Abstract
This review article discusses a recent work using engineered cardiac cells to study the function of the intercalated disc putting emphasis on mechanical and electrical coupling.
INTRODUCTION: ENGINEERING CARDIAC TISSUE
The myocardium consists of a complex three-dimensional network of excitable cells, which are excited by a propagating electrical impulse, which in turn acts as a trigger for and a modulator of contraction of individual myocytes. The electrical impulse (action potential) is created by a complex interplay of ion currents flowing through multiple ion channels. Exact understanding of the process of impulse propagation requires knowledge not only about the mechanism of action potential generation but also about so-called passive electrical properties of the myocardium which include cell dimensions, cell membrane capacity and resistance, the resistance of the cytoplasmic space, and the gap junction plaques containing low-resistance gap junction channels. (CitationKleber & Rudy, 2004) There have been many experimental attempts to determine these properties, which mostly used experimental and theoretical models derived from nerve tissue. Inherent to all these models is the specific selection of a cardiac structure (Purkinje fiber segments and cylindrical papillary muscles) with the aim to use a defined arrangement of myocytes that would allow deduction of cellular electrical parameters from experiments involving the passage of electrical currents across a multicellular, functional syncytium (CitationKleber & Riegger, 1987).
In the 1970s CitationLieberman et al. (1972, Citation1975) produced “1-dimensional” artificial strands of cardiac myocytes growing along nylon filaments or in agar grooves to determine passive electrical properties and propagation. Subsequently, two-dimensional engineered cell cultures fabricated by photoresist microlithography were used by CitationRohr et al. (1991) and later in many studies of our laboratory to engineer defined cardiac tissue geometries in cell cultures for studying cardiac electrical impulse propagation and defining basic biophysical rules which determine the effect of extracellular defibrillation shocks (CitationFast & Kleber, 1993; CitationKucera et al., 1998, Citation2001; CitationRohr et al., 1997, Citation1998; CitationGillis et al., 1996; CitationFast et al., 1998). A quantum step in terms of spatial resolution in tissue and cell engineering was made by the introduction of soft-microlithography (CitationSinghvi et al., 1994). This technique, which enables the engineering of single cells and multicellular preparations with micrometer resolution on defined extracellular substrates, was applied to myocardial tissue and cells by Parker et al. (CitationFeinberg et al., 2007; CitationBray et al., 2008; CitationGeisse et al., 2009; CitationMcCain & Parker, 2011) to determine shape and arrangement of contractile proteins and the dependence of contractile force generation on cell geometry (length/width ratio) and the organization of the contractile apparatus.
This brief review article focuses on engineering cardiac myocyte pairs and the cardiac cell junction using soft-microlithography to study mechanotransduction at the intercalated disc (ID) and the effects of genetic connexin ablation and heterogeneous connexin expression on electrical impulse transfer. In the classical view, it was perceived that the cardiac cell junction or ID would contain two important functional elements, the mechanical junctions (fascia adherens, desmosomal complexes, and area composita) (CitationLi & Radice, 2010) and gap junctional plaques and ribbons (CitationHoyt et al., 1989) consisting of gap junction channels. These channels, formed by connexin proteins, provide a low resistance pathway to electrical currents and small regulatory molecules (CitationHarris, 2001; CitationValiunas et al., 2005) and thus play a key role in electrical cell-to-cell impulse transfer. More recently it has become evident that the ID hosts ion channels as a third class of functional proteins, critically important to cell function (CitationMaier et al., 2004; CitationPetitprez et al., 2011; CitationMilstein et al., 2012; CitationBalse et al., 2012). Inherent to this triad of functional protein complexes is mutual regulatory interactions. Thus it has been shown that ablation of plakophyllin-2 (PKP-2), a desmosomal protein containing armadillo repeats, decreases the inward Na+ current carried by Nav1.5 (CitationSato et al., 2009). Likewise, genetic ablation of connexin also modulates Nav1.5, (Desplantez et al., 2012) as will be discussed in the third section of this article. A further aspect of this fascinating story of interaction between closely apposed protein complexes of the ID, exerting entirely different functions, relates to arrhythmogenic cardiomyopathy. This disease is primarily due to mutations in desmosomal proteins, (CitationCampuzano et al., 2013) but leads to change in expression and/or malfunction of the partner protein complexes in the ID (CitationAsimaki et al., 2009; CitationNoorman et al., 2013). This is a further interesting example showing that the analysis of a disease mechanism can amend to new knowledge about basic cellular function. Furthermore, IDs are exposed to highly dynamic mechanical forces because they exist at the interface of actively contracting cardiac myocytes. Studies are increasingly revealing that mechanical forces are important regulators of cell–cell coupling, (CitationZhuang et al., 2000; CitationYamada et al., 2005; CitationSalameh et al., 2010) motivating new hypotheses about the mechanosensitivity of processes related to ID assembly, structure, and function.
ENGINEERING CARDIAC CELL PAIRS AND THE INTERCELLULAR JUNCTION
Mechanical coupling between cardiac cells is assumed by adherens junctions and desmosomes, (CitationLi & Radice, 2010; CitationBorrmann et al., 2006) which couple extracellularly to corresponding complexes of neighboring cells and intracellularly to myofibrils and intermediate filaments. Thus, forces generated by the cytoskeleton are exerted against the ID (and vice versa), which must maintain the structural integrity of the tissue while exposed to mechanical stress. The specific location, diverse composition, and multiple inputs and outputs of the ID suggest that there are complex biophysical factors and molecular feedback mechanisms that regulate its structure and function. Although the structure of the ID has been thoroughly studied in explanted animal (CitationFishman et al., 1990; CitationHirschy et al., 2006; CitationOxford et al., 2007) and human (CitationAsimaki et al., 2009; CitationPeters et al., 1994; CitationSepp et al., 1996) hearts, ex vivo systems have limitations in identifying structure–function relationships and underlying mechanisms due to heterogeneities and concurrent remodeling processes that occur in the whole heart. Thus, in vitro models are essential for isolating the effects of individual stimuli on the structure and function of the ID. By leveraging techniques in tissue engineering, we and others have begun to develop more sophisticated, quantitative in vitro systems to reveal not only novel structure–function relationships at the ID, but also how intracellular and extracellular factors, such as cytoskeletal architecture or extracellular matrix elasticity, regulate these relationships.
