Overview summary of ‘Measuring immune responses in non-human primates for drug development—Opportunities and challenges for predicting human efficacy and immunotoxicity’
Cris Kamperschroer
Immunotoxicology Center of Emphasis, Drug Safety Research and Development, Pfizer, Inc., Eastern Point Road, Groton, CT 06340, USA
Non-human primates (NHP) are important pre-clinical toxicology models, particularly in cases where therapeutics such as monoclonal antibodies (mAbs) only cross-react with NHP. Therapeutics can be toxic to the immune system because of unexpected effects or because of exaggerated pharmacology of those intended to enhance or suppress immune responses in order to treat immune-related diseases. To adequately assess the risk of potentially immunotoxic drugs in NHP, we need more advanced tools to measure their effects on the immune system. In March 2010, on the 25th Anniversary of the Immunotoxicology Specialty Section of the Society of Toxicology (SOT), several methods for measuring immune responses in NHP were presented and discussed at the annual SOT meeting in Salt Lake City. The session was co-chaired by Cris Kamperschroer and Herve Lebrec, who also gave presentations. JoAnne Flynn and Amitinder Kaur also gave presentations on methods they use for measuring NHP immune responses to important human pathogens. The presentations covered issues with more established methods as well as methods that are generally not used in pre-clinical development.
Dr Cris Kamperschroer from Pfizer, Inc. provided a brief introduction to the session. The introduction covered some of the pros and cons of using NHP as test species for drug development and stressed the need for more advanced tools to measure immune responses in NHP. He then spoke about the idea of exploiting immune responses against persistent viral infections as a tool for monitoring immune function. He described ELISPOT and other methods for measuring T-cell responses against persistent viruses like cytomegalovirus (CMV) and lymphocryptovirus (LCV) in cynomolgus macaques. Both CMV and LCV can be pathogenic to NHP or humans under immunosuppressive conditions, and immunosuppression is common with therapeutics for transplant, autoimmune conditions, or other inflammatory diseases. As a result, this approach may be useful for identifying reduced immunity to CMV or LCV, which may reflect the potential for CMV or LCV-associated disease. Although it is in the early stages, the approach may also provide a way to monitor overall adaptive immune function in NHPs without the need for intentional induction of immune responses by vaccination. The rationale, basic methodology, and potential utility of this approach are reviewed by Dr Kamperschroer in this article.
Tuberculosis is a major health problem worldwide, and reactivation of latent infection with the causative agent, Mycobacterium tuberculosis can be a concern during immunosuppression. Dr JoAnne Flynn from the University of Pittsburgh introduced a cynomolgus macaque model for studying tuberculosis and discussed the utility of this model for studying latency. The talk covered the components of the immune system important for control of the bacterium during latency, the process of reactivation of M. tuberculosis from latency, and factors that correlate with reactivation (targeted immune suppression, etc.). In addition to more conventional methods for measuring M. tuberculosis load and M. tuberculosis-specific T-cell responses, she introduced a PET-/CT-based method for visualizing M. tuberculosis-associated lesions in the lungs of NHPs over time, including those with latent infection as well as those showing reactivation. This is based on the detection of a fluoro-deoxyglucose (FDG) tracer to identify foci of metabolically active cells, as occurs in active lung lesions. This technique may prove to be a powerful tool for monitoring the status of lung infection and possibly even for predicting whether an individual will reactivate infection. Studies involving this methodology are expensive and can currently be done only in a small number of specialized laboratories. However, when used along with other end-points, the methodology may provide an option for testing whether an immunomodulatory drug candidate has the risk of M. tuberculosis reactivation.
Dr. Amitinder Kaur from the New England Primate Research Center associated with Harvard University presented her recent studies aimed at understanding the mechanisms of immunity to CMV and other persistent viruses in rhesus macaques. She briefly reviewed CMV biology and then discussed various ways of measuring antiviral immune responses against CMV. She focused mainly on ELISPOT and intracellular cytokine staining (ICS) approaches for measuring T-cell responses, and highlighted pros and cons of each. She covered methodological considerations for each technique and discussed measuring polyfunctional multi-cytokine secreting T-cells by ICS, as polyfunctional T-cells are thought to be more effective in controlling viral infection. Finally, she discussed the negative impact of immunosuppression via simian immunodeficiency virus (SIV) infection on CMV. Her data showed that SIV immunosuppression decreased neutralizing antibody against CMV and decreased T-cell responses to CMV, and that this immunosuppressive state correlated with a loss of control over CMV as measured by increased CMV viremia. Dr Kaur covers this information in detail later in this article. These studies reiterate the utility of methods such as ELISPOT and ICS for measuring T-cell responses against specific antigens, and show that responses measured by such methods can be sensitive to immunosuppression. These methods may, therefore, be useful in the setting of drug development to test the effects of therapeutics on T-cell functions.
Both immunophenotyping and the T-dependent antibody response (TDAR) assay are often used for pre-clinical development and are commonly accepted methods for assessing toxicity to the immune system (CPMP, Citation2000; FDA, Citation2002; ICH, Citation2006). Dr Herve Lebrec presented work describing a large-scale retrospective statistical analysis undertaken by various members of the Immunotoxicology Technical Committee of ILSI-HESI to evaluate the TDAR and immunophenotyping in NHP. Various factors that may influence the design, performance, and interpretation of NHP studies were analyzed using data from both immunophenotyping and TDAR assays. He described some of the initial findings with regards to how certain parameters affect the results as well as how variability observed in data sets may inform future study designs. This large scale analysis should prove useful in the future when planning and executing NHP studies involving the TDAR or immunophenotyping as end-points.
The session provided information about methods currently being used to measure immune responses in NHP, some of which are not typically used in drug development. Hopefully, the discussions will spark further investigation into such methods, or even into developing entirely novel methods. The hope is that this will result in better tools for evaluating immunotoxicity in NHP.
Declaration of interest
The author reports no conflicts of interest. The author alone is responsible for this segment of the publication.
Exploiting viruses to measure effects of immunomodulatory drugs on T-cell responses in non-human primates
Cris Kamperschroer
Immunotoxicology Center of Emphasis, Drug Safety Research and Development, Pfizer, Inc., Eastern Point Road, Groton, CT 06340, USA
Address for Correspondence: Cris Kamperschroer, Immunotoxicology Center of Emphasis, Drug Safety Research and Development, Pfizer, Inc., Eastern Point Road, Groton, CT 06340, USA. Tel: 860 686-1393; E-mail: [email protected]
Introduction
Immunomodulatory drugs inherently carry the risk of undesirable effects on the immune system due to exaggerated or unwanted pharmacology. Indeed, a number of immunomodulatory drugs have increased the risk of unwanted consequences, which include an increased risk of infections (Kotton and Fishman, Citation2005; Berger and Houff, Citation2009; Furst, Citation2010; Johnson et al., Citation2010), an increased risk of tumor formation (Bugelski et al., Citation2010; Krathen et al., Citation2010), or in the case of immune enhancement, adverse events related to overactive T-cell responses (e.g., cytokine-driven adverse events) (Suntharalingam et al., Citation2006). T-cells are the targets of a number of immunomodulatory drugs—some drugs are designed to enhance responses against tumors or infections and others are designed suppress (or alter) T-cells involved in disease states with an autoimmune component, like rheumatoid arthritis, colitis/inflammatory bowel disease (IBD), multiple sclerosis (MS), and psoriasis. As we develop immunomodulatory drugs with greater target specificity and more diverse mechanisms of action, we will require a more sophisticated understanding of how those drugs affect T-cells so that we can best evaluate the safety (or pharmacology) of candidate drugs.
As a result, it could be argued that it is worth the investment to develop new methods or adapt more sophisticated methods to the drug development setting for the purpose of evaluating safety. Our group has recently been working to enhance our repertoire of tools to measure immune responses in NHP. This brief review focuses on measuring T-cell responses in NHP and highlights recent efforts by our laboratory to develop methodology for monitoring T-cell responses against viruses in cynomolgus monkeys.
