 2004; CitationRoss et al., 2008), and Cx32 pausing at actin structures en route to the hepatocyte cell surface (CitationFort et al., 2011).
By monitoring timed release of Cx43 accumulated in the ER (CitationMisumi et al., 1986), we identified F-actin integrity as necessary for de novo Cx43 delivery to cell–cell borders in neonatal ventricular cardiomyocytes. Cx43 plaque formation at N-cadherin-containing IDs was examined in Langedorff-perfused mouse hearts subjected to acute no-flow ischemia, actin disruption by latrunculin A, or both treatments. Cx43 localization at the cell–cell junction was comparably reduced with either treatment, but the effect was not additive with both treatments, suggesting a common pathway. In control hearts, Cx43 and β actin interact biochemically and this was disrupted when actin polymerization was inhibited, or when the heart became ischemic. Together, these studies reveal that F-actin is associated with a slow-moving Cx43 cargo population, and that this interaction is an essential component of the Cx43 forward trafficking machinery.
Based on the growing evidence supporting directed delivery, the microtubules that load Cx43 at the Golgi apparatus may not end up delivering the cargo to the cellular junction. This flexibility in transport provides cardiomyocytes with a means to respond quickly to the microenvironment such that Cx43 deposited along F-actin can be readily recruited by dynamic EB1-tipped microtubules. Our studies revealed noncontractile F-actin as a cytoplasmic Cx43 reservoir, serving as an alternate trafficking route and a means to mass acute Cx43 delivery in response to cellular stress.
Future work is needed to test how the F-actin network determines cargo directionality toward the cellular junction, and identify additional cytoskeletal players involved in this dynamic process. Interestingly, secretory vesicles in plant cells are transported by an actin–myosin system involving two types of F-actin. Vesicles exhibit faster and directional motility along long F-actin tracks, but move slowly with little directionality in areas containing a fine F-actin meshwork (CitationAkkerman et al., 2011). Could separate types of cytosolic actin structures exist in the cardiomyocyte cytoplasm to affect Cx43 trafficking? How is Cx43 trafficking specificity controlled by actin? Does interplay exist between the actin and microtubule machineries in directing Cx43 delivery to the cell–cell junction? These are intriguing questions that remain to be answered.
Organization and maintenance within the dynamic ID
Once delivered to the ID, GJ coupling conductance is dependent upon the stability and organization of resident proteins. The original view, first articulated in the late 1980s, held that the ID contains adhesion molecules (desmosomes and adherens junctions), and GJ channels that exist independently from each other (CitationUnwin & Zampighi, 1980; CitationForbes & Sperelakis, 1985; CitationSevers, 1989). Recent data found that the ID is a highly complex and dynamic membrane space, which is continuously organized by scaffolding proteins linking the cytoskeleton to resident transmembrane proteins.
During development, polarization of mechanical junctions at end-to-end contacts was observed before that of Cx43, which is initially widely distributed throughout the sarcolemma, including the lateral sides of the cell (CitationMuhlfeld & Richter, 2006; CitationMaass et al., 2007; CitationFromaget et al., 1992; CitationGourdie et al., 1991, Citation1992; CitationPeters et al., 1994). Preferential Cx43 localization to IDs over developmental time was proposed to be a consequence of slower Cx43 internalization rates at IDs in comparison with other membrane areas, which are sparsely decorated with adhesion junctions and are thus less stable (CitationHirschy et al., 2006; CitationAngst et al., 1997; CitationPalatinus et al., 2012). Moreover, a close link between Cx43 localization and mechanical adhesion was demonstrated in experimental and clinical cardiomyopathy studies, which revealed Cx43 dissociation from N-cadherin and desmosomes, as well as membrane lateralization (CitationAkar et al., 2004; CitationSmyth et al., 2010; CitationAkar et al., 2007; CitationHesketh et al., 2010; CitationKostin et al., 2004; CitationNygren et al., 2007; CitationSepp et al., 1996; CitationUzzaman et al., 2000; CitationLi et al., 2008). These studies demonstrate a hierarchical dependence of Cx43 on mechanical junctions and that cardiomyocytes form polarized physical adhesions with neighboring cells before organizing communication via Cx43 GJs.
The GJ plaque, and the surrounding perinexus space, are increasingly appreciated as a dynamic structure in which resident proteins form composite structures that are constantly delivered, organized, and turned over (CitationMajoul et al., 2013; CitationAgullo-Pascual et al., 2013; CitationPalatinus et al., 2012; CitationRhett et al., 2013). The perinexus is envisaged as a sieve-like network containing Cx43 hemichannels interacting with the tight junction protein, zonula occludens protein 1 (ZO-1), via the postsynaptic density/disc large/ZO-1 (PDZ) domain in the Cx43 C-terminal tail (CitationGiepmans et al., 2001; CitationToyofuku et al., 1998). Interactions between Cx43 and ZO-1 were initially implicated in Cx43 GJ endocytosis under specific settings (CitationBarker et al., 2001, Citation2002, Citation2008; Citationvan Zeijl et al., 2007; CitationPiehl et al., 2007; CitationDuffy et al., 2004). Moreover, given the ability of ZO-1 to link GJs, adherens junctions and the actin cytoskeleton, it was proposed that the ZO-1/Cx43 interaction held an additional role in GJ aggregation. Supporting evidence revealed preferential localization of ZO-1 at the edge of the Cx43 GJ plaque, and an increase in GJ size with the loss of ZO-1/Cx43 interactions (CitationPalatinus et al., 2011; CitationHunter et al., 2005; CitationRhett et al., 2011; CitationMaass et al., 2007). The ZO-1/Cx43 interaction is now understood to have multiple roles including restricting the accrual of new connexons into the GJ plaque.
A mouse model expressing a Cx43 C-terminal truncation mutant at amino acid 258, lacking the PDZ domain to interact with ZO-1, exhibited no disruption of GJ formation at the ID (CitationMaass et al., 2007). However, GJ plaques appeared bigger and accumulated at the periphery of the ID, in line with a role for ZO-1 in sequestering Cx43 in the perinexus space. Adding to the complexity of the dynamic ID disc, deletion of the last five amino acids (encoding one of two PDZ domains) of the Cx43 C-terminus in another mouse model did not disrupt ZO-1 binding, localization at the ID, or GJ channel function. Instead, these last five residues were required for maintaining proper functional properties of the sodium and potassium channels (CitationLubkemeier et al., 2013).