Adherens junctions have been primarily studied in non-cardiac cell types, such as endothelial cells, but many of the lessons learned from other cell types can be applied to the cardiac ID. In cardiac myocytes and other adherent cells, mechanical forces generated by the cytoskeleton are exerted against either the extracellular matrix via cell-matrix adhesions (CitationSchiller & Fassler, 2013) or neighboring cells via cell–cell adhesions (CitationTwiss & de Rooij, 2013). The field of cellular biomechanics has developed tools to quantify the magnitude and distribution of forces generated by a single cell against its extracellular matrix by culturing cells on compliant hydrogels (CitationButler et al., 2002) or arrays of flexible microposts (CitationSniadecki & Chen, 2007). By deriving a simple force balance between cell-matrix and cell–cell adhesions, these same techniques have been adapted to quantify cellular forces transmitted to the cell–cell junction in cell pairs (CitationMaruthamuthu et al., 2011; CitationLiu et al., 2010). Results have shown that, similar to focal adhesions, cell–cell junctions are mechanosensitive and grow or shrink in response to increasing or decreasing cytoskeletal tension, respectively (CitationMaruthamuthu et al., 2011; CitationLiu et al., 2010, Citationle Duc et al., 2010). Also similar to focal adhesions, cell–cell adhesions are active sites of signaling, (CitationWeber et al., 2011) indicating that, in addition to mechanically coupling cells together to maintain tissue structure, cell–cell junctions are also sites of dynamic mechanotransduction. Because adherens junctions proteins are also expressed in cardiac myocytes, these results can be extrapolated to suggest that IDs also translate mechanical forces into biochemical signals to regulate processes inside the myocyte.
Because adherens junctions are mechanosensitive, myocyte contractility is likely an important regulator of ID development. The ID co-develops with myofibrils during prenatal and postnatal growth, not reaching full maturity in humans until six years of age (CitationPeters et al., 1994). Early in development, cell–cell junction proteins are found along all cell borders with minimal spatial organization. Over the course of development, cell–cell junction proteins localize to a major extent to longitudinal borders while cell-matrix adhesions form costameres registered with the Z-lines (CitationHoyt et al., 1989; CitationHirschy et al., 2006; CitationPeters et al., 1994; CitationWu et al., 2002; CitationAngst et al., 1997). Because much of this organization occurs during post-natal growth, while myofibrils are also maturing and generating increasing magnitudes of force, cardiac myocytes must be remodeling adhesions while actively contracting. The temporal synchrony of the maturation of the contractile apparatus with the ID indicates that adhesion remodeling is a dynamic process where mechanics likely plays an important role. To better understand the spatiotemporal co-development of ID structures with the cytoskeleton, we cultured pairs of cardiac myocytes on micropatterned islands of fibronectin on compliant polyacrylamide gels with physiological elastic moduli (). With this technique, we could independently modulate tissue geometry and extracelluar matrix elasticity and monitor cytoskeletal maturation, force generation, and cell–cell junction formation over time in culture in a controlled setting (CitationMcCain et al., 2012a). At early time points in culture, myocytes were contracting but not well-coupled, as contractile forces were transmitted through focal adhesions to the extracellular matrix near the cell–cell interface. Paired myocytes were also not completely synchronous. Over time, the magnitude of contractile force increased while, concurrently, focal adhesions disassembled at the cell–cell interface and adherens junctions developed, causing forces to be transmitted to the newly formed cell–cell junction instead of the extracellular matrix. These data suggest that there is a hierarchical relationship between focal adhesions and cell–cell junctions, where focal adhesions attach the longitudinal ends of myocytes to the extracellular matrix early in development but eventually disassemble as cell–cell junctions mature and transmit contractile forces across the ID.
Figure 1. Engineering cardiac cell pairs and the intercellular junction. Panel A: Pairs of neonatal rat ventricular myocytes cultured on micropatterned fibronectin islands on polyacrylamide gels were used to study force transmission at the intercalated disc (ID). After one day of culture, myocytes expressed cell–cell junction proteins (i), but were not fully mechanically coupled, as evidenced by traction stresses generated against the extracellular matrix near the cell–cell interface (ii). After four days of culture (iii), myocytes generated insignificant amounts of traction stresses near the cell–cell interface (iv), indicating more complete mechanical coupling at the ID. i, iii: red: actin, blue: nuclei, green: β-catenin. Bars = 10 μm. Panel B: Pairs of neonatal rat ventricular myocytes cultured on micropatterned fibronectin islands were used to study electrical cell–cell coupling at the ID. Dual voltage clamp measurements paired with quantitative immunofluorescence revealed that pairs with lower length to width aspect ratios (i) had lower intercellular conductance values and less Cx43 immunosignal at the cell–cell interface compared to pairs with higher length to width aspect ratios (ii). Volumetry of Cx43 immunosignals was performed from stacks of high-resolution confocal slices after wide-field deconvolution. Panel C: Relationship between Cx43 immunosignal and intercellular conductance. The intersection with the y axis shows a lower limit for immunosignal detection at low levels of intercellular conductance (approximately 10 nS). Modified from references CitationWeber GF, et al. (2011).and CitationMatsushita T, et al. (1999).
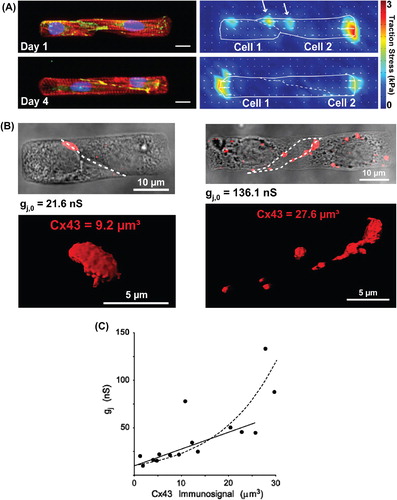
In addition to intracellular remodeling, the mechanical properties of the extracellular matrix are also undergoing dynamic changes during cardiac development, which could affect cell–cell coupling. The elastic modulus of prenatal hearts has been measured at approximately 1 kPa and increases to about 10 kPa in the adult heart (CitationEngler et al., 2008). After a myocardial infarction, the scar tissue that remains has an elastic modulus that can exceed 50 kPa (CitationBerry et al., 2006). Diseases such as hypertrophic cardiomyopathy are associated with increased fibrosis, (CitationHo et al., 2010) which also stiffens the myocardium. In vitro studies have shown that myocyte morphology, sarcomere structure, force generation (CitationEngler et al., 2008; CitationMajkut et al., 2013), and calcium cycling (CitationJacot et al., 2008) are each affected by the elastic modulus of the extracellular matrix, indicating that cardiac myocytes are highly sensitive to the mechanical properties of their microenvironment. Because of the crosstalk between cell-matrix and cell–cell adhesions, we asked how changing the elastic modulus of the extracellular matrix affected cell–cell adhesion (CitationMcCain et al., 2012a). On polyacrylamide gels with elastic moduli mimicking developing and mature hearts, pairs of cardiac myocytes coupled together with minimal focal adhesion attachment at the cell–cell interface, indicating that contractile forces were essentially transmitted completely to the cell–cell junction. However, on stiff polyacrylamide gels with elastic moduli in the range of fibrosis, focal adhesions remained attached to the cell–cell interface, indicating that forces were transmitted both to the extracellular matrix and the cell–cell junction. Thus, on gels with high elastic moduli, myocytes were not as well-coupled, which could lower the efficiency of contractile force transmission across the cell–cell interface and potentially disrupt mechanoelectrical coupling. Our results combined with in vivo observations that cell–cell and cell-matrix adhesions undergo significant remodeling in the infarct border zone (CitationPeters et al., 1997; CitationMatsushita et al., 1999) suggest that altered mechanical properties of the microenvironment could contribute to dysfunctional cell–cell coupling in pathological settings.