Methods for measuring T-cell responses in NHP
There are various ways to measure responses of T-cells in NHP, and this section gives a brief overview of the different methods available. One of the traditional methods for measuring T-cell responses is the delayed type hypersensitivity (DTH) reaction, and it has been used to test immunomodulatory drugs in NHP (Imamura et al., Citation1993; Schmitz et al., Citation1999; Yuzawa et al., Citation2003). The DTH assay has the advantages of being simple to perform and of being done in vivo (e.g., added to a toxicology study), but has the disadvantage of relying on a fairly crude end-point—local swelling. As a result, the assay can be highly variable, although there are efforts underway to reduce this issue by including the evaluation of cell infiltration by histology (Cordoba et al., Citation2008). Also, other cell types besides T-cells are involved in the DTH reaction, so it is not necessarily a direct measure of T-cell function.
Other traditional methods of measuring T-cell responses involve stimulating cells in vitro or ex vivo (from treated animals) with concanavalin A (ConA), phytohemagglutinin (PHA), phorbol myristate acetate (PMA), and ionomycin, or other polyclonal activators, and then measuring proliferation or cytokine production from bulk cultures (Dumont et al., Citation1990; Lebrec et al., Citation1995; Barten et al., Citation2003; Carfi et al., Citation2007). These assays stimulate a large proportion of T-cells and the responses are easy to detect. The assays are also simple to perform and the reagents for stimulating T-cells are relatively inexpensive. However, these assays generally cannot provide detail about the number of responders or phenotypic information about the responding T-cells, nor can they be used to measure T-cell responses against specific foreign antigens.
Today, in the field of NHP immunology, the most commonly used techniques are single cell assays to detect T-cells directly or via specific functions. One of these, the ELISPOT assay, measures single T-cells secreting factors in response to antigen or other stimulus (Schmittel et al., Citation2000; Kumar et al., Citation2001; Kaur et al., Citation2002; Fogg et al., Citation2005). The factors most commonly measured are cytokines, but proteins released from degranulating cytotoxic T-cells can also be detected using ELISPOT. The advantage to the ELISPOT is that it is highly sensitive and can detect low frequencies of responding T-cells (McCutcheon et al., Citation1997; Kaur et al., Citation2002).
Flow cytometry is also commonly used to detect responsive T-cells. As for ELISPOT, cytokines (detected by intracellular cytokine staining [ICS]) or other molecules produced by responding T-cells are used as readouts. The major advantage to flow cytometry is the ability to simultaneously detect multiple functions and to obtain phenotypic information about responding T-cells (Chan and Kaur, Citation2007; Seder et al., Citation2008; Bolton and Roederer, Citation2009). A large amount of information can be obtained using standard fluorochrome-conjugated antibodies/reagents, allowing various sub-populations of T-cells to be identified, and throughput can be greatly increased by ‘bar coding’ samples with different amounts of various cell dyes (Krutzik and Nolan, Citation2006; Krutzik et al., Citation2011). Using tetrameric or other multimeric major histocompatibility complex (MHC)-peptide reagents, it is possible to detect individual T-cells of a defined antigenic specificity without relying on any function of the cell (Altman et al., Citation1996; Vollers and Stern, Citation2008; Hadrup and Schumacher, Citation2010). This can be useful for enumerating antigen-specific T-cells, or, when used along with functional end-points, for understanding whether T-cells are functional. Recent advances in flow cytometry using novel metal tags and mass spectrometry have allowed for better resolution of individual staining reagents and multiplexing of very large numbers of analytes, making it possible to generate even more phenotypic information on individual cells in a given sample (Ornatsky et al., Citation2010; Bendall et al., Citation2011). Other approaches available for detecting T-cell responses include the use of CD40 ligand and 4-1BB to detect (or isolate) CD4 or CD8 T-cells responding to antigen (Chattopadhyay et al., Citation2005; Cohen et al., Citation2005; Frentsch et al., Citation2005; Wolfl et al., Citation2008), and imaging approaches like PET-CT (Radu et al., Citation2008; Ribas et al., Citation2010; Singh et al., Citation2010).
For specifically measuring cytotoxic T-lymphocytes (CTL), there are a variety of assays that can be done in vitro or ex vivo, or even in vivo. These include the chromium release assay or its various non-radioactive alternatives, ELISPOT to detect perforin or granzyme B, or flow cytometry analysis of markers of degranulation like CD107a (Zaritskaya et al., Citation2010).
The above methods are very often used to detect T-cell responses against defined antigens instead of mitogens or polyclonal stimulators. Defined antigens better reflect true biology, they allow one to evaluate effects of drugs on T-cell immunity against specific pathogens of concern, and they may be more sensitive to immunosuppression than some mitogens (unpublished observations). For these reasons, it is worth exploring the use of antigen-specific responses, but they have not typically been used for evaluating immunotoxicity because: (1) the numbers of T-cells specific to a given antigen are relatively small and harder to detect; (2) a source of antigen is not always readily available; (3) immunization would usually be necessary to generate responses against an antigen of interest; and (4) not all animals have detectable T-cell responses to a given protein or peptide. Despite these hurdles, measuring antigen-specific T-cell responses may prove useful for evaluation of immunotoxicity, especially in the setting of immunomodulatory drug development.
Rationale for measuring immune responses against viruses
Our laboratory has recently explored the idea of using viruses as tools for monitoring T-cell responses in NHP. Why use viruses as antigens to monitor T-cell responses? There are several possible explanations: (1) Persistent viruses like cytomegalovirus (CMV) and lymphocryptovirus (LCV) infect most NHP early in life (Vogel et al., Citation1994; Carville and Mansfield, Citation2008); (2) After infection, persistent viruses are present for the remainder of the lifetime of the host; likewise, the T-cell responses that control them are always present; (3) The frequencies of T-cells specific for some persistent viruses like CMV are very high compared to many other infectious agents, allowing for the detection of responses without in vitro stimulation/expansion; (4) Measurements could be done from standard toxicity studies without the need for additional immunization; and (5) In humans, persistent viruses like CMV and Epstein-Barr virus (EBV; homologous to LCV in NHPs) are important opportunistic pathogens that cause disease in the context of treatment with immunomodulatory drugs.
The NHP homologues of EBV and CMV are very similar to their human counterparts in terms of genetic similarity, biology, and pathogenesis (Moghaddam et al., Citation1997; Rivailler et al., Citation2004; Carville and Mansfield, Citation2008). These characteristics make CMV and LCV attractive antigens for tracking T-cell responses and we have focused mostly on these viruses, although T-cell responses to other persistent viruses or viruses that NHP are routinely vaccinated against could also be measured.
Assays used in our laboratory to measure T-cell responses against viruses
Antigen source
For measuring T-cell responses against viruses, our laboratory has primarily used lysates of cells infected with the virus of interest. CMV lysate was purchased and is derived from infected fibroblasts as described elsewhere (Pitcher et al., Citation2002). The CMV is an isolate from rhesus macaques, but is sufficiently homologous to stimulate CMV-specific T-cells in cynomolgus macaques ( and unpublished observations), which are more commonly used for toxicology studies. To generate a source of LCV antigen, peripheral blood mononuclear cells (PBMC) from cynomolgus monkeys were cultured long-term in the presence of cyclosporine to suppress T-cell control over LCV-infected cells and allow for the outgrowth of LCV infected lymphoblastic cell lines (LCLs). The LCLs were characterized and then used to prepare lysates containing LCV antigen. Cell lysates can vary in potency from batch to batch and contain cellular components as well as the desired viral antigen, so we have titrated each batch for potency and specificity. Lysates may also introduce a bias towards CD4 T-cell responses due to the need for less efficient cross-presentation to stimulate CD8 T-cells, so they may not be optimal when CD8 T-cell responses are the primary interest. For these situations, we have also pursued using peptides or peptide pools as antigens to detect T-cell responses.