The concept that the ID is a hub for cell adhesion, electrical coupling, as well as cellular excitability, is rapidly emerging. The pore-forming alpha subunit of the sodium channel encoded by Nav1.5, along with the scaffolding proteins plakophilin-2 (Pkp2) and Ankryin-G (AnkG), have been detected at the ID space to interacting with Cx43 (CitationKucera et al., 2002; CitationLowe et al., 2008; CitationPetitprez et al., 2011; CitationMalhotra et al., 2004; CitationRhett et al., 2013; CitationSato et al., 2011). Further support comes from a super-resolution florescence microcopy study that revealed colocalization of Cx43, Pkp2, and AnkG inside the GJ plaque (CitationAgullo-Pascual et al., 2013). This study added another layer of complexity to the ID space, by implicating AnkG–actin interactions as a means to further restrict the flow of Cx43 hemichannels within the perinexus.
Taken together, these studies offer distinct, but not mutually exclusive, mechanisms for polarized Cx43 GJ localization in cardiomyocytes. Dynamic cytoskeletal-based Cx43 trafficking directly to adherens junctions accounts for rapid Cx43 delivery in response to cellular need. Other models focus on how specific protein–protein interactions afforded by the Cx43 C-terminal tail can affect GJ organization and stabilization over time. These studies highlight the complexity of the dynamic ID and surrounding space in which GJs intermingle with other ion channels important for membrane depolarization. Future work is required to elucidate how these trafficking and organizational mechanisms, which could function in concert, determine intercellular conductance in context of specific cardiac demands and remodeling processes.
CONCLUSIONS
To achieve precise and timely Cx43 expression, multiple regulatory steps have been identified to include initial transcription in the nucleus, translational control, ER to Golgi transport, directed trafficking along the cytoskeletal network, and finally delivery and organization within the ID. Together, the studies presented expand on previous knowledge of the ID as a collection of separate protein entities either involved in communication or adhesion. The GJ and the surrounding perinexus space are appreciated as a highly dynamic structure within which resident channels and adhesion proteins intermingle, while interacting with scaffolding proteins and cytoskeletal elements to effect intercellular coupling and excitation. We bring to light that in addition to previously known mechanisms influencing how much Cx43 is expressed in the cardiomyocyte, alternative translation of Gja1 mRNA generates truncated Cx43 isoforms that can act as autoregulatory chaperones during ER to Golgi transport. The need to understand cytoskeletal control of the specificity and directionality of Cx43 trafficking toward the GJ plaque is also highlighted.
Cx43 is expressed in most excitable as well as nonexcitable tissues, and is indispensable for their development and function. Various pathologic conditions, including cancer and heart disease, are characterized by altered Cx43 expression and cellular distribution. Together the studies presented illustrate the importance of Cx43 in maintaining cellular communication and tissue function, and emphasize the need to identify specific mechanisms regulating the Cx43 lifecycle. The field is rapidly evolving, driven by the need to understand how perturbations of these highly regulated processes contribute to disease progression in a variety of tissues, and introducing new therapeutic classes such as mTOR inhibitors to regulate cell–cell coupling. This knowledge will be key for clinical interventions aimed at affecting intercellular communication in patients with heart failure, cancer, and other common maladies.
ACKNOWLEDGMENTS
We thank Drs. James W. Smyth and Matthew L. Wheeler for helpful discussion and critical review of this manuscript. SS Zhang and RM Shaw are supported by the American Heart Association (Postdoctoral and Established Investigator Awards), as well as by the NIH/NHLBI (RO1).
Declaration of interest: The authors report no declartions of interest. The authors alone are responsible for the content and writing of the paper.
REFERENCES
- Agullo-Pascual E, Reid DA, Keegan S, Sidhu M, Fenyo D, Rothenberg E, Delmar M (2013). Super-resolution fluorescence microscopy of the cardiac connexome reveals plakophilin-2 inside the connexin43 plaque. Cardiovasc Res. 100: 231–240.
- Ai X, Pogwizd SM (2005). Connexin 43 downregulation and dephosphorylation in nonischemic heart failure is associated with enhanced colocalized protein phosphatase type 2A. Circ Res. 96: 54–63.
- Akar FG, Nass RD, Hahn S, Cingolani E, Shah M, Hesketh GG, Disilvestre D, Tunin RS, Kass DA, Tomaselli GF (2007). Dynamic changes in conduction velocity and gap junction properties during development of pacing-induced heart failure. Am J Physiol Heart Circ Physiol. 293: H1223–H1230.
- Akar FG, Spragg DD, Tunin RS, Kass DA, Tomaselli GF (2004). Mechanisms underlying conduction slowing and arrhythmogenesis in nonischemic dilated cardiomyopathy. Circ Res. 95: 717–725.
- Akkerman M, Overdijk EJ, Schel JH, Emons AM, Ketelaar T (2011). Golgi body motility in the plant cell cortex correlates with actin cytoskeleton organization. Plant Cell Physiol. 52: 1844–1855.
- Angst BD, Khan LU, Severs NJ, Whitely K, Rothery S, Thompson RP, Magee AI, Gourdie RG (1997). Dissociated spatial patterning of gap junctions and cell adhesion junctions during postnatal differentiation of ventricular myocardium. Circ Res. 80: 88–94.
- Aschenbrenner L, Naccache SN, Hasson T (2004). Uncoated endocytic vesicles require the unconventional myosin, Myo6, for rapid transport through actin barriers. Mol Biol Cell. 15: 2253–2263.
- Baker SM, Kim N, Gumpert AM, Segretain D, Falk MM (2008). Acute internalization of gap junctions in vascular endothelial cells in response to inflammatory mediator-induced G-protein coupled receptor activation. FEBS Lett. 582: 4039–4046.