Many cardiac pathologies are also associated with distinct changes in myocyte geometry. For example, concentric hypertrophy is characterized by decreased length to width aspect ratio, while eccentric hyper- trophy is characterized by increased length to width aspect (CitationGerdes, 2002). By combining traction force microscopy with microcontact printing, it has been shown that myocyte shape regulates myofibril alignment (CitationBray et al., 2008) and force generation in isolated myocytes (CitationKuo et al., 2012), implying that myocyte shape remodeling impacts contractility in vivo. Using the same cell pair assay described above, we quantified how myocyte shape regulates the amount of force generated against the cell–cell junction (CitationMcCain et al., 2012a). Similar to results from single cell studies, the amount of force generated against the cell–cell junction was regulated by myocyte shape, as myocyte pairs with physiological length to width aspect ratios generated more force against the cell–cell junction compared to those with lower aspect ratios. Because cell–cell junctions are mechanosensitive, our study suggests that remodeling of myocyte shape not only affects contractility, but likely also cell–cell communication.
To elucidate the effects of myocyte shape remodeling on electrical cell–cell coupling, we performed dual voltage clamp studies to correlate cell pair geometry to gap junction structure and function (CitationMcCain et al., 2012b). By seeding cardiac myocytes on micropatterned islands of fibronectin, we controlled cell dimensions (length, height, and width) and junction geometry, as myocyte pairs consistently formed sigmoidal cell–cell junctions with relatively little variability in junction length or height. By performing quantitative immunostaining after dual voltage clamp experiments (), we identified a positive correlation between intercellular conductance and connexin43 (Cx43) immunosignal (), complementing measurements in engineered multi-cellular tissues showing correlations between Cx43 immunosignal and propagation velocity (CitationBeauchamp et al., 2004). We also found that myocyte pairs with intermediate length to width aspect ratios had the highest intercellular conductance and Cx43 density, likely because this aspect ratio provided optimal contact at the cell–cell interface for gap junction formation. Thus, gap junction density seemed to be mostly a function of cell–cell contact area. Extrapolating our results also suggested that low levels of electrical coupling are present in the absence of measureable Cx43 immunosignal. This suggests that there is a lower detection threshold for gap junction immunostaining, where low levels of Cx43 gap junction channels can be present and functioning (as evidenced by whole cell dual voltage clamp), but are too small or diffuse to be identified with immunostaining.
GENETIC DELETION OF Connexin IN ENGINEERED ATRIAL CELL PAIRS
Atrial cells are connected by Cx43 and connexin40 (Cx40). In addition a low amount of Cx45 can be detected by immunofluorescence (CitationVozzi et al., 1999; CitationSevers et al., 2008). Both Cx40 and Cx43 make pores of relatively large electrical conductance, if expressed separately in heterologous expression systems (CitationHarris, 2001; CitationCottrell & Burt, 2005; CitationCottrell et al., 2002; CitationDesplantez et al., 2011). Electrical propagation velocity in atrial tissue is markedly heterogeneous with a relatively high velocity in the Crista terminalis (forming a “propagation highway” between the sino-atrial node and the atrio-ventricular node) and Bachmann's bundle (forming a main connection between the right and left atria) (CitationKleber et al., 2003). The remainder of the atria shows a relatively low velocity, lower than ventricular myocardium, despite the presence of Cx43 and Cx40 (CitationKleber et al., 2003). The role of the individual connexins in atrial propagation, especially the question of Cx40 and Cx43 co-expression, has not been fully elucidated and is a matter of ongoing debate.
A way to resolve the functions of Cx43 and Cx40 in the atria is to measure propagation velocity in presence or absence of Cx43 or Cx40 in mice, in which one of the connexins has been ablated. In whole mice, ablation of Cx40 has shown conflicting results. In some studies, a decrease of atrial conduction time was observed and/or lengthening of the P-wave. This was taken to indicate a simple rule, namely that ablation of a connexin with a high pore conductance would lead to a decrease in cell– to-cell coupling, and accordingly to a decrease in velocity. However, in other studies it was shown that P-wave length did not increase with Cx40 deletion, and propagation in the right atrium became inhomogeneous (see discussion in reference Desplantez et al. 2012). Moreover, atrial malformations were observed with Cx40 haplo- insufficiency, suggesting that macroscopic excitation spread in such hearts might be affected by factors other than the change of connexin expression (CitationGu et al., 2003).
In an earlier study, we engineered strands from murine atrial tissue using photomicrolithography to show that linear propagation velocity at a cellular level was markedly decreased with Cx43 ablation and significantly increased versus WT with Cx40 ablation (CitationBeauchamp et al., 2006). These findings were in seeming conflict with some of the aforementioned studies in whole mice, but in accordance with studies in human hearts. In fact, studies in human hearts suggest a complex, reciprocal relationship between propagation velocity and connexin Cx43 and Cx40 expression (CitationKanagaratnam et al., 2002). This complexity might have several components: There could be interaction (1) between the atrial connexins at the level of expression at the ID, (2) at the level of gap junction channels with formation of heteromeric hemichannels (or connexons) and mixed gap junctions channels composed of all three atrial Cx subspecies, and (3) between connexins and expression of other functional complexes, such as ion channels. Thus, in our earlier work, we could show that the area occupied by Cx40 at the ID decreased with Cx43 ablation, whereas the Cx43 immunofluorescence signal increased with Cx40 ablation (CitationBeauchamp et al., 2006). These changes occurred with a constant cellular pool of Cx40 and Cx43 proteins, as demonstrated by whole cell Western blots, suggesting the possibility of interaction at the level of trafficking.
To perform high-resolution volumetric analysis of connexin immunosignals in defined atrial cell–cell junctions and assessment of intercellular conductance by dual voltage clamp, we engineered atrial cell pairs from wild type (WT) and Cx43 knock out (Cx43 KO) atrial myocytes. In contrast to ventricular pairs, the atrial ID is mostly oriented perpendicular to the main cell pair axis (). As a major finding, and in line with our previous study, we could show that germline ablation of Cx43 leads to a decrease of the immunosignals of partner connexins, Cx40 and Cx45 at the IDs (). This result points to an interaction between Cx40, Cx45, and Cx43 at the level of trafficking. This is because whole cell levels of Cx43 and Cx40 proteins remained unchanged in the case of either Cx43 or Cx40 genetic ablation (CitationBeauchamp et al., 2006). A defect of Cx40 and Cx43 trafficking has been shown in experiments involving the co-expression of mutated Cx40 protein (rare somatic mutation found in patients with lone atrial fibrillation) with wild-type Cx43 (CitationGollob et al., 2006). In this case, the mutated protein prevented the integration of the wild-type partner connexin into the ID, again suggesting mutual interaction of Cx40 and Cx43 at the level of trafficking. While very recently a number of basic papers on connexin trafficking have appeared (CitationZhang et al., 2014), the possible mechanism underlying Cx43/Cx40 interaction in atria still remains to be elucidated.