Figure 1. IFNγ ELISPOT assay for measuring T-cell responses to CMV or LCV. To measure T-cell responses against CMV or LCV, peripheral blood mononuclear cells (PBMC) are isolated from whole blood of cynomolgus macaques (from Mauritius) using CPT tubes. PBMC are added to ELISPOT plates pre-coated with anti-IFNγ capture antibody and stimulated with cell lysates containing viral antigen. Lysates of CMV-infected fibroblasts were purchased (note: CMV is derived from rhesus macaques) and lysates of LCV-infected lymphoblastic cell lines generated in house. After ~ 48 h, plates are washed and then spots are visualized using an enzyme-secondary antibody and an insoluble colorimetric enzyme substrate. Spots represent individual T-cells secreting IFNγ in response to the stimulus, and the data is expressed as the number of spot forming cells per total number of input PBMC. The example shown indicates clear T-cell responses against both viruses directly ex vivo, and is representative of the results typically observed from untreated cynomolgus macaques.
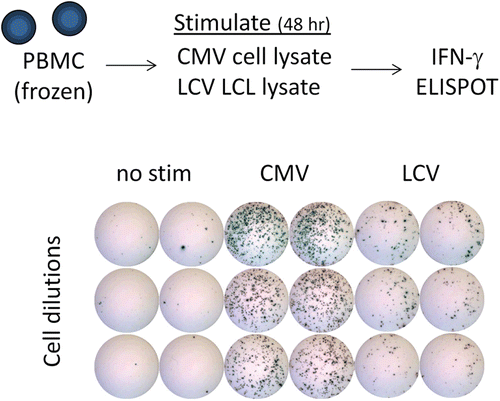
Assay formats
As discussed above, many assay formats are available for measuring T-cell responses. For our initial work, we chose to focus on the ELISPOT assay because of its sensitivity and relative ease to perform, and we measured interferon (IFN)-γ as it is produced by NHP T-cells upon recognition of most viruses, including LCV and CMV (Kaur et al., Citation2002; Fogg et al., Citation2005). Our lab has spent some time optimizing a variety of conditions for the IFNγ ELISPOT to detect T-cell responses to CMV and LCV. This work is currently being prepared for a manuscript submission and will not be detailed here; however, an example of T-cell responses against CMV and LCV measured by IFNγ ELISPOT from cynomolgus macaques is shown in . Our results have indicated that nearly all NHPs in our colony have detectable T-cell responses against both viruses. Larger numbers of T-cells respond to CMV than to LCV (), and the numbers of responding cells we have observed is in a similar range to what has been reported in the literature on human or rhesus macaque CMV or EBV/LCV-specific T-cells (Kaur et al., Citation2002; Fogg et al., Citation2005; Hislop et al., Citation2007). This work has provided a sensitive tool for monitoring T-cell responses against these opportunistic viruses.
We have also been using flow cytometry to detect intracellular cytokine staining (ICS) as a method for measuring antiviral T-cell responses. An example of the detection of CMV-specific and LCV-specific T-cells from cynomolgus monkeys is shown in . We have been able to detect T-cell responses against both CMV and LCV using ICS, and the frequency of responders by ICS is similar to that seen in ELISPOT. We have generally detected a larger number of cells producing tumor necrosis factor (TNF)-α than IFNγ. This has been observed previously for macaques (Kaur et al., Citation2002), and may reflect limitations of the cross-reactive anti-human IFNγ antibodies used for staining. Responses against LCV come from both CD4 T-cells and CD8 T-cells, whereas responses against CMV are almost exclusively from CD4 T-cells, at least with lysate as antigen (). Other forms of antigen will be needed to measure CD8 T-cell responses against CMV. As mentioned above, with flow cytometry we can collect additional phenotypic information about the responding cells with relative ease. This could be valuable in situations where drug effects on specific functions or sub-populations of T-cells are a concern.
Figure 2. Intracellular cytokine staining and flow cytometry for measuring T-cell responses to CMV or LCV. To detect T-cell responses by flow cytometry, PBMC from cynomolgus macaques are stimulated with lysates from either CMV- or LCV-infected cells, as described in and in the text. However, cells are stimulated in the presence of brefeldin A to block cytokine secretion. After 6 h of stimulation, cells are stained with antibodies against desired surface antigens. Cells are subsequently fixed, permeabilized, and stained with antibodies against cytokines such as IFNγ and TNFα. Cells are analyzed using a multi-parameter flow cytometer. A representative example of data obtained from untreated cynomolgus macaques is shown. Stimuli are shown at the top of each set of plots (no stim = no stimulant added) and parameters analyzed are shown with axis labels. (A) Responses of CD4 T-cells (CD3+CD4+CD8−). (B) Responses of CD8 T-cells (CD3+CD8+CD4−).
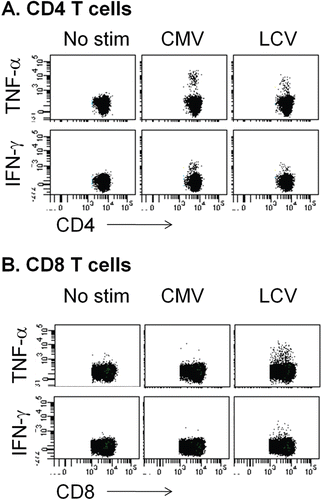
A final format we have explored for measuring virus-specific T-cell responses is a perforin ELISA assay. Perforin can serve as a surrogate marker for cytolytic T-lymphocyte (CTL) function because it is a component of cytotoxic granules and can be detected in vitro in supernatant upon degranulation of CTL from macaques (Zuber et al., Citation2006). We have done less characterization of this assay for measuring CMV- or LCV-specific CTL responses, but we have been able to detect Hepatitis B virus-specific CTL responses from Hepatitis B vaccinated and not from unvaccinated cynomolgus monkeys (unpublished results), suggesting that the assay may be useful for monitoring CTL activity.
Mauritian cynomolgus macaques as the model system
We are interested in measuring NHP T-cell responses against defined peptide epitopes (see section on antigen source). Defined peptides restricted by known MHC alleles would be a more consistent source of antigen and would also allow us to generate tools such as MHC-peptide multimers for detecting antigen-specific T-cells or artificial antigen presenting cells (aAPC) for stimulation of antigen-specific CD8 T-cells (Oelke et al., Citation2003; Oelke and Schneck, Citation2010). Cynomolgus macaques from the island of Mauritius may allow us to use such tools because there are as few as seven MHC haplotypes in the entire population (O’Connor et al., Citation2007; Wiseman et al., Citation2007; Florese et al., Citation2008; Burwitz et al., Citation2009; Budde et al., Citation2010). As a result, a small panel of defined epitopes within CMV or LCV could conceivably cover the entire population such that every individual would show T-cell responses. This would not be possible using more diverse populations of cynomolgus macaques with extensive MHC polymorphism. Using defined T-cell epitopes in Mauritian cynomolgus macaques may allow for the development of powerful tools for probing effects on antiviral T-cell responses.
Looking ahead to applying assays for detecting virus-specific T-cells
Effects of immunomodulators
It will be important to understand whether the assays we have developed are sensitive to effects of immunomodulatory therapeutics. Preliminary in vitro data are encouraging (unpublished results), and studies are underway to test whether our assays can detect the effects of immunosuppressive drugs in NHP.
Application to drug development
Will the approach of monitoring virus-specific T-cells be generally useful for detecting and/or managing T-cell immunotoxicity? Developing these more specialized methods requires an investment of time and resources and it is possible that the data will show that, at least for general use, more traditional methods are just as effective for addressing our concerns. On the other hand, the assays may turn out to be broadly useful for determining effects of drugs on T-cells for the reasons outlined above. We cannot find out unless we explore the idea by developing the methods, applying them to a range of immunomodulators, and ultimately correlating non-clinical results with clinical outcomes.
Irrespective of broad use, we anticipate that the methods we developed for measuring virus-specific T-cells in cynomolgus monkeys will enable us to better understand safety issues associated with CMV and LCV as well as related viruses with similar mechanisms of immune control. Work currently underway should provide proof-of-principle data on how sensitive the T-cell assays are when used on cells from drug-treated NHP. This and future work should lead to better tools for assessing safety of immunomodulatory drugs.
Acknowledgments
The author acknowledges Lynn M. O’Donnell and Patricia A. Schneider for performing the work in the laboratory that is described in this review, Thomas T. Kawabata for helpful discussion, and Ellen Evans for reviewing the manuscript.