- Bakker ML, Boink GJ, Boukens BJ, Verkerk AO, VAN Den Boogaard M, Den Haan AD, Hoogaars WM, Buermans HP, DE Bakker JM, Seppen J, Tan HL, Moorman AF, ‘T Hoen PA, Christoffels VM (2012). T-box transcription factor TBX3 reprogrammes mature cardiac myocytes into pacemaker-like cells. Cardiovasc Res. 94: 439–449.
- Bakker ML, Boukens BJ, Mommersteeg MT, Brons JF, Wakker V, Moorman AF, Christoffels VM (2008). Transcription factor Tbx3 is required for the specification of the atrioventricular conduction system. Circ Res. 102: 1340–1349.
- Barker RJ, Price RL, Gourdie RG (2001). Increased co-localization of connexin43 and ZO-1 in dissociated adult myocytes. Cell Commun Adhes. 8: 205–208.
- Barker RJ, Price RL, Gourdie RG (2002). Increased association of ZO-1 with connexin43 during remodeling of cardiac gap junctions. Circ Res. 90: 317–324.
- Beardslee MA, Laing JG, Beyer EC, Saffitz JE (1998). Rapid turnover of connexin43 in the adult rat heart. Circ Res. 83: 629–635.
- Beardslee MA, Lerner DL, Tadros PN, Laing JG, Beyer EC, Yamada KA, Kleber AG, Schuessler RB, Saffitz JE (2000). Dephosphorylation and intracellular redistribution of ventricular connexin43 during electrical uncoupling induced by ischemia. Circ Res. 87: 656–662.
- Benson DW, Silberbach GM, Kavanaugh-Mchugh A, Cottrill C, Zhang Y, Riggs S, Smalls O, Johnson MC, Watson MS, Seidman JG, Seidman CE, Plowden J, Kugler JD (1999). Mutations in the cardiac transcription factor NKX2.5 affect diverse cardiac developmental pathways. J Clin Invest. 104: 1567–1573.
- Boateng SY, Goldspink PH (2008). Assembly and maintenance of the sarcomere night and day. Cardiovasc Res. 77: 667–675.
- Boukens BJ, Christoffels VM (2012). Electrophysiological patterning of the heart. Pediatr Cardiol. 33: 900–906.
- Candeias MM, Powell DJ, Roubalova E, Apcher S, Bourougaa K, Vojtesek B, Bruzzoni-Giovanelli H, Fahraeus R (2006). Expression of p53 and p53/47 are controlled by alternative mechanisms of messenger RNA translation initiation. Oncogene. 25: 6936–6947.
- Cochrane K, Su V, Lau AF (2013). The connexin43-interacting protein, CIP85, mediates the internalization of connexin43 from the plasma membrane. Cell Commun Adhes. 20: 53–66.
- D'hondt C, Iyyathurai J, Vinken M, Rogiers V, Leybaert L, Himpens B, Bultynck G (2013). Regulation of connexin- and pannexin-based channels by post-translational modifications. Biol Cell. 105: 373–398.
- Danik SB, Liu F, Zhang J, Suk HJ, Morley GE, Fishman GI, Gutstein DE (2004). Modulation of cardiac gap junction expression and arrhythmic susceptibility. Circ Res. 95: 1035–1041.
- Delmar M (2004). The intercalated disk as a single functional unit. Heart Rhythm. 1: 12–13.
- Domes K, Ding J, Lemke T, Blaich A, Wegener JW, Brandmayr J, Moosmang S, Hofmann F (2011). Truncation of murine CaV1.2 at Asp-1904 results in heart failure after birth. J Biol Chem. 286: 33863–33871.
- Duffy HS, Ashton AW, O'donnell P, Coombs W, Taffet SM, Delmar M, Spray DC (2004). Regulation of connexin43 protein complexes by intracellular acidification. Circ Res. 94: 215–222.
- Dupays L, Jarry-Guichard T, Mazurais D, Calmels T, Izumo S, Gros D, Theveniau-Ruissy M (2005). Dysregulation of connexins and inactivation of NFATc1 in the cardiovascular system of Nkx2–5 null mutants. J Mol Cell Cardiol. 38: 787–798.
- Eckardt D, Theis M, Degen J, Ott T, Van Rijen HV, Kirchhoff S, Kim JS, De Bakker JM, Willecke K (2004). Functional role of connexin43 gap junction channels in adult mouse heart assessed by inducible gene deletion. J Mol Cell Cardiol. 36: 101–110.
- Eloff BC, Lerner DL, Yamada KA, Schuessler RB, Saffitz JE, Rosenbaum DS (2001). High resolution optical mapping reveals conduction slowing in connexin43 deficient mice. Cardiovasc Res. 51: 681–690.
- Falk MM, Baker SM, Gumpert AM, Segretain D, Buckheit RW, III 2009. Gap junction turnover is achieved by the internalization of small endocytic double-membrane vesicles. Mol Biol Cell. 20: 3342–3352.
- Feldman ME, Apsel B, Uotila A, Loewith R, Knight ZA, Ruggero D, Shokat KM (2009). Active-site inhibitors of mTOR target rapamycin-resistant outputs of mTORC1 and mTORC2. PLoS Biol. 7: e38.
- Fidler LM, Wilson GJ, Liu F, Cui X, Scherer SW, Taylor GP, Hamilton RM (2009). Abnormal connexin43 in arrhythmogenic right ventricular cardiomyopathy caused by plakophilin-2 mutations. J Cell Mol Med. 13: 4219–4228.
- Fishman GI, Eddy RL, Shows TB, Rosenthal L, Leinwand LA (1991). The human connexin gene family of gap junction proteins: distinct chromosomal locations but similar structures. Genomics. 10: 250–256.
- Folkes AJ, Ahmadi K, Alderton WK, Alix S, Baker SJ, Box G, Chuckowree IS, Clarke PA, Depledge P, Eccles SA, Friedman LS, Hayes A, Hancox TC, Kugendradas A, Lensun L, Moore P, Olivero AG, Pang J, Patel S, Pergl-Wilson GH, Raynaud FI, Robson A, Saghir N, Salphati L, Sohal S, Ultsch MH, Valenti M, Wallweber HJ, Wan NC, Wiesmann C, Workman P, Zhyvoloup A, Zvelebil MJ, Shuttleworth SJ (2008). The identification of 2-(1H-indazol-4-yl)-6-(4-methanesulfonyl-piperazin-1-ylmethyl)-4-morpholin-4-yl-t hieno[3,2-d]pyrimidine (GDC-0941) as a potent, selective, orally bioavailable inhibitor of class I PI3 kinase for the treatment of cancer. J Med Chem. 51: 5522–5532.