Figure 2. Engineering atrial cell pairs. Panel A: Engineered atrial cell pair. Left: Wide-field image of engineered murine atrial cell Cx43+/+ pair overlaid with the immunofluorescence of N-Cadherin (green) and Cx40 (red). Note the sharply defined interface between the two myocytes (x/z plane). Right: 3D surface rendering of Cx40 (red) immunofluorescence in the y/z plane showing plaque-like arrangement of Cx40. Image deconvolution was applied to confocal signals before reconstruction. Panel B: Relative levels of Cx43, Cx40, and Cx45 immunofluorescence signals in Cx43+/+, Cx43+/−, Cx43−/− fetal mice myocytes (levels in Cx43+/+ pairs are taken as unity). Panel C: Intercellular electrical conductances in pairs of Cx43+/+, Cx43+/−, Cx43−/− fetal (1 day before birth) mice myocytes. Modified from reference CitationDesplantez T, et al. (2012).
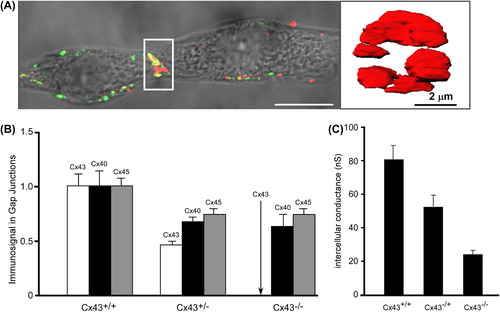
At the level of electrical cell–cell coupling, whole cell dual voltage clamp showed a massive decrease in electrical intercellular conductance by 70% associated with Cx43 ablation (). Analysis of the biophysical properties of gap junction channels was performed by assessing the bell-shaped relationship between the voltage across the gap junction channel, Vj and the relative gap junctional conductance, grel = gj,ss/gj,inst. With Cx43 ablation, the dependence of grel on Vj showed a change in shape typical for Cx40 dominating the gap junction channel properties. Determination of unitary channel conductances, γj showed not only a general shift toward higher values, but also an appearance of a bimodal distribution, with a peak at 20–30 pS. Although the explanation for these changes, and especially the appearance of a peak at low conductance values, remains in part speculative, it may indicate emergence of a population of homomeric-homotypic Cx45, (CitationDesplantez et al., 2011; CitationMoreno et al., 1995; CitationElenes et al., 2001) corresponding to the Cx45 pool, which in wild-type cells forms heteromeric connexons with Cx43. While Cx45 forms heteromers with Cx43 and possibly Cx40, (CitationCottrell & Burt, 2005; CitationMartinez et al., 2002; CitationDesplantez et al., 2004, Citation2007) experiments on the question of Cx43/Cx40 interaction (formation of Cx40/Cx43 heteromeric connexons and heterologous gap junction channels) have produced controversial results ([CitationBurt et al., 2001; CitationValiunas et al., 2001] and discussion in reference [Desplantez et al., 2012)]). While overlap of Cx43 and Cx40 immunosignals in atrium is undisputed, it remains to be shown, whether and how Cx43 and Cx40 combine in individual gap junction channels.
A third point, increasing the complexity of protein regulation at the atrial cell junction even more, is the observation of a significant decrease of INa current with ablation of Cx43 (Desplantez et al., 2012). The decrease of INa current by approximately 50% may indicate that almost the whole ID fraction of Nav1.5 is affected by Cx43 ablation. It has recently been shown that a significant fraction of cardiac Nav1.5 channels is located at the ID, (CitationPetitprez et al., 2011) together with the K+ inwardly rectifying channel Kir2.1 ion channels (CitationMilstein et al., 2012). Interestingly, silencing of plakophyllin-2 (a desmosomal protein) was also associated with a decrease of INa (CitationSato et al., 2009). In this latter case however, a shift in channel kinetics (shift of activation curve) was mentioned as the underlying cause.
In summary, the observations made in several recent studies on a multitude of interactions among the proteins at the ID forming either desmosomal complexes, ion channels or gap junctions underline the importance of complex regulatory system(s) for normal cardiac electrical (and probably mechanic) function and disease mechanisms. At the level of mechanistic explanations, these experiments show that simple hypotheses and models are not adequate to explain the physiology of the ID, and major questions remain unanswered.
ELECTRICAL CELLULAR INTERACTIONS AND PROPAGATION IN TISSUE WITH HETEROGENEOUS Cx EXPRESSION
Remodeling of gap junctions and intercellular electrical communication has been implicated in arrhythmogenesis in the setting of ventricular and atrial arrhythmias and in aging myocardium. A key and basic question concerning cardiac electrical cell-to-cell transfer relates to the theoretical possibility that, besides the transfer via gap junctions (representing an electrical resistance), a further mechanism could play a role, which would implicate so-called “field-effect” or “ephaptic” transmission, a mechanism which does not necessitate any low resistance connection (gap junction channels) between cardiac cells. This concept and the dispute over these two mechanisms is almost than five decades old (CitationWeidmann, 1966), but it has recently received new attention by (i) unexplained experimental findings, (ii) the observation that ion channels are present in the ID, and (iii) the possibility to design theoretical models of the ID containing all major functional elements (CitationKucera et al., 2002; CitationMori et al., 2008). These models can show ephaptic transmission in almost totally uncoupled tissue indeed, however, only within a very narrow setting of critical model parameters. Especially intriguing is the experimental observation that cardio-specific ablation of Cx43, the major connexin forming gap junction channels in the ventricular myocardium may only moderately affect propagation velocity. Thus in artificial strands of neonatal rat ventricular myocytes, heterogeneous germline ablation of Cx43 had only a very minor effect on propagation (CitationThomas et al., 2003). A further observation was made in mouse hearts with cardio-specific conditional ablation of Cx43. These hearts show a variable and inhomogeneous expression of Cx43, probably as a consequence of a subtotal efficiency of the Cre/flox system. In such hearts, a massive decrease of Cx43 by approximately 90%, as judged from Cx43 immunofluorescence, produced only a moderate decrease of propagation velocity (by about 50%) (CitationGutstein et al., 2001). In a further mouse model of conditional Cx43 ablation it was shown that Cx43 immunofluorescence decreased with age and became markedly heterogeneous, whereby only a marked decrease was associated with the occurrence of ventricular arrhythmias (CitationDanik et al., 2004).