Declaration of interest
The author reports no conflicts of interest. The author alone is responsible for this segment of the publication.
T-dependent antibody responses and immunophenotyping in cynomolgus monkeys—Impact on study design and interpretation
Herve Lebrec
Amgen Inc., Seattle, WA, USA
Address for Correspondence: Dr Hervé Lebrec, Amgen Inc., 1201 Amgen Ct. West, AW1-J4144, Seattle WA 98119-3105, USA. Tel: 206–265-7614; Fax: 206–216-5930; E-mail: [email protected]
The Immunotoxicology Technical Committee (ITC) of the International Life Sciences Institute (ILSI) Health and Environmental Sciences Institute (HESI) sponsored retrospective analyses of both the T-cell-dependent antibody response and peripheral blood immunophenotyping in non-human primates (NHP).
The T-cell-dependent antibody response (TDAR) to T-cell-dependent antigens is a functional assay used in immunopharmacology and immunotoxicology to assess the ability of the species of interest to mount a specific antibody (IgM and/or IgG) response to immunization (Luster et al., Citation1992, Citation1993; reviewed by Piccotti, Citation2008). In toxicology regulatory guidance documents, the TDAR is considered as one of the functional assays of choice to evaluate potential immunotoxicity of investigational new drugs (CPMP, Citation2000; FDA, Citation2002; ICH, Citation2006). While this assay is extensively used in rodents, the significant increase in the number of biopharmaceuticals being developed and lacking cross-reactivity in rodents has been associated with a need for non-human primate immunotoxicology models.
Studies have been dedicated to the optimization of the immunization protocols for conducting a TDAR assay in non-human primates (Piccotti et al., Citation2005; Caldwell et al., Citation2007; Haggerty, Citation2007; Kirk et al., Citation2008; Tichenor et al., Citation2010). However, the multiple variables potentially associated with such studies render the optimization of such protocols challenging. In that context, the ITC of the ILSI-HESI sponsored a retrospective inter-laboratory study of TDAR in NHP. The TDAR responses to keyhole limpet hemocyanin (KLH), tetanus toxoid (TT), and/or sheep red blood cells (SRBC) in 178 NHP (cynomolgus monkeys from eight sponsors, 13 testing sites, and 30 studies) were statistically analyzed. Rates of positive or negative anti-KLH, -TT, and -SRBC primary and secondary IgM and IgG responses were compared. The influence of gender, country of origin, and previous immunization with a different antigen on response rate and kinetics of anti-KLH and anti-TT responses were analyzed. In addition, the magnitude of the antibody responses and the impact of the above-mentioned factors were analyzed. Also, based upon the inter-individual variability of the peak response values, power calculations were conducted. The analysis demonstrated that the rates of positive responses were similar between the two genders, were high for KLH, SRBC, and TT challenges by 21 days following immunization (87, 100, and 84%, respectively, for IgGs) and did not include statistically significant differences based on NHP country of origin. Mean peak secondary responses were greater than peak primary responses; the magnitude of the response to KLH was increased by incomplete Freund’s adjuvant (IFA). Gender had little effect on the magnitude and variability of these responses. KLH and TT were associated with similar inter-animal variability, while in some situations KLH responses were less variable than responses to SRBC. The data suggested that inter-animal variability with KLH was similar with or without IFA. Power analysis illustrated that animal group sizes of typical standard toxicology studies (generally ≤ 4/sex) are likely to detect only fairly large treatment effects. However, combining males and females, when appropriate, will improve the power: an n of 8–12 could detect ≤ 3.1-fold differences in anti-KLH IgG responses. The detail of this work was published in the Journal of Immunotoxicology (Lebrec et al., Citation2011). In addition, immunophenotyping of peripheral lymphocytes is also commonly used to identify immunotoxic effects of compounds in several species including NHPs. A retrospective inter-laboratory analysis of immunophenotyping in NHPs was conducted as well; while not the main focus of this presentation at the SOT session, results will be published in the near future.
Declaration of interest
This publication stems from a sub-group of the Health and Environmental Sciences Institute Immunotoxicology Technical Committee, whose work is funded through ILSI-HESI. The author reports no conflicts of interest. The author alone is responsible for this segment of the publication.
Monitoring T-lymphocyte responses against cytomegalovirus infection in rhesus macaques
Amitinder Kaur
Division of Immunology, New England Primate Research Center, Harvard Medical School, Southborough, MA, USA
Address for Correspondence: Dr Amitinder Kaur, Division of Immunology, New England Primate Research Center, Harvard Medical School, Southborough, MA 01772, USA. Tel: 508–624-8169; E-mail: [email protected]
Introduction
Non-human primates are closely related to humans and serve as the most biologically relevant animal model available for study of host-pathogen interactions in human. Human cytomegalovirus (CMV) is a ubiquitous pathogen belonging to the betaherpesvirus sub-family of herpesviruses that induces persistent, life-long infection in humans. CMV infection is also widely prevalent among other primates, and CMV isolates have been recovered from great Apes, New World monkeys, and Old World monkeys. CMV is highly species-specific and each non-human primate species harbors its own indigenous virus that appears to have co-evolved with its host.
Rhesus macaques are a leading non-human primate animal model of AIDS and AIDS-related opportunistic pathogens such as CMV in humans (Kaur et al., Citation2003). Rhesus CMV was first isolated in 1968 from the urine of healthy adult rhesus macaques (Asher et al., Citation1974). Since then two isolates of rhesus CMV have been completely sequenced (Hansen et al., Citation2003; Rivailler et al., Citation2006). Rhesus CMV is closely similar to human CMV with regards to natural history, disease syndromes, and virus sequence homology, and, thus, the rhesus macaque animal model has important advantages over murine and guinea pig models of CMV infection (Yue and Barry, Citation2008). This review will focus on the available tools and assays for measurement of cellular immunity in rhesus macaques with emphasis on detection of rhesus CMV-specific CD4+ and CD8+ T-lymphocyte responses and their relation to immune control of CMV in immunocompetent and immunocompromised rhesus macaques. CMV infection in rhesus macaques serves as a model chronic virus infection that can be utilized for assessing the potential effects of immunomodulatory drugs in humans.
Natural history of CMV infection in rhesus macaques
Seroepidemiologic studies have shown close to 100% prevalence rates of CMV infection in captive, group-housed rhesus macaques (Vogel et al., Citation1994; Andrade et al., Citation2003). Maternal antibodies to CMV wane around 1 year of age, and seroconversion following natural infection occurs soon thereafter (Vogel et al., Citation1994; Kaur et al., Citation1996). Hand-reared newborn macaques separated at birth from their mothers remain CMV-seronegative after loss of their maternal CMV antibodies, and can be used for experimental CMV infection studies (Kaur et al., Citation1996).
The course of CMV infection in rhesus macaques is similar to that of human CMV infection in several key respects. Immunocompetent CMV-seropositive macaques show intermittent virus shedding in the urine but are otherwise asymptomatic (Swack and Hsiung, Citation1982). Overt CMV disease only manifests in immunosuppressed NHPs, often in the setting of simian AIDS or organ transplantation (Baskin, Citation1987; Mueller et al., Citation2002; Pearson et al., Citation2002; Sequar et al., Citation2002; Kaur et al., Citation2003). CMV disease has been reported at necropsy in 30–50% of simian immunodeficiency virus (SIV)-infected rhesus macaques with AIDS (Baskin et al., Citation1988; Kaur et al., Citation2003). With the exception of CMV retinitis, the profile of organs affected by CMV disease in simian AIDS is similar to HIV-infected humans with CMV disease.
Detection of CMV-specific cellular immunity in rhesus macaques
The availability of rhesus CMV-specific reagents and optimization of flow cytometric assays for detection of cytokine-secreting and degranulating antigen-specific T-lymphocytes in rhesus macaques has enabled comprehensive evaluation of rhesus CMV-specific T-lymphocyte responses. Some of these considerations are detailed below.