- Fong JT, Kells RM, Falk MM (2013). Two tyrosine-based sorting signals in the Cx43 C-terminus cooperate to mediate gap junction endocytosis. Mol Biol Cell. 24: 2834–2848.
- Fong JT, Kells RM, Gumpert AM, Marzillier JY, Davidson MW, Falk MM (2012). Internalized gap junctions are degraded by autophagy. Autophagy. 8: 794–811.
- Forbes MS, Sperelakis N (1985). Intercalated discs of mammalian heart: a review of structure and function. Tissue Cell. 17: 605–648.
- Fort AG, Murray JW, Dandachi N, Davidson MW, Dermietzel R, Wolkoff AW, Spray DC (2011). In vitro motility of liver connexin vesicles along microtubules utilizes kinesin motors. J Biol Chem. 286: 22875–22885.
- Francis R, Xu X, Park H, Wei CJ, Chang S, Chatterjee B, Lo C (2011). Connexin43 modulates cell polarity and directional cell migration by regulating microtubule dynamics. PLoS One. 6: e26379.
- Fromaget C, El Aoumari A, Gros D (1992). Distribution pattern of connexin 43, a gap junctional protein, during the differentiation of mouse heart myocytes. Differentiation. 51: 9–20.
- Fu Y, Westenbroek RE, Yu FH, Clark JP, III, Marshall MR, Scheuer T, Catterall WA (2011). Deletion of the distal C terminus of CaV1.2 channels leads to loss of beta-adrenergic regulation and heart failure in vivo. J Biol Chem. 286: 12617–12626.
- Gaborit N, Sakuma R, Wylie JN, Kim KH, Zhang SS, Hui CC, Bruneau BG (2012). Cooperative and antagonistic roles for Irx3 and Irx5 in cardiac morphogenesis and postnatal physiology. Development. 139: 4007–4019.
- Gaietta G, Deerinck TJ, Adams SR, Bouwer J, Tour O, Laird DW, Sosinsky GE, Tsien RY, Ellisman MH (2002). Multicolor and electron microscopic imaging of connexin trafficking. Science. 296: 503–507.
- Gao T, Cuadra AE, Ma H, Bunemann M, Gerhardstein BL, Cheng T, Eick RT, Hosey MM (2001). C-terminal fragments of the alpha 1C (CaV1.2) subunit associate with and regulate L-type calcium channels containing C-terminal-truncated alpha 1C subunits. J Biol Chem. 276: 21089–21097.
- Gerhardstein BL, Gao T, Bunemann M, Puri TS, Adair A, Ma H, Hosey MM (2000). Proteolytic processing of the C terminus of the alpha(1C) subunit of L-type calcium channels and the role of a proline-rich domain in membrane tethering of proteolytic fragments. J Biol Chem. 275: 8556–8563.
- Giepmans BN, Verlaan I, Hengeveld T, Janssen H, Calafat J, Falk MM, Moolenaar WH (2001). Gap junction protein connexin-43 interacts directly with microtubules. Curr Biol. 11: 1364–1368.
- Go AS, Mozaffarian D, Roger VL, Benjamin EJ, Berry JD, Borden WB, Bravata DM, Dai S, Ford ES, Fox CS, Franco S, Fullerton HJ, Gillespie C, Hailpern SM, Heit JA, Howard VJ, Huffman MD, Kissela BM, Kittner SJ, Lackland DT, Lichtman JH, Lisabeth LD, Magid D, Marcus GM, Marelli A, Matchar DB, Mcguire DK, Mohler ER, Moy CS, Mussolino ME, Nichol G, Paynter NP, Schreiner PJ, Sorlie PD, Stein J, Turan TN, Virani SS, Wong ND, Woo D, Turner MB, American Heart Association Statistics C, Stroke Statistics S (2013). Heart disease and stroke statistics–2013 update: a report from the American Heart Association. Circulation. 127: e6–e245.
- Goldmuntz E, Geiger E, Benson DW (2001). NKX2.5 mutations in patients with tetralogy of fallot. Circulation. 104: 2565–2568.
- Gomez-Skarmeta JL, Modolell J (2002). Iroquois genes: genomic organization and function in vertebrate neural development. Curr Opin Genet Dev. 12: 403–408.
- Gourdie RG, Green CR, Severs NJ (1991). Gap junction distribution in adult mammalian myocardium revealed by an anti-peptide antibody and laser scanning confocal microscopy. J Cell Sci. 99: 41–55.
- Gourdie RG, Green CR, Severs NJ, Thompson RP (1992). Immunolabelling patterns of gap junction connexins in the developing and mature rat heart. Anat Embryol (Berl). 185: 363–378.
- Guerrero PA, Schuessler RB, Davis LM, Beyer EC, Johnson CM, Yamada KA, Saffitz JE (1997). Slow ventricular conduction in mice heterozygous for a connexin43 null mutation. J Clin Invest. 99: 1991–1998.
- Gutstein DE, Morley GE, Tamaddon H, Vaidya D, Schneider MD, Chen J, Chien KR, Stuhlmann H, Fishman GI (2001). Conduction slowing and sudden arrhythmic death in mice with cardiac-restricted inactivation of connexin43. Circ Res. 88: 333–339.
- Hayakawa K, Ono S, Nagaoka R, Saitoh O, Obinata T (1996). Differential assembly of cytoskeletal and sarcomeric actins in developing skeletal muscle cells in vitro. Zoolog Sci. 13: 509–517.
- Hesketh GG, Shah MH, Halperin VL, Cooke CA, Akar FG, Yen TE, Kass DA, Machamer CE, Van Eyk JE, Tomaselli GF (2010). Ultrastructure and regulation of lateralized connexin43 in the failing heart. Circ Res. 106: 1153–1163.