These observations prompted these authors to recur to the ephaptic impulse transmission hypothesis, which was also tested in a theoretical model (CitationMori et al., 2008). In order to analyze the role of heterogeneous Cx43 expression at cellular resolution, we developed a model of engineered neonatal mouse ventricular tissue using two different cell populations (CitationBeauchamp et al., 2012). In a first stage we engineered cell pairs from two population of cells, one of wild-type ventricular myocytes carrying a GFP reporter system (CitationOkabe et al., 1997), the other consisting of Cx43−/− myocytes (further denoted as Cx43KO). demonstrates that the ID between the two WT cells shows, as expected, immunofluorescence signals for Cx43. Importantly, the engineered interface between a WT cell and a Cx43KO cell is negative for either Cx43 immunofluorescence, indicating that gap junction channels are below immunodetection threshold (CitationMcCain et al., 2012b). Intercellular electrical conductance between these two types of cells is depicted in . It can be seen, that all three combinations of IDs conduct electrical current indicating the presence of functional gap junction channels in all three cases. However, the ID between the WT–Cx43KO and Cx43KO–Cx43KO cells show a marked decrease in conductance. The bell-shaped current–voltage relationships of the three types of junctions were typical for the respective cell types, which contribute either Cx43/Cx45 or only Cx45 connexon hemichannels to the respective gap junction channels. Thus the I/V relationship of WT–Cx43KO pairs was typically asymmetric indicating the presence of only Cx45 homomers in one half of the channel and Cx43/Cx45 heteromers in the other ().
Figure 3. Engineering cell pairs with different Cx43 expression. Top view of murine ventricular cell pairs depicted from an average of superimposed confocal slices. Green: GFP; blue: DAPI; orange: Cx43. Panel A: Top: pair of Cx43+/+/Cx45+/+/GFP+/− cells. Note two large junctional plaques and smaller but abundant Cx43 fluorescence in the cytoplasmic space. Bottom: Pair composed of a Cx43+/+/Cx45+/+/GFP+/− cell (right) and a Cx43−/−/Cx45+/+ cell (left). Only one small spot of Cx43 fluorescence is present at the junction (arrow). Panel B: I/V curve of the heterogeneous junction in pair B showing asymmetry with increased voltage-dependent gating if Cx43−/− cell is hyperpolarized (typical for homomeric Cx45 connexons). Panel C: Comparison of intercellular conductance among pairs of Cx43+/+, Cx43−/+ and Cx43−/− murine ventricular myocytes. Modified from reference CitationThomas SP, et al. (2003).
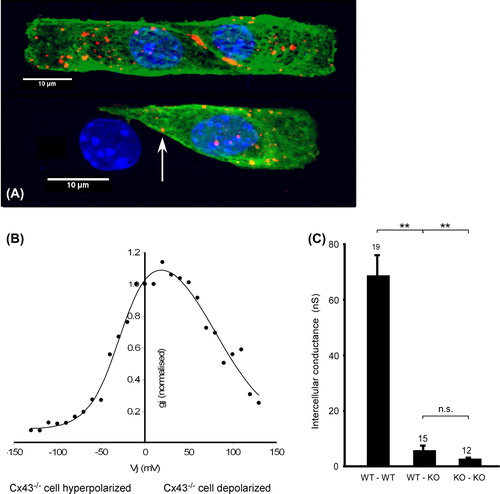
The presence of Cx43 and Cx45 in these channels was also verified by measurements of single-channel conductances. What are the implications of these findings for the mechanism of impulse transfer? First, all the cells including those with full Cx43 ablation were able to transfer the electrical impulse through gap junction channels. Second, electrical transfer is possible in absence of immunofluorescence for Cx43. Third, it can be inferred that the percentage decrease in Cx43 immunofluorescence in a cardiac cellular network with heterogeneous Cx43 expression does not reflect the percentage of WT cells. This is because only the interfaces between WT cells are expected to show Cx43 fluorescence, and there is still substantial electrical coupling at the threshold of Cx43 immunodetection. This predicts that in mixed tissue, depending on the size of the WT and Cx43−/− cell clusters in the mixture, the percentage of Cx43 immunofluorescence may largely overestimate the degree of Cx43 ablation. The consequences of heterogeneous Cx43 for impulse propagation at a cellular level was verified by multisite high-resolution optical mapping of membrane potential in synthetic strands engineered from mixtures of WT and Cx43−/− cells. In accordance with the measurements in isolated mouse hearts, propagation velocity decreased to about 50% of normal in mixtures of 50% Cx43−/− cells. Further decrease will occur only with a higher degree of Cx43 ablation. The preservation of relatively high velocities is due to a “meandering type” of propagation along a fast path of connected WT cells, while Cx43−/− cells show large local delays in excitation. Overall, these data demonstrate that (1) a very low level of Cx43 immunofluorescence is compatible with relative rapid propagation, and (2) cardiac impulse propagation in normal and pathological states does not necessitate the presence of ephaptic transmission, although co-existence of such a mechanism cannot entirely be ruled out (CitationBeauchamp et al., 2012).
OUTLOOK
New technological developments in fields such as tissue engineering, imaging, biomaterials, and microelectronics continue to enhance and refine our experimental capabilities for understanding cell–cell coupling in cardiac myocytes. In addition to the studies described above, micropatterned cardiac cell pairs have been used as a model system for understanding gap junction trafficking (CitationZhang et al., 2014) and coupling between cardiac myocytes and other cell types, such as mesenchymal stem cells (CitationPedrotty et al., 2008). New microdevices have been developed to selectively apply electrical stimulation to only one myocyte in a pair, a tool useful for studying the efficiency of gap junction coupling (CitationZhang et al., 2013). Instead of culturing pairs of cardiac myocytes on extracellular matrix proteins, another approach has been to culture isolated myocytes directly on substrates coated with cadherin such that myocytes form pseudo-cell–cell junctions directly with the substrate (CitationGanz et al., 2006; CitationChopra et al., 2011). This method allows investigators to more directly compare the biophysical properties of cell–cell versus cell-matrix adhesions. Investigators have also moved from two to three dimensions by culturing cardiac myocytes in hydrogels as another model system for understanding the spatiotemporal development of the ID (CitationZhou et al., 2013). The impact of each of the studies described in this review are dependent on constant feedback between in vivo and in vitro systems. In vivo systems are important for identifying characteristics of physiological and pathological cell–cell junction remodeling in functioning hearts, while in vitro systems are necessary for isolating individual biophysical factors to test their effects in a controlled setting. This synergy we gain with in vivo and in vitro systems enriches our understanding of IDs in health and disease and has potential to contribute to the development of novel therapeutic strategies focused on the cell-cell junction.
Declaration of interest: The authors report no declarations of interest. The authors alone are responsible for the content and writing of the paper.
REFERENCES
- Angst BD, Khan LU, Severs NJ, Whitely K, Rothery S, Thompson RP, Magee AI, Gourdie RG (1997). Dissociated spatial patterning of gap junctions and cell adhesion junctions during postnatal differentiation of ventricular myocardium. Circ Res. 80: 88–94.
- Asimaki A, Tandri H, Huang H, Halushka MK, Gautam S, Basso C, Thiene G, Tsatsopoulou A, Protonotarios N, McKenna WJ, Calkins H, Saffitz JE (2009). A new diagnostic test for arrhythmogenic right ventricular cardiomyopathy. N Engl J Med. 360: 1075–1084.
- Balse E, Steele DF, Abriel H, Coulombe A, Fedida D, Hatem SN (2012). Dynamic of ion channel expression at the plasma membrane of cardiomyocytes. Physiol Rev. 92: 1317–1358.