Rhesus CMV antigens for in vitro stimulation
Antigens currently being used to stimulate peripheral blood mononuclear cells (PBMC) for detection of rhesus CMV-specific T-lymphocytes include whole virus rhesus CMV antigen, vaccinia recombinants, and 15 amino acid long overlapping peptides spanning the open reading frames of rhesus CMV immediate early 1 and 2 (IE1 and IE2), phosphoprotein 65 (pp65), and viral IL-10 proteins. Whole rhesus CMV antigen prepared from primary monkey fibroblast lines infected with a clinical isolate of rhesus CMV was shown to predominantly stimulate CMV-specific CD4+ T-lymphocytes, whereas rhesus CMV vaccinia recombinants pre-dominantly stimulate CMV-specific CD8+ T-lymphocytes (Kaur et al., Citation2002, Citation2003). The overlapping peptide pools detect both CD4+ and CD8+ T-lymphocyte responses.
Assays for quantitation of antigen-specific T-lymphocytes
The interferon (IFN)-γenzyme-linked immunospot (ELISPOT) assay and the flow cytometric intracellular cytokine staining (ICS) assay are now widely used for detection of antigen-specific cytokine-secreting T-lymphocytes at the single cell level and have largely supplanted traditional proliferative and 51Cr release cytotoxic T-lymphocytes (CTL) assays. Advantages over conventional assays of measuring cellular immunity include increased sensitivity, short duration of in vitro stimulation, and low likelihood of activation-induced apoptosis of antigen-specific T-lymphocytes. Because detection of positive responses precedes in vitro expansion, these assays provide sensitive quantitative estimates of ex vivo frequencies of antigen-specific T-lymphocytes. Flow cytometric-based assays additionally enable simultaneous evaluation of multiple effector functions at the single cell level and provide information on the precise phenotype of antigenic-specific T-lymphocytes of interest. We have successfully adapted the ICS and ELISPOT assays to detect CMV-specific CD4+ and CD8+ T-lymphocytes in rhesus macaques. Our results show that the ELISPOT and ICS assays are 3–1000-fold more sensitive than the traditional proliferative limiting dilution assay (LDA) for measurement of CMV-specific CD4+ T-lymphocytes (Kaur et al., Citation2002). The frequency of CMV-specific CD4+ T-lymphocytes detected by ICS was 1–3 logs greater than by proliferative LDA, while frequencies determined by IFNγ elispot were 3–100-fold greater than those obtained by proliferative LDA assays. The frequency of CMV-specific CD4+ T-lymphocytes determined by IFNγ ICS was 1.3–16.3-fold higher than that obtained by IFNγ ELISPOT (Kaur et al., Citation2002). As expected, we observed a significant positive correlation between the frequency of CMV-specific CD4+ T-lymphocytes determined by IFNγ ICS and ELISPOT assay (Kaur et al., Citation2002).
We are using a step-wise approach to measure CMV-specific CD4+ and CD8+ T-lymphocyte responses in rhesus macaques. Initial determination of frequency and specificity is performed with the ELISPOT assay followed by the ICS assay to establish the CD8 or CD4 specificity, and enable multifunctional analysis and phenotypic characterization of responder cells for individual CMV-specific responses. The relative advantages and disadvantages of the ELISPOT and ICS assay for measurement of CMV-specific T-lymphocytes in rhesus macaques are summarized in . Initial screening with an ELISPOT assay has several advantages. ELISPOT allows for measurement of responses against multiple antigens with a limited number of cells, assays are reproducible and much less subject to inter-operator variation, they generally provide better discrimination of a positive signal from background noise, and thus can be more sensitive for detection of low frequency responses (). In contrast, low frequency responses can be missed in an ICS assay because of a low signal-to-noise ratio, even though ICS is more sensitive than ELISPOT for precise quantitation of high frequency responses ().
Table 1. Comparison of ELISPOT and ICS assays for detection of CMV-specific T-lymphocyte responses in rhesus macaques.
Optimization of ICS assay in rhesus macaques
Studies to determine the optimal experimental conditions for detecting rhesus CMV-specific and SIV-specific T-lymphocyte responses by ICS assay were performed in rhesus macaques as previously described (Kaur et al., Citation2002; Gauduin et al., Citation2004). Parameters that were examined included conditions required for optimal co-stimulation, antigen dose, duration of stimulation, antibody combinations, comparison of freshly isolated vs viably cryopreserved PBMC, and use of monensin or brefeldin A for intracellular retention of secreted cytokines.
In contrast to human studies, soluble co-stimulatory antibodies of antihuman specificity had no effect on detection of antigen-specific T-lymphocyte responses in rhesus macaques. Co-stimulation only worked after antibody cross-linking. Thus, cross-linked co-stimulatory anti-CD28 mAb resulted in a roughly 2-fold enhancement of the frequency of tumor necrosis factor (TNF)-α-secreting CD4+ T-lymphocytes responding to super-antigen or whole CMV and SIV antigens (Kaur et al., Citation2002; Gauduin et al., Citation2004). The use of two co-stimulatory antibodies, anti-CD28 in combination with anti-CD49d or anti-CD5, resulted in an additional 3–4-fold enhancement of the specific cytokine signal without increasing the background. The enhancing effect of co-stimulatory antibodies was not as pronounced in peptide-stimulated cells. Other experimental conditions that were found essential for obtaining low noise and high specific cytokine signals included the use of freshly isolated PBMC that were either used the same day or cryopreserved immediately for later use, antigen-specific stimulation conducted in tubes slanted 5° above the horizontal, as described by Waldrop et al. (Citation1997), use of CD69 co-expression to identify recently activated cells, and optimal concentration of CMV antigen (Kaur et al., Citation2002).
Although both brefeldin A and monensin are agents that inhibit protein transport from the endoplasmic reticulum to the Golgi complex and are used in ICS assays to block cytokine egress and allow detection of accumulated intracellular cytokine in activated lymphocytes, they may have differential inhibitory effects on cytokine transport. In experiments to determine the optimal agent for use in rhesus macaques, we observed that monensin resulted in a significantly lower frequency of both IFNγ- and TNFα-secreting cells compared to brefeldin A (Chan and Kaur, Citation2007). The blunting effect of monensin on intracellular IFNγ and TNFα signal was more pronounced at the end of 18 h. Hence, brefeldin A is the agent of choice for blocking cytokine egress in ICS assays in rhesus macaques.
Flow cytometric detection of degranulating rhesus CMV-specific CD8+ T-lymphocytes
Granule-dependent lysis of target cells by CTL is mediated by release of lytic granules located within membrane-bound secretory lysosomes. Granule release or degranulation is associated with transient exposure of lysosomal associated membrane glycoproteins and this property has been used for flow cytometric identification of degranulating CD8+ T-lymphocytes in humans (Betts et al., Citation2003). Adaptation of this protocol successfully identified degranulating effector CMV-specific CD8+ T-lymphocytes in rhesus macaques (Chan and Kaur, Citation2007).
CMV-specific cellular immunity in immunocompetent rhesus macaques
Using optimal conditions for ICS assays as described above, we were able to detect recently activated (CD69+) CD4+ T-lymphocytes secreting IFNγ or TNFα in CMV-seropositive rhesus macaques after stimulation with whole virus rhesus CMV antigen (Kaur et al., Citation2002). The cytokine response of CD4+ T-lymphocytes was MHC-class II restricted. The ICS assay was highly sensitive and specific and had a low intra-assay and inter-assay variation. Using this technique, we determined the frequency of CMV-specific CD4+ T-lymphocytes in a cohort of CMV-seropositive rhesus macaques in the breeding colony at NEPRC (). In 23 CMV-seropositive SIV naive rhesus macaques with a median age of 12 years (range 2.7–22 years), the frequency of IFNγ-secreting CMV-specific CD4+ T-lymphocytes ranged between 0.16–5.8% () and varied <2-fold over a 6-month period (Kaur et al., Citation2002). Using peptide pools of rhesus CMV IE1, IE2, pp65, and IL-10 proteins, CMV-specific CD8+ T-lymphocytes targeting one or more of the four rhesus CMV proteins were detected in all the CMV-seropositive macaques (). In contrast to human CMV infection, the IE1 protein was the most frequent immunodominant target. In individual animals, the magnitude of CMV-specific CD8+ T-lymphocytes targeting the immediate early proteins accounted for >25% of the circulating CD8+ T-lymphocytes in some animals ().