- Hesketh GG, Van Eyk JE, Tomaselli GF (2009). Mechanisms of gap junction traffic in health and disease. J Cardiovasc Pharmacol. 54: 263–272.
- Hirschy A, Schatzmann F, Ehler E, Perriard JC (2006). Establishment of cardiac cytoarchitecture in the developing mouse heart. Dev Biol. 289: 430–441.
- Hong TT, Cogswell R, James CA, Kang G, Pullinger CR, Malloy MJ, Kane JP, Wojciak J, Calkins H, Scheinman MM, Tseng ZH, Ganz P, De Marco T, Judge DP, Shaw RM. 2012a. Plasma BIN1 correlates with heart failure and predicts arrhythmia in patients with arrhythmogenic right ventricular cardiomyopathy. Heart Rhythm. 9: 961–967.
- Hong TT, Smyth JW, Chu KY, Vogan JM, Fong TS, Jensen BC, Fang K, Halushka MK, Russell SD, Colecraft H, Hoopes CW, Ocorr K, Chi NC, Shaw RM. 2012b. BIN1 is reduced and Cav1.2 trafficking is impaired in human failing cardiomyocytes. Heart Rhythm. 9: 812–820.
- Hoogaars WM, Barnett P, Moorman AF, Christoffels VM. 2007a. T-box factors determine cardiac design. Cell Mol Life Sci. 64: 646–660.
- Hoogaars WM, Engel A, Brons JF, Verkerk AO, DE Lange FJ, Wong LY, Bakker ML, Clout DE, Wakker V, Barnett P, Ravesloot JH, Moorman AF, Verheijck EE, Christoffels VM. 2007b. Tbx3 controls the sinoatrial node gene program and imposes pacemaker function on the atria. Genes Dev. 21: 1098–1112.
- Howard J (1997). Molecular motors: structural adaptations to cellular functions. Nature. 389: 561–567.
- Huang GY, Wessels A, Smith BR, Linask KK, Ewart JL, Lo CW (1998). Alteration in connexin 43 gap junction gene dosage impairs conotruncal heart development. Dev Biol. 198: 32–44.
- Hulme JT, Konoki K, Lin TW, Gritsenko MA, Camp DG, II Bigelow, DJ, Catterall WA (2005). Sites of proteolytic processing and noncovalent association of the distal C-terminal domain of CaV1.1 channels in skeletal muscle. Proc Natl Acad Sci U S A. 102: 5274–5279.
- Hulme JT, Yarov-Yarovoy V, Lin TW, Scheuer T, Catterall WA (2006). Autoinhibitory control of the CaV1.2 channel by its proteolytically processed distal C-terminal domain. J Physiol. 576: 87–102.
- Hunter AW, Barker RJ, Zhu C, Gourdie RG (2005). Zonula occludens-1 alters connexin43 gap junction size and organization by influencing channel accretion. Mol Biol Cell. 16: 5686–5698.
- Ingolia NT, Lareau LF, Weissman JS (2011). Ribosome profiling of mouse embryonic stem cells reveals the complexity and dynamics of mammalian proteomes. Cell. 147: 789–802.
- Itoh T, Erdmann KS, Roux A, Habermann B, Werner H, DE Camilli P (2005). Dynamin and the actin cytoskeleton cooperatively regulate plasma membrane invagination by BAR and F-BAR proteins. Dev Cell. 9: 791–804.
- Jaiswal JK, Rivera VM, Simon SM (2009). Exocytosis of post-Golgi vesicles is regulated by components of the endocytic machinery. Cell. 137: 1308–1319.
- Johnson RG, Meyer RA, Li XR, Preus DM, Tan L, Grunenwald H, Paulson AF, Laird DW, Sheridan JD (2002). Gap junctions assemble in the presence of cytoskeletal inhibitors, but enhanced assembly requires microtubules. Exp Cell Res. 275: 67–80.
- Johnstone SR, Billaud M, Lohman AW, Taddeo EP, Isakson BE (2012). Posttranslational modifications in connexins and pannexins. J Membr Biol. 245: 319–332.
- Jordan K, Solan JL, Dominguez M, Sia M, Hand A, Lampe PD, Laird DW (1999). Trafficking, assembly, and function of a connexin43-green fluorescent protein chimera in live mammalian cells. Mol Biol Cell. 10: 2033–2050.
- Kalcheva N, Qu J, Sandeep N, Garcia L, Zhang J, Wang Z, Lampe PD, Suadicani SO, Spray DC, Fishman GI (2007). Gap junction remodeling and cardiac arrhythmogenesis in a murine model of oculodentodigital dysplasia. Proc Natl Acad Sci U S A. 104: 20512–20516.
- Kapoor N, Galang G, Marban E, Cho HC (2011). Transcriptional suppression of connexin43 by TBX18 undermines cell-cell electrical coupling in postnatal cardiomyocytes. J Biol Chem. 286: 14073–14079.
- Kapoor N, Liang W, Marban E, Cho HC (2013). Direct conversion of quiescent cardiomyocytes to pacemaker cells by expression of Tbx18. Nat Biotechnol. 31: 54–62.
- Kasahara H, Ueyama T, Wakimoto H, Liu MK, Maguire CT, Converso KL, Kang PM, Manning WJ, Lawitts J, Paul DL, Berul CI, Izumo S (2003). Nkx2.5 homeoprotein regulates expression of gap junction protein connexin 43 and sarcomere organization in postnatal cardiomyocytes. J Mol Cell Cardiol. 35: 243–256.
- Kasahara H, Wakimoto H, Liu M, Maguire CT, Converso KL, Shioi T, Huang WY, Manning WJ, Paul D, Lawitts J, Berul CI, Izumo S (2001). Progressive atrioventricular conduction defects and heart failure in mice expressing a mutant Csx/Nkx2.5 homeoprotein. J Clin Invest. 108: 189–201.
- Kostin S, Dammer S, Hein S, Klovekorn WP, Bauer EP, Schaper J (2004). Connexin 43 expression and distribution in compensated and decompensated cardiac hypertrophy in patients with aortic stenosis. Cardiovasc Res. 62: 426–436.