- Beauchamp P, Choby C, Desplantez T, de Peyer K, Green K, Yamada KA, Weingart R, Saffitz JE, Kléber AG (2004). Electrical propagation in synthetic ventricular myocyte strands from germline connexin43 knockout mice. Circ Res. 95: 170–178.
- Beauchamp P, Desplantez T, McCain ML, Li W, Asimaki A, Rigoli G, Parker KK, Saffitz JE, Kleber AG (2012). Electrical coupling and propagation in engineered ventricular myocardium with heterogeneous expression of connexin43. Circ Res. 110: 1445–1453.
- Beauchamp P, Yamada KA, Baertschi AJ, Green K, Kanter EM, Saffitz JE, Kléber AG (2006). Relative contributions of connexins 40 and 43 to atrial impulse propagation in synthetic strands of neonatal and fetal murine cardiomyocytes. Circ Res. 99: 1216–1224.
- Berry MF, Engler AJ, Woo YJ, Pirolli TJ, Bish LT, Jayasankar V, Morine KJ, Gardner TJ, Discher DE, Sweeney HL (2006). Mesenchymal stem cell injection after myocardial infarction improves myocardial compliance. Am J Physiol Heart Circ Physiol. 290: H2196–H2203.
- Borrmann CM, Grund C, Kuhn C, Hofmann I, Pieperhoff S, Franke WW (2006). The area composita of adhering junctions connecting heart muscle cells of vertebrates. II. Colocalizations of desmosomal and fascia adhaerens molecules in the intercalated disk. Eur J Cell Biol. 85: 469–485.
- Bray MA, Sheehy SP, Parker KK (2008). Sarcomere alignment is regulated by myocyte shape. Cell Motil Cytoskeleton. 65: 641–651.
- Burt JM, Fletcher AM, Steele TD, Wu Y, Cottrell GT, Kurjiaka DT (2001). Alteration of Cx43:Cx40 expression ratio in A7r5 cells. Am J Physiol Cell Physiol. 280: C500–C508.
- Butler JP, Tolić-Nørrelykke IM, Fabry B, Fredberg JJ (2002). Traction fields, moments, and strain energy that cells exert on their surroundings. Am J Physiol Cell Physiol. 282: C595–C605.
- Campuzano O, Alcalde M, Allegue C, Iglesias A, García-Pavía P, Partemi S, Oliva A, Pascali VL, Berne P, Sarquella-Brugada G, Brugada J, Brugada P, Brugada R (2013). Genetics of arrhythmogenic right ventricular cardiomyopathy. J Med Genet. 50: 280–289.
- Chopra A, Tabdanov E, Patel H, Janmey PA, Kresh JY (2011). Cardiac myocyte remodeling mediated by N-cadherin-dependent mechanosensing. Am J Physiol Heart Circ Physiol. 300: H1252–H1266.
- Cottrell GT, Burt JM (2005). Functional consequences of heterogeneous gap junction channel formation and its influence in health and disease. Biochim Biophys Acta. 1711: 126–141.
- Cottrell GT, Wu Y, Burt JM (2002). Cx40 and Cx43 expression ratio influences heteromeric/heterotypic gap junction channel properties. Am J Physiol Cell Physiol. 282: C1469–C1482.
- Danik SB, Liu F, Zhang J, Suk HJ, Morley GE, Fishman GI, Gutstein DE (2004). Modulation of cardiac gap junction expression and arrhythmic susceptibility. Circ Res. 95: 1035–1041.
- Desplantez T, Dupont E, Severs NJ, Weingart R (2007). Gap junction channels and cardiac impulse propagation. J Membr Biol. 218: 13–28.
- Desplantez T, Halliday D, Dupont E, Severs NJ, Weingart R (2011). Influence of v5/6-His tag on the properties of gap junction channels composed of connexin43, connexin40 or connexin45. J Membr Biol. 240: 139–150.
- Desplantez T, Halliday D, Dupont E, Weingart R (2004). Cardiac connexins Cx43 and Cx45: formation of diverse gap junction channels with diverse electrical properties. Pflugers Arch. 448: 363–375.
- Desplantez T, McCain ML, Beauchamp P, Rigoli G, Rothen-Rutishauser B, Parker KK, Kleber AG (2012). Connexin43 ablation in foetal atrial myocytes decreases electrical coupling, partner connexins, and sodium current. Cardiovasc Res. 94: 58–65.
- Elenes S, Martinez AD, Delmar M, Beyer EC, Moreno AP (2001). Heterotypic docking of Cx43 and Cx45 connexons blocks fast voltage gating of Cx43. Biophys J. 81: 1406–1418.
- Engler AJ, Carag-Krieger C, Johnson CP, Raab M, Tang HY, Speicher DW, Sanger JW, Sanger JM, Discher DE (2008). Embryonic cardiomyocytes beat best on a matrix with heart-like elasticity: scar-like rigidity inhibits beating. J Cell Sci. 121: 3794–3802.
- Fast VG, Kleber AG (1993). Microscopic conduction in cultured strands of neonatal rat heart cells measured with voltage-sensitive dyes. Circ Res. 73: 914–925.
- Fast VG, Rohr S, Gillis AM, Kléber AG (1998). Activation of cardiac tissue by extracellular electrical shocks: formation of ‘secondary sources’ at intercellular clefts in monolayers of cultured myocytes. Circ Res. 82: 375–385.
- Feinberg AW, Feigel A, Shevkoplyas SS, Sheehy S, Whitesides GM, Parker KK (2007). Muscular thin films for building actuators and powering devices. Science. 317: 1366–1370.
- Fishman GI, Spray DC, Leinwand LA (1990). Molecular characterization and functional expression of the human cardiac gap junction channel. J Cell Biol. 111: 589–598.
- Ganz A, Lambert M, Saez A, Silberzan P, Buguin A, Mège RM, Ladoux B (2006). Traction forces exerted through N-cadherin contacts. Biol Cell. 98: 721–730.
- Geisse NA, Sheehy SP, Parker KK (2009). Control of myocyte remodeling in vitro with engineered substrates. In Vitro Cell Dev Biol Anim. 45: 343–50.
- Gerdes AM (2002). Cardiac myocyte remodeling in hypertrophy and progression to failure. J Card Fail. 8: S264–S268.
- Gillis AM, Fast VG, Rohr S, Kléber AG (1996). Spatial changes in transmembrane potential during extracellular electrical shocks in cultured monolayers of neonatal rat ventricular myocytes. Circ Res. 79: 676–690.
- Gollob MH, Jones DL, Krahn AD, Danis L, Gong XQ, Shao Q, Liu X, Veinot JP, Tang AS, Stewart AF, Tesson F, Klein GJ, Yee R, Skanes AC, Guiraudon GM, Ebihara L, Bai D (2006). Somatic mutations in the connexin 40 gene (GJA5) in atrial fibrillation. N Engl J Med. 354: 2677–2688.
- Gu H, Smith FC, Taffet SM, Delmar M (2003). High incidence of cardiac malformations in connexin40-deficient mice. Circ Res. 93: 201–206.