Table 2. CMV-specific T-lymphocyte frequencies determined by IFNγ ICS assay in a cohort of CMV-seropositive rhesus macaques at NEPRC.
CMV-specific cellular immunity and immune control in rhesus macaques
CMV reactivation progressing to overt CMV disease is a frequent occurrence in SIV-infected rhesus macaques and is related to impaired CMV-specific cellular and humoral immunity (Kaur et al., Citation2002, Citation2003; Sequar et al., Citation2002). In a longitudinal study of SIV-infected CMV-seropositive rhesus macaques, we showed that declining CMV-specific CD4+ and CD8+ T-lymphocytes along with low anti-CMV neutralizing antibodies were predictive of occurrence of CMV disease in simian AIDS (Kaur et al., Citation2003). A direct relationship between loss of cellular immunity and CMV reactivation in non-human primates was also observed following in vivo administration of lymphocyte-depleting antibodies. SIV-negative pig-tailed macaques and African green monkeys showed evidence of a transient increase in CMV viremia following in vivo deletion of CD8+ T-lymphocytes (Schmitz et al., Citation2009). CD8+ T-lymphocyte depletion of SIV-infected pig-tailed macaques led to an irreversible increase in CMV viremia and progression to AIDS (Schmitz et al., Citation2009).
Overall, studies of CMV infection in rhesus macaques have highlighted similarities to human CMV infection and demonstrated the central role of adaptive immunity in immune control of CMV infection. The sensitive inter-play between CMV-specific immunity and CMV reactivation suggest that rhesus macaques will be a useful non-human primate model for testing the immunomodulatory properties of potential drug candidates.
Declaration of interest
The author reports no conflicts of interest. The author alone is responsible for this segment of the publication.
References
- Altman, J. D., Moss, P. A., Goulder, P. J., Barouch, D. H., Mcheyzer-Williams, M. G., Bell, J. I., McMichael, A. J., and Davis, M. M. 1996. Phenotypic analysis of antigen-specific T-lymphocytes. Science 274:94–96.
- Andrade, M. R., Yee, J., Barry, P., Spinner, A., Roberts, J. A., Cabello, P. H., Leite, J. P., and Lerche, N. W. 2003. Prevalence of antibodies to selected viruses in a long-term closed breeding colony of rhesus macaques (Macaca mulatta) in Brazil. Am. J Primatol. 59:123–128.
- Asher, D. M., Gibbs, C. J., Lang, D. J., Gajdusek, D. C., and Chanock, R. M. 1974. Persistent shedding of cytomegalovirus in the urine of healthy Rhesus monkeys. Proc. Soc. Exp. Biol. Med. 145:794–801.
- Barten, M. J., Dhein, S., Chang, H., Bittner, H. B., Tarnok, A., Rahmel, A., Mohr, F. W., and Gummert, J. F. 2003. Assessment of immunosuppressive drug interactions: Inhibition of lymphocyte function in peripheral human blood. J Immunol. Meth. 283:99–114.
- Baskin, G. B. 1987. Disseminated cytomegalovirus infection in immunodeficient rhesus monkeys. Am. J Pathol.129:345–352.
- Baskin, G. B., Murphey-Corb, M., Watson, E. A., and Martin, L. N. 1988. Necropsy findings in rhesus monkeys experimentally infected with cultured simian immunodeficiency virus (SIV)/delta. Vet. Pathol. 25:456–467.
- Bendall, S. C., Simonds, E. F., Qui P., Amir, E. D., Krutzik, P. O., Finck, R., Bruggner, R. V., Melamed, R., Trejo, A., Ornatsky, O., Balderas, R. S., Plevritis, S. K., Sachs, K., Pe’er, D., Tanner, S. D., and Nolan, G. P. 2011. Single-cell mass cytometry of differential immune and drug responses across a human hematopoetic continuum. Science 332:687–696.
- Berger, J. R., and Houff, S. 2009. Opportunistic infections and other risks with newer multiple sclerosis therapies. Ann. Neurol. 65:367–377.
- Betts, M. R., Brenchley, J. M., Price, D. A., De Rosa, S. C., Douek, D. C., Roederer, M., and Koup, R. A. 2003. Sensitive and viable identification of antigen-specific CD8+ T-cells by a flow cytometric assay for degranulation. J Immunol. Meth. 281:65–78.
- Bolton, D. L., and Roederer, M. 2009. Flow cytometry and the future of vaccine development. Expert Rev. Vaccines 8:779–789.
- Budde, M. L., Wiseman, R. W., Karl, J. A., Hanczaruk, B., Simen, B. B., and O’ Connor, D. H. 2010. Characterization of Mauritian cynomolgus macaque major histocompatibility complex Class I haplotypes by high-resolution pyrosequencing. Immunogenetics 62:773–780.
- Bugelski, P. J., Volk, A., Walker, M. R., Krayer, J. H., Martin, P., and Descotes, J. 2010. Critical review of preclinical approaches to evaluate the potential of immunosuppressive drugs to influence human neoplasia. Int. J Toxicol. 29:435–466.
- Burwitz, B. J., Pendley, C. J., Greene, J. M., Detmer, A. M., Lhost, J. J., Karl, J. A., Piaskowski, S. M., Rudersdorf, R. A., Wallace, L. T., Bimber, B. N., Loffredo, J. T., Cox, D. G., Bardet, W., Hildebrand, W., Wiseman, R. W., O’ Connor, S. L., and O’ Connor, D. H. 2009. Mauritian cynomolgus macaques share two exceptionally common major histocompatibility complex Class I alleles that restrict simian immunodeficiency virus-specific CD8+ T-cells. J Virol. 83:6011–6019.
- Caldwell, R., Guirguis, M., and Kornbrust, E. 2007. Evaluation of various keyhole limpet hemocyanin dosing regimens for the cynomolgus monkey TDAR assay. The Toxicologist Abstract #1723.
- Carfi, M., Gennari, A., Malerba, I., Corsini, E., Pallardy, M., Pieters, R., van Loveren, H., Vohr, H. W., Hartung, T, and Gribaldo, L. 2007. In vitro tests to evaluate immunotoxicity: A preliminary study. Toxicology 229:11–22.
- Carville, A., and Mansfield, K. G. 2008. Comparative pathobiology of macaque lympho-cryptoviruses. Comp. Med. 58:57–67.
- Chan, K. S., and Kaur, A. 2007. Flow cytometric detection of degranulation reveals phenotypic heterogeneity of degranulating CMV-specific CD8+ T-lymphocytes in rhesus macaques. J Immunol. Meth. 325:20–34.
- Chattopadhyay, P. K., Yu, J., and Roederer, M. 2005. A live-cell assay to detect antigen-specific CD4+ T-cells with diverse cytokine profiles. Nat. Med. 11:1113–1117.
- Cohen, G. B., Kaur, A., and Johnson, R. P. 2005. Isolation of viable antigen-specific CD4 T-cells by CD40L surface trapping. J Immunol. Meth. 302:103–115.
- Cordoba, F., Wieczorek, G., Preussing, E., and Bigaud, M. 2008. Modeling of delayed type hypersensitivity (DTH) in the non-human primate (NHP). Drug Discov Today: Dis Models 5:63–71.
- CPMP (Committee for Proprietary Medicinal products). 2000. Note for guidance on repeated dose toxicity. CPMP/SWP/1042/99.
- Dumont, F. J., Melino, M. R., Staruch, M. J., Koprak, S. L., Fischer, P. A., and Sigal, N. H. 1990. The immunosuppressive macrolides FK-506 and rapamycin act as reciprocal antagonists in murine T-cells. J Immunol. 144:1418–1424.
- FDA (Food and Drug Administration, Center for Drug Evaluation and Research). 2002. Guidance for Industry. Immunotoxicology evaluation of investigational new drugs. FDA.
- Florese, R. H., Wiseman, R. W., Venzon, D., Karl, J. A., Demberg, T., Larsen, K., Flanary, L., Kalyanaraman, V. S., Pal, R., Titi, F., Patterson, L. J., Heath, M. J., O’ Connor, D. H., Cafaro, A., Ensoli, B., and Robert-Guroff, M. 2008. Comparative study of Tat vaccine regimens in Mauritian cynomolgus and Indian rhesus macaques: influence of Mauritian MHC haplotypes on susceptibility/resistance to SHIV(89.6P) infection. Vaccine 26:3312–3321.