- Kucera JP, Rohr S, Rudy Y (2002). Localization of sodium channels in intercalated disks modulates cardiac conduction. Circ Res. 91: 1176–1182.
- Lauf U, Giepmans BN, Lopez P, Braconnot S, Chen SC, Falk MM (2002). Dynamic trafficking and delivery of connexons to the plasma membrane and accretion to gap junctions in living cells. Proc Natl Acad Sci U S A. 99: 10446–10451.
- Li J, Levin MD, Xiong Y, Petrenko N, Patel VV, Radice GL (2008). N-cadherin haploinsufficiency affects cardiac gap junctions and arrhythmic susceptibility. J Mol Cell Cardiol. 44: 597–606.
- Lowe JS, Palygin O, Bhasin N, Hund TJ, Boyden PA, Shibata E, Anderson ME, Mohler PJ (2008). Voltage-gated Nav channel targeting in the heart requires an ankyrin-G dependent cellular pathway. J Cell Biol. 180: 173–186.
- Lubkemeier I, Requardt RP, Lin X, Sasse P, Andrie R, Schrickel JW, Chkourko H, Bukauskas FF, Kim JS, Frank M, Malan D, Zhang J, Wirth A, Dobrowolski R, Mohler PJ, Offermanns S, Fleischmann BK, Delmar M, Willecke K (2013). Deletion of the last five C-terminal amino acid residues of connexin43 leads to lethal ventricular arrhythmias in mice without affecting coupling via gap junction channels. Basic Res Cardiol. 108: 348.
- Lyons I, Parsons LM, Hartley L, Li R, Andrews JE, Robb L, Harvey RP (1995). Myogenic and morphogenetic defects in the heart tubes of murine embryos lacking the homeo box gene Nkx2–5. Genes Dev. 9: 1654–1666.
- Maass K, Chase SE, Lin X, Delmar M (2009). Cx43 CT domain influences infarct size and susceptibility to ventricular tachyarrhythmias in acute myocardial infarction. Cardiovasc Res. 84: 361–367.
- Maass K, Shibayama J, Chase SE, Willecke K, Delmar M (2007). C-terminal truncation of connexin43 changes number, size, and localization of cardiac gap junction plaques. Circ Res. 101: 1283–1291.
- Majoul IV, Gao L, Betzig E, Onichtchouk D, Butkevich E, Kozlov Y, Bukauskas F, Bennett MV, Lippincott-Schwartz J, Duden R (2013). Fast structural responses of gap junction membrane domains to AB5 toxins. Proc Natl Acad Sci U S A. 110: E4125–4133.
- Malhotra JD, Thyagarajan V, Chen C, Isom LL (2004). Tyrosine-phosphorylated and nonphosphorylated sodium channel beta1 subunits are differentially localized in cardiac myocytes. J Biol Chem. 279: 40748–40754.
- Marquez-Rosado L, Solan JL, Dunn CA, Norris RP, Lampe PD (2012). Connexin43 phosphorylation in brain, cardiac, endothelial and epithelial tissues. Biochim Biophys Acta. 1818: 1985–1992.
- Misumi Y, Misumi Y, Miki K, Takatsuki A, Tamura G, Ikehara Y (1986). Novel blockade by brefeldin A of intracellular transport of secretory proteins in cultured rat hepatocytes. J Biol Chem. 261: 11398–11403.
- Morley GE, Vaidya D, Samie FH, Lo C, Delmar M, Jalife J (1999). Characterization of conduction in the ventricles of normal and heterozygous Cx43 knockout mice using optical mapping. J Cardiovasc Electrophysiol. 10: 1361–1375.
- Muhlfeld C, Richter J (2006). High-pressure freezing and freeze substitution of rat myocardium for immunogold labeling of connexin 43. Anat Rec A Discov Mol Cell Evol Biol. 288: 1059–1067.
- Musil LS, Goodenough DA (1993). Multisubunit assembly of an integral plasma membrane channel protein, gap junction connexin43, occurs after exit from the ER. Cell. 74: 1065–1077.
- Noorman M, Van Der Heyden MA, van Veen TA, Cox MG, Hauer RN, De Bakker JM, van Rijen HV (2009). Cardiac cell-cell junctions in health and disease: Electrical versus mechanical coupling. J Mol Cell Cardiol. 47: 23–31.
- Nygren A, Olson ML, Chen KY, Emmett T, Kargacin G, Shimoni Y (2007). Propagation of the cardiac impulse in the diabetic rat heart: reduced conduction reserve. J Physiol. 580: 543–560.
- Olbina G, Eckhart W (2003). Mutations in the second extracellular region of connexin 43 prevent localization to the plasma membrane, but do not affect its ability to suppress cell growth. Mol Cancer Res. 1: 690–700.
- Oxford EM, Musa H, Maass K, Coombs W, Taffet SM, Delmar M (2007). Connexin43 remodeling caused by inhibition of plakophilin-2 expression in cardiac cells. Circ Res. 101: 703–711.
- Oyamada M, Takebe K, Oyamada Y (2013). Regulation of connexin expression by transcription factors and epigenetic mechanisms. Biochim Biophys Acta. 1828: 118–133.
- Palatinus JA, Gourdie RG (2007). Xin and the art of intercalated disk maintenance. Am J Physiol Heart Circ Physiol. 293: H2626–2628.
- Palatinus JA, O'quinn MP, Barker RJ, Harris BS, Jourdan J, Gourdie RG (2011). ZO-1 determines adherens and gap junction localization at intercalated disks. Am J Physiol Heart Circ Physiol. 300: H583–594.
- Palatinus JA, Rhett JM, Gourdie RG (2012). The connexin43 carboxyl terminus and cardiac gap junction organization. Biochim Biophys Acta. 1818: 1831–1843.
- Peters NS, Coromilas J, Severs NJ, Wit AL (1997). Disturbed connexin43 gap junction distribution correlates with the location of reentrant circuits in the epicardial border zone of healing canine infarcts that cause ventricular tachycardia. Circulation. 95: 988–996.
- Peters NS, Green CR, Poole-Wilson PA, Severs NJ (1993). Reduced content of connexin43 gap junctions in ventricular myocardium from hypertrophied and ischemic human hearts. Circulation. 88: 864–875.