- Gutstein DE, Morley GE, Tamaddon H, Vaidya D, Schneider MD, Chen J, Chien KR, Stuhlmann H, Fishman GI (2001). Conduction slowing and sudden arrhythmic death in mice with cardiac-restricted inactivation of connexin43. Circ Res. 88: 333–339.
- Harris AL (2001). Emerging issues of connexin channels: biophysics fills the gap. Q Rev Biophys. 34: 325–472.
- Hirschy A, Schatzmann F, Ehler E, Perriard JC (2006). Establishment of cardiac cytoarchitecture in the developing mouse heart. Develop Biol. 289: 430–441.
- Ho CY, López B, Coelho-Filho OR, Lakdawala NK, Cirino AL, Jarolim P, Kwong R, González A, Colan SD, Seidman JG, Díez J, Seidman CE (2010). Myocardial fibrosis as an early manifestation of hypertrophic cardiomyopathy. N Engl J Med. 363: 552–563.
- Hoyt RH, Cohen ML, Saffitz JE (1989). Distribution and three-dimensional structure of intercellular junctions in canine myocardium. Circ Res. 64: 563–574.
- Jacot JG, McCulloch AD, Omens JH (2008). Substrate stiffness affects the functional maturation of neonatal rat ventricular myocytes. Biophys J. 95: 3479–3487.
- Kanagaratnam P, Rothery S, Patel P, Severs NJ, Peters NS (2002). Relative expression of immunolocalized connexins 40 and 43 correlates with human atrial conduction properties. J Am Coll Cardiol. 39: 116–123.
- Kleber AG, Janse MJ, Fast VG (2003). Normal and abnomal conduction in the heart. In: Page E, Fozzard H, Solaro R (eds). The Handbook of Physiology. New York: Oxford University Press, pp. 455–530.
- Kleber AG, Riegger CB (1987). Electrical constants of arterially perfused rabbit papillary muscle. J Physiol. 385: 307–324.
- Kleber AG, Rudy Y (2004). Basic mechanisms of cardiac impulse propagation and associated arrhythmias. Physiol Rev. 84: 431–488.
- Kucera JP, Kléber AG, Rohr S (1998). Slow conduction in cardiac tissue, II: effects of branching tissue geometry. Circ Res. 83: 795–805.
- Kucera JP, Kléber AG, Rohr S (2001). Slow conduction in cardiac tissue: insights from optical mapping at the cellular level. J Electrocardiol. 34: 57–64.
- Kucera JP, Rohr S, Rudy Y (2002). Localization of sodium channels in intercalated disks modulates cardiac conduction. Circ Res. 91: 1176–1182.
- Kuo PL, Lee H, Bray MA, Geisse NA, Huang YT, Adams WJ, Sheehy SP, Parker KK (2012). Myocyte shape regulates lateral registry of sarcomeres and contractility. Am J Pathol. 181: 2030–2037.
- le Duc Q, Shi Q, Blonk I, Sonnenberg A, Wang N, Leckband D, de Rooij J (2010). Vinculin potentiates E-cadherin mechanosensing and is recruited to actin-anchored sites within adherens junctions in a myosin II-dependent manner. J Cell Biol. 189: 1107–1115.
- Li J, Radice GL (2010). A new perspective on intercalated disc organization: implications for heart disease. Dermatol Res Pract. 2010: 207835.
- Lieberman M, Sawanobori T, Kootsey JM, Johnson EA (1975). A synthetic strand of cardiac muscle: its passive electrical properties. J Gen Physiol. 65: 527–550.
- Lieberman M, Roggeveen AE, Purdy JE, Johnson EA (1972). Synthetic strands of cardiac muscle: growth and physiological implication. Science. 175: 909–911.
- Liu Z, Tan JL, Cohen DM, Yang MT, Sniadecki NJ, Ruiz SA, Nelson CM, Chen CS (2010). Mechanical tugging force regulates the size of cell-cell junctions. Proc Natl Acad Sci U S A. 107: 9944–9949.
- Maier SK, Westenbroek RE, McCormick KA, Curtis R, Scheuer T, Catterall WA (2004). Distinct subcellular localization of different sodium channel alpha and beta subunits in single ventricular myocytes from mouse heart. Circulation. 109: 1421–1427.
- Majkut S, Idema T, Swift J, Krieger C, Liu A, Discher DE (2013). Heart-specific stiffening in early embryos parallels matrix and myosin expression to optimize beating. Curr Biol. 23: 2434–2439.
- Martinez AD, Hayrapetyan V, Moreno AP, Beyer EC (2002). Connexin43 and connexin45 form heteromeric gap junction channels in which individual components determine permeability and regulation. Circ Res. 90: 1100–1107.
- Maruthamuthu V, Sabass B, Schwarz US, Gardel ML (2011). Cell-ECM traction force modulates endogenous tension at cell-cell contacts. Proc Natl Acad Sci U S A. 108: 4708–4713.
- Matsushita T, Oyamada M, Fujimoto K, Yasuda Y, Masuda S, Wada Y, Oka T, Takamatsu T (1999). Remodeling of cell-cell and cell-extracellular matrix interactions at the border zone of rat myocardial infarcts. Circ Res. 85: 1046–1055.
- McCain ML, Desplantez T, Geisse NA, Rothen-Rutishauser B, Oberer H, Parker KK, Kleber AG (2012b). Cell-to-cell coupling in engineered pairs of rat ventricular cardiomyocytes: relation between Cx43 immunofluorescence and intercellular electrical conductance. Am J Physiol Heart Circ Physiol. 302: H443–H450.
- McCain ML, Lee H, Aratyn-Schaus Y, Kléber AG, Parker KK (2012a). Cooperative coupling of cell-matrix and cell-cell adhesions in cardiac muscle. Proc Natl Acad Sci U S A. 109: 9881–9886.
- McCain ML, Parker KK (2011). Mechanotransduction: the role of mechanical stress, myocyte shape, and cytoskeletal architecture on cardiac function. Pflugers Arch. 462: 89–104.
- Milstein ML, Musa H, Balbuena DP, Anumonwo JM, Auerbach DS, Furspan PB, Hou L, Hu B, Schumacher SM, Vaidyanathan R, Martens JR, Jalife J (2012). Dynamic reciprocity of sodium and potassium channel expression in a macromolecular complex controls cardiac excitability and arrhythmia. Proc Natl Acad Sci U S A. 109: E2134–E2143.
- Moreno AP, Laing JG, Beyer EC, Spray DC (1995). Properties of gap junction channels formed of connexin 45 endogenously expressed in human hepatoma (SKHep1) cells. Am J Physiol. 268: C356–C365.
- Mori Y, Fishman GI, Peskin CS (2008). Ephaptic conduction in a cardiac strand model with 3D electrodiffusion. Proc Natl Acad Sci U S A. 105: 6463–6468.
- Noorman M, Hakim S, Kessler E, Groeneweg JA, Cox MG, Asimaki A, van Rijen HV, van Stuijvenberg L, Chkourko H, van der Heyden MA, Vos MA, de Jonge N, van der Smagt JJ, Dooijes D, Vink A, de Weger RA, Varro A, de Bakker JM, Saffitz JE, Hund TJ, Mohler PJ, Delmar M, Hauer RN, van Veen TA (2013). Remodeling of the cardiac sodium channel, connexin43, and plakoglobin at the intercalated disk in patients with arrhythmogenic cardiomyopathy. Heart Rhythm. 10: 412–419.