- Fogg, M. H., Kaur, A., Cho, Y. G., and Wang, F. 2005. The CD8+ T-cell response to an Epstein-Barr virus-related gammaherpesvirus infecting rhesus macaques provides evidence for immune evasion by the EBNA-1 homologue. J Virol. 79:12681–12691.
- Frentsch, M., Arbach, O., Kirchoff, D., Moewes, B., Worm, M., Rothee, M., Scheffold, A., and Thiel, A. 2005. Direct access to CD4+ T-cells specific for defined antigens according to CD154 expression. Nat. Med. 11:1118–1124.
- Furst, D. E. 2010. The risk of infections with biologic therapies for rheumatoid arthritis. Semin. Arthritis Rheum. 39:327–346.
- Gauduin, M. C., Kaur, A., Ahmad, S., Yilma, T., Lifson, J. D., and Johnson, R. P. 2004. Optimization of intracellular cytokine staining for the quantitation of antigen-specific CD4+ T-cell responses in rhesus macaques. J Immunol. Meth..288:61–79.
- Hadrup, S. R., and Schumacher, T. N. 2010. MHC-based detection of antigen-specific CD8+ T-cell responses. Cancer Immunol. Immunother. 59:1425–1433.
- Haggerty, H. 2007. Immunotoxicity testing in non-rodent species. J Immunotoxicol. 4:165–169.
- Hansen, S. G., Strelow, L. I., Franchi, D. C., Anders, D. G., and Wong, S. W. 2003. Complete sequence and genomic analysis of rhesus cytomegalovirus. J Virol. 77:6620–6636.
- Hislop A. D., Taylor G. S., Sauce D., and Rickinson A. B. 2007. Cellular responses to viral infection in humans: Lessons from Epstein-Barr virus. Annu. Rev. Immunol. 25:587–617.
- ICH (International Conference on Harmonization). 2006. Guidance for Industry. S8 Immunotoxicity Studies for Human Pharmaceuticals.
- Imamura, T., Iyama, K., Takeya, M., Kambara, T., and Nakamura, S. 1993. Role of macrophage tissue factor in the development of the delayed hypersensitivity reaction in monkey skin. Cell. Immunol. 152:614–622.
- Johnson, D. E., Smith, D. A., and Park, B. K. 2010. Immunomodulatory therapies and the risk of opportunistic infections. Curr. Opin. Drug Discov. Devel. 13:20–22.
- Kaur, A., Daniel, M. D., Hempel, D., Lee-Parritz, D., Hirsch, M. S., and Johnson, R. P. 1996. Cytotoxic T-lymphocyte responses to cytomegalovirus in normal and simian immuno-deficiency virus-infected rhesus macaques. J Virol. 70:7725–7733.
- Kaur, A., Hale, C. L., Noren, B., Kassis, N., Simon, M. A., and Johnson R. P. 2002. Decreased frequency of cytomegalovirus (CMV)-specific CD4+ T-lymphocytes in simian immuno-deficiency virus-infected rhesus macaques: inverse relationship with CMV viremia. J Virol. 76:3646–3658.
- Kaur, A., Kassis, N., Hale, C. L., Simon, M., Elliott, M., Gomez-Yafal, A., Lifson, J. D., Desrosiers, R. C., Wang, F., Barry, P., Mach, M., and Johnson, R. P. 2003. Direct relationship between suppression of virus-specific immunity and emergence of cytomegalovirus disease in simian AIDS. J Virol. 77:5749–5758.
- Kirk, S. A., Fraser, S. R., Gordon, D., and Templeton, A. 2008. Evaluation of the T-cell dependent antibody response (TDAR) to tetanus toxoid (TT), in the cynomolgus monkey, in the presence of a known immunosuppressant. The Toxicologist. Abstract #2134.
- Kotton, C. N., and Fishman, J. A. 2005. Viral infection in the renal transplant recipient. J Am. Soc. Nephrol. 16:1758–1774.
- Krathen, M. S., Gottlieb, A. B., and Mease, P. J. 2010. Pharmacologic immunomodulation and cutaneous malignancy in rheumatoid arthritis, psoriasis, and psoriatic arthritis. J Rheumatol. 37:2205–2215.
- Krutzik, P. O., and Nolan, G. P. 2006. Fluorescent cell barcoding in flow cytometry allows high-throughput drug screening and signaling profiling. Nat. Meth. 3:361–368.
- Krutzik, P. O., Clutter, M. R., Trejo, A., and Nolan, G. P. 2011. Fluorescent cell barcoding for multiplex flow cytometry. Curr. Protocol Cytom. Chapter 6, Unit 6 31.
- Kumar, A., Weiss, W., Tine, J. A., Hoffman, S. L., and Rogers, W. O. 2001. ELISPOT assay for detection of peptide specific IFNγ-secreting cells in rhesus macaques. J Immunol. Meth. 247:49–60.
- Lebrec, H., Roger, R., Blot, C., Burleson, G. R., Bohuoun, C., and Pallardy, M. 1995. Immunotoxicological investigation using pharmaceutical drugs. In vitro evaluation of immune effects using rodent or human immune cells. Toxicology 96:147–156.
- Lebrec, H., Cowan, L., Lagrou, M., Krejsa, C., Neradilek, M., Polissar, N., Black, L., and Bussiere, J. 2011. An inter-laboratory retrospective analysis of immunotoxicological endpoints in non-human primates: T-Cell-dependent antibody responses. J Immunotoxicol. 8:238–250.
- Luster, M. I., Portier, C., Pait, D. G., Rosenthal, G., Germolec, D. R., Corsini, E., Blaylock, B. L., Pollock, P., Kouchi, Y., Craig, W., White, K. L. Munson, A. E., and Comment, C. E. 1993. Risk assessment in immunotoxicology. II. Relationships between immune and host resistance tests. Fundam. Appl. Toxicol. 21:71–82.
- Luster, M. I., Portier, C., Pait, D. G., White, K. L. Gennings, C., Munson, A. E., and Rosenthal, G. J. 1992. Risk assessment in immunotoxicology. I. Sensitivity and predictability of immune tests. Fundam. Appl. Toxicol. 18:200–210.
- McCutcheon, M., Wehner, N., Wensky, A., Kushner, M., Doan, S., Hsiao, L., Calabresi, P., Ha, T., Tran, T. V., Tate, K. M., Winkelhake, J., and Spack, E. G. 1997. A sensitive ELISPOT assay to detect low-frequency human T-lymphocytes. J Immunol. Meth. 210:149–166.
- Moghaddam, A., Rosenzweig, M., Lee-Parritz, D., Annis, B., Johnson, R. P., and Wang, F. 1997. An animal model for acute and persistent Epstein-Barr virus infection. Science 276:2030–2033.
- Mueller, N. J., Barth, R. N., Yamamoto, S., Kitamura, H., Patience, C., Yamada, K., Cooper, D. K., Sachs, D. H., Kaur, A., and Fishman, J. A. 2002. Activation of cytomegalovirus in pig-to-primate organ xenotransplantation. J Virol. 76:4734–4740.
- O’ Connor, S. L., Blasky, A. J., Pendley, C. J., Becker, E. A., Wiseman, R. W., Karl, J. A., Hughes, A. L., and O’ Connor, D. H. 2007. Comprehensive characterization of MHC Class II haplotypes in Mauritian cynomolgus macaques. Immunogenetics 59:449–462.
- Oelke, M., and Schneck, J. P. 2010. Overview of a HLA-Ig based “Lego-like system” for T-cell monitoring, modulation and expansion. Immunol. Res. 47:248–256.
- Oelke, M., Maus, M. V., Didiano, D., June, C. H., Mackensen, A., and Schneck, J. P. 2003. Ex vivo induction and expansion of antigen-specific cytotoxic T-cells by HLA-Ig-coated artificial antigen-presenting cells. Nat. Med. 9:619–624.