- Peters NS, Severs NJ, Rothery SM, Lincoln C, Yacoub MH, Green CR (1994). Spatiotemporal relation between gap junctions and fascia adherens junctions during postnatal development of human ventricular myocardium. Circulation. 90: 713–725.
- Petitprez S, Zmoos AF, Ogrodnik J, Balse E, Raad N, El-Haou S, Albesa M, Bittihn P, Luther S, Lehnart SE, Hatem SN, Coulombe A, Abriel H (2011). SAP97 and dystrophin macromolecular complexes determine two pools of cardiac sodium channels Nav1.5 in cardiomyocytes. Circ Res. 108: 294–304.
- Pfeifer I, Anderson C, Werner R, Oltra E (2004). Redefining the structure of the mouse connexin43 gene: selective promoter usage and alternative splicing mechanisms yield transcripts with different translational efficiencies. Nucleic Acids Res. 32: 4550–4562.
- Piehl M, Lehmann C, Gumpert A, Denizot JP, Segretain D, Falk MM (2007). Internalization of large double-membrane intercellular vesicles by a clathrin-dependent endocytic process. Mol Biol Cell. 18: 337–347.
- Qu C, Gardner P, Schrijver I (2009). The role of the cytoskeleton in the formation of gap junctions by Connexin 30. Exp Cell Res. 315: 1683–1692.
- Reaume AG, De Sousa PA, Kulkarni S, Langille BL, Zhu D, Davies TC, Juneja SC, Kidder GM, Rossant J (1995). Cardiac malformation in neonatal mice lacking connexin43. Science. 267: 1831–1834.
- Remo BF, Qu J, Volpicelli FM, Giovannone S, Shin D, Lader J, Liu FY, Zhang J, Lent DS, Morley GE, Fishman GI (2011). Phosphatase-resistant gap junctions inhibit pathological remodeling and prevent arrhythmias. Circ Res. 108: 1459–1466.
- Rhee DY, Zhao XQ, Francis RJ, Huang GY, Mably JD, Lo CW (2009). Connexin 43 regulates epicardial cell polarity and migration in coronary vascular development. Development. 136: 3185–3193.
- Rhett JM, Jourdan J, Gourdie RG (2011). Connexin 43 connexon to gap junction transition is regulated by zonula occludens-1. Mol Biol Cell. 22: 1516–1528.
- Rhett JM, Ongstad EL, Jourdan J, Gourdie RG (2012). Cx43 associates with Na(v)1.5 in the cardiomyocyte perinexus. J Membr Biol. 245: 411–422.
- Rhett JM, Veeraraghavan R, Poelzing S, Gourdie RG (2013). The perinexus: sign-post on the path to a new model of cardiac conduction?Trends Cardiovasc Med. 23: 222–228.
- Rodriguez-Sinovas A, Boengler K, Cabestrero A, Gres P, Morente M, Ruiz-Meana M, Konietzka I, Miro E, Totzeck A, Heusch G, Schulz R, Garcia-Dorado D (2006). Translocation of connexin 43 to the inner mitochondrial membrane of cardiomyocytes through the heat shock protein 90-dependent TOM pathway and its importance for cardioprotection. Circ Res. 99: 93–101.
- Rogers SL, Gelfand VI (2000). Membrane trafficking, organelle transport, and the cytoskeleton. Curr Opin Cell Biol. 12: 57–62.
- Ross JL, Ali MY, Warshaw DM (2008). Cargo transport: molecular motors navigate a complex cytoskeleton. Curr Opin Cell Biol. 20: 41–47.
- Sato PY, Coombs W, Lin X, Nekrasova O, Green KJ, Isom LL, Taffet SM, Delmar M (2011). Interactions between ankyrin-G, Plakophilin-2, and Connexin43 at the cardiac intercalated disc. Circ Res. 109: 193–201.
- Schiavi A, Hudder A, Werner R (1999). Connexin43 mRNA contains a functional internal ribosome entry site. FEBS Lett. 464: 118–122.
- Schott JJ, Benson DW, Basson CT, Pease W, Silberbach GM, Moak JP, Maron BJ, Seidman CE, Seidman JG (1998). Congenital heart disease caused by mutations in the transcription factor NKX2–5. Science. 281: 108–111.
- Schuh M (2011). An actin-dependent mechanism for long-range vesicle transport. Nat Cell Biol. 13: 1431–1436.
- Scott MP, Tamkun JW, Hartzell GW, III 1989. The structure and function of the homeodomain. Biochim Biophys Acta. 989: 25–48.
- Sepp R, Severs NJ, Gourdie RG (1996). Altered patterns of cardiac intercellular junction distribution in hypertrophic cardiomyopathy. Heart. 76: 412–417.
- Severs NJ (1989). Gap junction shape and orientation at the cardiac intercalated disk. Circ Res. 65: 1458–1462.
- Shaw RM, Fay AJ, Puthenveedu MA, Von Zastrow M, Jan YN, Jan LY (2007). Microtubule plus-end-tracking proteins target gap junctions directly from the cell interior to adherens junctions. Cell. 128: 547–560.
- Shaw RM, Rudy Y (1997). Ionic mechanisms of propagation in cardiac tissue. Roles of the sodium and L-type calcium currents during reduced excitability and decreased gap junction coupling. Circ Res. 81: 727–741.
- Smith JH, Green CR, Peters NS, Rothery S, Severs NJ (1991). Altered patterns of gap junction distribution in ischemic heart disease. An immunohistochemical study of human myocardium using laser scanning confocal microscopy. Am J Pathol. 139: 801–821.
- Smith TD, Mohankumar A, Minogue PJ, Beyer EC, Berthoud VM, Koval M (2012). Cytoplasmic amino acids within the membrane interface region influence connexin oligomerization. J Membr Biol. 245: 221–230.
- Smyth JW, Hong TT, Gao D, Vogan JM, Jensen BC, Fong TS, Simpson PC, Stainier DY, Chi NC, Shaw RM (2010). Limited forward trafficking of connexin 43 reduces cell-cell coupling in stressed human and mouse myocardium. J Clin Invest. 120: 266–279.