- Okabe M, Ikawa M, Kominami K, Nakanishi T, Nishimune Y (1997). ‘Green mice’ as a source of ubiquitous green cells. FEBS Lett. 407: 313–319.
- Oxford EM, Everitt M, Coombs W, Fox PR, Kraus M, Gelzer AR, Saffitz J, Taffet SM, Moïse NS, Delmar M (2007). Molecular composition of the intercalated disc in a spontaneous canine animal model of arrhythmogenic right ventricular dysplasia/cardiomyopathy. Heart Rhythm. 4: 1196–1205.
- Pedrotty DM, Klinger RY, Badie N, Hinds S, Kardashian A, Bursac N (2008). Structural coupling of cardiomyocytes and noncardiomyocytes: quantitative comparisons using a novel micropatterned cell pair assay. Am J Physiol Heart Circ Physiol. 295: H390–H400.
- Peters NS, Coromilas J, Severs NJ, Wit AL (1997). Disturbed connexin43 gap junction distribution correlates with the location of reentrant circuits in the epicardial border zone of healing canine infarcts that cause ventricular tachycardia. Circulation. 95: 988–996.
- Peters NS, Severs NJ, Rothery SM, Lincoln C, Yacoub MH, Green CR (1994). Spatiotemporal relation between gap junctions and fascia adherens junctions during postnatal development of human ventricular myocardium. Circulation. 90: 713–725.
- Petitprez S, Zmoos AF, Ogrodnik J, Balse E, Raad N, El-Haou S, Albesa M, Bittihn P, Luther S, Lehnart SE, Hatem SN, Coulombe A, Abriel H (2011). SAP97 and dystrophin macromolecular complexes determine two pools of cardiac sodium channels Nav1.5 in cardiomyocytes. Circ Res. 108: 294–304.
- Rohr S, Kucera JP, Fast VG, Kléber AG (1997). Paradoxical improvement of impulse conduction in cardiac tissue by partial cellular uncoupling. Science. 275: 841–844.
- Rohr S, Kucera JP, Kléber AG (1998). Slow conduction in cardiac tissue, I: effects of a reduction of excitability versus a reduction of electrical coupling on microconduction. Circ Res. 83: 781–794.
- Rohr S, Schölly DM, Kléber AG (1991). Patterned growth of neonatal rat heart cells in culture. Morphological and electrophysiological characterization. Circ Res. 68: 114–130.
- Salameh A, Wustmann A, Karl S, Blanke K, Apel D, Rojas-Gomez D, Franke H, Mohr FW, Janousek J, Dhein S (2010). Cyclic mechanical stretch induces cardiomyocyte orientation and polarization of the gap junction protein connexin43. Circ Res. 106: 1592–1602.
- Sato PY, Musa H, Coombs W, Guerrero-Serna G, Patiño GA, Taffet SM, Isom LL, Delmar M (2009). Loss of plakophilin-2 expression leads to decreased sodium current and slower conduction velocity in cultured cardiac myocytes. Circ Res. 105: 523–526.
- Schiller HB, Fassler R (2013). Mechanosensitivity and compositional dynamics of cell-matrix adhesions. EMBO Rep. 14: 509–519.
- Sepp R, Severs NJ, Gourdie RG (1996). Altered patterns of cardiac intercellular junction distribution in hypertrophic cardiomyopathy. Heart. 76: 412–417.
- Severs NJ, Bruce AF, Dupont E, Rothery S (2008). Remodelling of gap junctions and connexin expression in diseased myocardium. Cardiovasc Res. 80: 9–19.
- Singhvi R, Kumar A, Lopez GP, Stephanopoulos GN, Wang DI, Whitesides GM, Ingber DE (1994). Engineering cell shape and function. Science. 264: 696–698.
- Sniadecki NJ, Chen CS (2007). Microfabricated silicone elastomeric post arrays for measuring traction forces of adherent cells. Methods Cell Biol. 83: 313–328.
- Thomas SP, Kucera JP, Bircher-Lehmann L, Rudy Y, Saffitz JE, Kléber AG (2003). Impulse propagation in synthetic strands of neonatal cardiac myocytes with genetically reduced levels of connexin43. Circ Res. 92: 1209–1216.
- Twiss F, de Rooij J (2013). Cadherin mechanotransduction in tissue remodeling. Cell Mol Life Sci. 70: 4101–4116.
- Valiunas V, Gemel J, Brink PR, Beyer EC (2001). Gap junction channels formed by coexpressed connexin40 and connexin43. Am J Physiol Heart Circ Physiol. 281: H1675–H1689.
- Valiunas V, Polosina YY, Miller H, Potapova IA, Valiuniene L, Doronin S, Mathias RT, Robinson RB, Rosen MR, Cohen IS, Brink PR (2005). Connexin-specific cell-to-cell transfer of short interfering RNA by gap junctions. J Physiol. 568: 459–468.
- Vozzi C, Dupont E, Coppen SR, Yeh HI, Severs NJ (1999). Chamber-related differences in connexin expression in the human heart. J Mol Cell Cardiol. 31: 991–1003.
- Weber GF, Bjerke MA, DeSimone DW (2011). Integrins and cadherins join forces to form adhesive networks. J Cell Sci. 124: 1183–1193.
- Weidmann S (1966). The diffusion of radiopotassium across intercalated disks of mammalian cardiac muscle. J Physiol. 187: 323–342.
- Wu JC, Sung HC, Chung TH, DePhilip RM (2002). Role of N-cadherin- and integrin-based costameres in the development of rat cardiomyocytes. J Cell Biochem. 84: 717–724.
- Yamada K, Green KG, Samarel AM, Saffitz JE (2005). Distinct pathways regulate expression of cardiac electrical and mechanical junction proteins in response to stretch. Circ Res. 97: 346–353.
- Zhang SS, Hong S, Kléber AG, Lee LP, Shaw RM (2014). A micropatterning approach for imaging dynamic Cx43 trafficking to cell-cell borders. FEBS Lett. pii: S0014-5793(14)00016-7. pub ahead of print.
- Zhang X, Wang Q, Gablaski B, Zhang X, Lucchesi P, Zhao Y (2013). A microdevice for studying intercellular electromechanical transduction in adult cardiac myocytes. Lab chip. 13: 3090–3097.
- Zhou J, Shu Y, Lü SH, Li JJ, Sun HY, Tang RY, Duan CM, Wang Y, Lin QX, Mou YC, Li X, Wang CY (2013). The spatiotemporal development of intercalated disk in three-dimensional engineered heart tissues based on collagen/matrigel matrix. PloS one. 8: e81420.
- Zhuang J, Yamada KA, Saffitz JE, Kléber AG (2000). Pulsatile stretch remodels cell-to-cell communication in cultured myocytes. Circ Res. 87: 316–322.