- Ornatsky, O., Bandura, D., Baranov, V., Nitz, M., Winnik, M. A., and Tanner, S. 2010. Highly multiparametric analysis by mass cytometry. J. Immunol. Meth. 361:1–20.
- Pearson, T. C., Trambley, J., Odom, K., Anderson, D. C., Cowan, S., Bray, R., Lin, A., Hollenbaugh, D., Aruffo, A., Siadak, A. W., Strobert, E., Hennigar, R., and Larse, C. P. 2002. Anti-CD40 therapy extends renal allograft survival in rhesus macaques. Transplantation 74:933–940.
- Piccotti, J., Alvey, J., Reindel, J., and Guzman, R. 2005. T-Cell-dependent antibody response: Assay development in cynomolgus monkeys. J. Immunotoxicol. 2:191–196.
- Picotti, J. R. 2008. T-cell dependent antibody response tests. In: Immunotoxicology Strategies for Pharmaceutical Safety Assessment, Danuta J.H., and Bussiere J., Eds., New York: John Wiley and Sons, Inc., pp. 67–76.
- Pitcher, C. J., Hagen, S. I., Walker, J. M., Lum, R., Mitchell, B. L., Maino, V. C., Axthelm, M. K., and Picker, L. J. 2002. Development and homeostasis of T-cell memory in rhesus macaque. J Immunol. 168:29–43.
- Radu, C. G., Shu, C. J., Nair-Gill, E., Shelly, S. M., Barrio, J. R., Satyamurthy, N., Phelops, M. E., and Witte, O. N. 2008. Molecular imaging of lymphoid organs and immune activation by positron emission tomography with a new [18F]-labeled 2′-deoxycytidine analog. Nat. Med. 14:783–788.
- Ribas, A., Benz, M. R., Allen-Auerbach, M. S., Radu, C., Chmielowski, B., Seja, E., Williams, J. L., Gomez-Navarro, J., McCarthy, T., and Czernin, J. 2010. Imaging of CTLA4 blockade-induced cell replication with [18F]-FLT PET in patients with advanced melanoma treated with tremelimumab. J Nucl. Med. 51:340–346.
- Rivailler, P., Carville, A., Kaur, A., Rao, P., Quink, C., Kutok, J. L., Westmoreland, S., Klumpp, S., Simon, M., Aster, J. C., and Wang, F. 2004. Experimental rhesus lymphocryptovirus infection in immunosuppressed macaques: An animal model for Epstein-Barr virus pathogenesis in the immunosuppressed host. Blood 104:1482–1489.
- Rivailler, P., Kaur, A., Johnson, R. P., and Wang, F. 2006. Genomic sequence of rhesus cytomegalovirus 180.92: Insights into the coding potential of rhesus cytomegalovirus. J VIrol. 80:4179–4182.
- Schmittel, A., Keilholz, U., Thiel, E., and Scheibenbogen, C. 2000. Quantification of tumor-specific T-lymphocytes with the ELISPOT assay. J Immunother. 23:289–295.
- Schmitz, J. E., Simon, M. A., Kuroda, M. J., Lifton, M. A., Ollert, M. W., Vogel, C. W., Racz, P., Tenner-Racz, K., Scallon, B. J., Dalesandro, M., Ghrayeb, J., Rieber, E. P., Sasseville, V. G., and Reimann, K. A. 1999. A non-human primate model for the selective elimination of CD8+ lymphocytes using a mouse-human chimeric monoclonal antibody. Am. J Pathol. 154:1923–1932.
- Schmitz, J. E., Zahn, R. C., Brown, C. R., Rett, M. D., Li, M., Tang, H., Pryputniewicz, S., Byrum, R. A., Kaur, A., Montefiori, D. C., Allan, J. S., Goldstein, S., and Hirsch, V. M. 2009. Inhibition of adaptive immune responses leads to a fatal clinical outcome in SIV-infected pigtailed macaques but not vervet African green monkeys. PLoS Pathog. 5:e1000691.
- Seder, R. A., Darrah, P. A., and Roederer, M. 2008. T-Cell quality in memory and protection: Implications for vaccine design. Nat. Rev. Immunol. 8:247–258.
- Sequar, G., Britt, W. J., Lakeman, F. D., Lockridge, K. M., Tarara, R. P., Canfield, D. R., Zhou, S. S., Gardner, M. B., and Barry, P. A. 2002. Experimental coinfection of rhesus macaques with rhesus cytomegalovirus and simian immunodeficiency virus: Pathogenesis. J Virol. 76:7661–7671.
- Singh, A. S., Radu, C. G., and Ribas, A. 2010. PET imaging of the immune system: Immune monitoring at the whole body level. Q. J Nucl. Med. Mol. Imaging. 54:281–290.
- Suntharalingam, G., Perry, M. R., Ward, S., Brett, S. J., Castello-Cortes, A., Brunner, M. D., and Panoskaltsis, N. 2006. Cytokine storm in a Phase 1 trial of the anti-CD28 monoclonal antibody TGN1412. New Engl. J Med. 355:1018–1028.
- Swack, N. S., and Hsiung, G. D. 1982. Natural and experimental simian cytomegalovirus infections at a primate center. J Med. Primatol. 11:169–177.
- Tichenor, J. N., Dumont, C., Coletti, K. S., LeSauteur, L., Calise, D. V., Christn-Piche, M. S., and Satterwhite, C. 2010. A 6-week study to determine the antibody response to FK-506-immunosuppressed cynomolgus monkeys following the subcutaneous administration of keyhole limpet hemocyanin and tetanus toxoid in the presence or absence of incomplete Freud’s adjuvant. The Toxicologist. Abstract # 1989.
- Vogel, P., Weigler, B. J., Kerr, H., Hendrickx, A. G., and Barry, P. A. 1994. Seroepidemiologic studies of cytomegalovirus infection in a breeding population of rhesus macaques. Lab. Anim. Sci. 44:25–30.
- Vollers, S. S., and Stern, L. J. 2008. Class II major histocompatibility complex tetramer staining: Progress, problems, and prospects. Immunology 123:305–313.
- Waldrop, S. L., Pitcher, C. J., Peterson, D. M., Maino, V. C., and Picker, L. J. 1997. Determination of antigen-specific memory/effector CD4+ T-cell frequencies by flow cytometry: Evidence for a novel, antigen-specific homeostatic mechanism in HIV-associated immunodeficiency. J Clin. Invest. 99:1739–1750.
- Wiseman, R. W., Wojcechowskyj, J. A., Greene, J. M., Blasky, A. J., Gopon, T., Soma, T., Friedrich, T. C., O’ Connor, S. L., and O’ Connor, D. H. 2007. Simian immunodeficiency virus SIVmac239 infection of major histocompatibility complex-identical cynomolgus macaques from Mauritius. J Virol. 81:349–361.
- Wolfl, M., Kuball, J., Eyrich, M., Schlegel, P. G., and Greenberg, P. D. 2008. Use of CD137 to study the full repertoire of CD8+ T-cells without the need to know epitope specificities. Cytometry A 73:1043–1049.
- Yue, Y., and Barry, P. A. 2008. Rhesus cytomegalovirus: A non-human primate model for the study of human cytomegalovirus. Adv. Virus Res. 72:207–226.
- Yuzawa, K., Konishi, A., Takehana, K., Iino, Y., Ohnuki, A., Kobayashi, T., Fukao, K., and Ohkohchi, N. 2003. APC0576: A novel small molecule, immunosuppressive agent effective in primate models. Transplantation 75:1463–1468.
- Zaritskaya, L., Shurin, M. R., Sayers, T. J., and Malyguine, A. M. 2010. New flow cytometric assays for monitoring cell-mediated cytotoxicity. Expert Rev. Vaccines. 9:601–616.
- Zuber, B., Quigley, M. F., Critchfield, J. W., Shacklett, B. L., Abel, K., Miller, C. J., Morner, A., Paulie, S., Ahlborg, N., and Sandberg, J. K. 2006. Detection of macaque perforin expression and release by flow cytometry, immunohistochemistry, ELISA, and ELISpot. J Immunol. Meth. 312:45–53.