- Smyth JW, Shaw RM (2012). The gap junction life cycle. Heart Rhythm. 9: 151–153.
- Smyth JW, Shaw RM (2013). Autoregulation of Connexin43 Gap Junction Formation by Internally Translated Isoforms. Cell Rep.
- Smyth JW, Vogan JM, Buch PJ, Zhang SS, Fong TS, Hong TT, Shaw RM (2012). Actin cytoskeleton rest stops regulate anterograde traffic of connexin 43 vesicles to the plasma membrane. Circ Res. 110: 978–989.
- Sohl G, Willecke K (2003). An update on connexin genes and their nomenclature in mouse and man. Cell Commun Adhes. 10: 173–180.
- Sohl G, Willecke K (2004). Gap junctions and the connexin protein family. Cardiovasc Res. 62: 228–232.
- Su V, Nakagawa R, Koval M, Lau AF (2010). Ubiquitin-independent proteasomal degradation of endoplasmic reticulum-localized connexin43 mediated by CIP75. J Biol Chem. 285: 40979–40990.
- Sullivan R, Ruangvoravat C, Joo D, Morgan J, Wang BL, Wang XK, Lo CW (1993). Structure, sequence and expression of the mouse Cx43 gene encoding connexin 43. Gene. 130: 191–199.
- Tanaka M, Chen Z, Bartunkova S, Yamasaki N, Izumo S (1999). The cardiac homeobox gene Csx/Nkx2.5 lies genetically upstream of multiple genes essential for heart development. Development. 126: 1269–1280.
- Theiss C, Meller K (2002). Microinjected anti-actin antibodies decrease gap junctional intercellular commmunication in cultured astrocytes. Exp Cell Res. 281: 197–204.
- Thevenin AF, Kowal TJ, Fong JT, Kells RM, Fisher CG, Falk MM (2013). Proteins and mechanisms regulating gap-junction assembly, internalization, and degradation. Physiology (Bethesda). 28: 93–116.
- Thibodeau IL, Xu J, Li Q, Liu G, Lam K, Veinot JP, Birnie DH, Jones DL, Krahn AD, Lemery R, Nicholson BJ, Gollob MH (2010). Paradigm of genetic mosaicism and lone atrial fibrillation: physiological characterization of a connexin 43-deletion mutant identified from atrial tissue. Circulation. 122: 236–244.
- Thomas SA, Schuessler RB, Berul CI, Beardslee MA, Beyer EC, Mendelsohn ME, Saffitz JE (1998). Disparate effects of deficient expression of connexin43 on atrial and ventricular conduction: evidence for chamber-specific molecular determinants of conduction. Circulation. 97: 686–691.
- Thomas T, Jordan K, Laird DW (2001). Role of cytoskeletal elements in the recruitment of Cx43-GFP and Cx26-YFP into gap junctions. Cell Commun Adhes. 8: 231–236.
- Toyofuku T, Yabuki M, Otsu K, Kuzuya T, Hori M, Tada M (1998). Direct association of the gap junction protein connexin-43 with ZO-1 in cardiac myocytes. J Biol Chem. 273: 12725–12731.
- Unwin PN, Zampighi G (1980). Structure of the junction between communicating cells. Nature. 283: 545–549.
- Uzzaman M, Honjo H, Takagishi Y, Emdad L, Magee AI, Severs NJ, Kodama I (2000). Remodeling of gap junctional coupling in hypertrophied right ventricles of rats with monocrotaline-induced pulmonary hypertension. Circ Res. 86: 871–878.
- Van Norstrand DW, Asimaki A, Rubinos C, Dolmatova E, Srinivas M, Tester DJ, Saffitz JE, Duffy HS, Ackerman MJ (2012). Connexin43 mutation causes heterogeneous gap junction loss and sudden infant death. Circulation. 125: 474–481.
- van Rijen HV, Eckardt D, Degen J, Theis M, Ott T, Willecke K, Jongsma HJ, Opthof T, De Bakker JM (2004). Slow conduction and enhanced anisotropy increase the propensity for ventricular tachyarrhythmias in adult mice with induced deletion of connexin43. Circulation. 109: 1048–1055.
- van Veen AA, van Rijen HV, Opthof T (2001). Cardiac gap junction channels: modulation of expression and channel properties. Cardiovasc Res. 51: 217–229.
- van Zeijl L, Ponsioen B, Giepmans BN, Ariaens A, Postma FR, Varnai P, Balla T, Divecha N, Jalink K, Moolenaar WH (2007). Regulation of connexin43 gap junctional communication by phosphatidylinositol 4,5-bisphosphate. J Cell Biol. 177: 881–891.
- Wiese C, Grieskamp T, Airik R, Mommersteeg MT, Gardiwal A, DE Gier-De Vries C, Schuster-Gossler K, Moorman AF, Kispert A, Christoffels VM (2009). Formation of the sinus node head and differentiation of sinus node myocardium are independently regulated by Tbx18 and Tbx3. Circ Res. 104: 388–397.
- Xu X, Francis R, Wei CJ, Linask KL, Lo CW (2006). Connexin 43-mediated modulation of polarized cell movement and the directional migration of cardiac neural crest cells. Development. 133: 3629–3639.
- Ya J, Erdtsieck-Ernste EB, De Boer PA, Van Kempen MJ, Jongsma H, Gros D, Moorman AF, Lamers WH (1998). Heart defects in connexin43-deficient mice. Circ Res. 82: 360–366.
- Zhang SS, Kim KH, Rosen A, Smyth JW, Sakuma R, Delgado-Olguin P, Davis M, Chi NC, Puviindran V, Gaborit N, Sukonnik T, Wylie JN, Brand-Arzamendi K, Farman GP, Kim J, Rose RA, Marsden PA, Zhu Y, Zhou YQ, Miquerol L, Henkelman RM, Stainier DY, Shaw RM, Hui CC, Bruneau BG, Backx PH (2011). Iroquois homeobox gene 3 establishes fast conduction in the cardiac His-Purkinje network. Proc Natl Acad Sci U S A. 108: 13576–13581.
- Zhang SS, Shaw RM (2013). Multilayered regulation of cardiac ion channels. Biochim Biophys Acta, 1833: 876–885.